Ecotoxicological impact of graphene oxide: toxic effects on the model organism Artemia franciscana†
Received
15th July 2020
, Accepted 28th September 2020
First published on 21st October 2020
Abstract
Given the numerous potential applications of graphene oxide (GO) and its consequent release into the environment, this study was carried out to assess the toxic effects of GO on Artemia franciscana, a well-established model organism for marine ecotoxicological studies. A. franciscana stage I nauplii or adults were exposed to GO (1–100 μg mL−1) up to 72 h, which induced a significant mortality only in adults exposed to the highest concentration for 72 h. The susceptibility of adults to GO was further investigated evaluating other biomarkers of toxicity: already 24 h exposure to 100 μg mL−1 GO induced significant activation of the xenobiotic detoxifying and antioxidant enzyme glutathione S-transferase, whereas other toxicological parameters, such as reactive oxygen species (ROS) production, cholinesterase activity and growth rate, were not affected even after 72 h exposure. Furthermore, the uptake of GO was studied in relation to food supplement: GO accumulation in the digestive tract was lower in the presence of food, with respect to non-fed organisms. In conclusion, this study highlights the weak toxic effects of GO on A. franciscana adults, lower than those induced by other carbon-based materials. However, this suggests a possible ecotoxicological impact of GO that needs to be further studied.
Environmental significance
The wide variety of technological applications of graphene-based materials (GBMs) and their consequent entrance into the market pose serious concerns on their environmental safety. However, their potential environmental impact, in particular on the aquatic ecosystem, is still scarcely investigated. To investigate the ecotoxicological effect of graphene oxide (GO), as representative of GBMs, this study was carried out on the model organism Artemia franciscana for ecotoxicological studies. GO showed weak toxicity on A. franciscana adults, but not on larvae, leading to organism death, not associated with oxidative stress, only after long exposure to high concentrations. These results cannot exclude that GO could compromise the Artemia population, with possible effects on ecosystem biodiversity, Artemia spp. being part of the aquatic food chain.
|
1. Introduction
Graphene-based materials (GBMs) are a class of carbon-based materials characterized by their extraordinary mechanical, optical, electrical and thermal features. Their physicochemical properties qualify them as attractive nanotools, finding potential applications in biomedicine and nanoelectronics as new components for photothermal/photodynamic therapy, tissue engineering, and gene/drug delivery,1 as well as chemical solar and fuel cells, supercapacitors, ultrasensitive sensors, and rapid charging/discharging batteries, just to name a few.2 Among GBMs, graphene oxide (GO) has been widely explored in several fields, in particular for clinical therapeutic strategies,1 thanks to the easy functionalization and dispersibility in aqueous solutions. The wide variety of possible applications of GO, the development of commercial products and the related waste generation pose the problem of the material introduction into the environment. Thus, it is important to study the ecotoxicological impact of GO. In particular, GO physicochemical properties, such as large surface area and the presence of reactive oxygen functional groups (e.g., epoxide, hydroxyl, ester, carboxyl and carbonyl groups), endow the material with superior hydrophilicity compared to other GBMs,3–7 favouring its dispersion in aquatic environments as well as interactions with aquatic organisms. However, the potential impact of GO on the aquatic ecosystem, in particular the marine one, has been scarcely elucidated so far. Consequently, the toxicity of GO on aquatic organisms and the risk associated with their exposure to the material in aquatic sources deserve to be fully investigated.
It has been already reported that this material could induce a negative impact on marine organisms. For instance, it has been shown that GO was able to reduce the growth and the photosynthetic pigment concentration in algae of the genus Picochlorum.8 In the polychaete Diopatra neapolitana, GO induced cellular damage, has negative effects on the regenerative capacity and altered energy-related responses.9 In addition, in vitro assays on mussel (Mytilus galloprovincialis) hemocytes showed that GO induced significant cytotoxic effects, with increased reactive oxygen species (ROS) production and membrane damage.10 Moreover, studies carried out on crustaceans, such as Amphibalanus amphitrite larvae11 and Artemia salina nauplii,12–14 demonstrated the ability of GO to increase mortality and ROS production.
Artemia, a genus of anostracan crustaceans adapted to the hypersaline environment and present in salt lakes, coastal lagoons and man-made salt pans,15 includes species commonly used in toxicological and ecotoxicological studies, in particular those related to the assessment of the adverse impacts at the marine level.16 The different characteristics of Artemia spp. make them model organisms in this research field. For instance, Artemia species are suitable for laboratory culture and maintenance, they have a short-life cycle and a wide geographic distribution and there is a good knowledge about their biology and ecology.15 These species are characterized by more than 15 molts during their life cycle, with four mainly distinguishable developmental stages (nauplius, metanauplius, juvenile and adult), and they feed on bacteria, microalgae and protozoa.17 The main predators of Artemia are birds and corixids18 but this crustacean is a suitable food source for different organisms, such as foraminifers, coelenterates, flatworms, polychaetes, cnidarians, squids, insects, chaetognaths, fish, and other crustaceans.19Artemia franciscana is the most abundant species of Artemia, largely used also for aquaculture purposes.
In the last decade, Artemia spp. have started to gain attention as a biological model suitable for nanoecotoxicity testing20 and specific International Organization for Standardization (ISO) guidelines have been recently introduced to assess the aquatic acute toxicity of nanomaterials by a standardized testing procedure using Artemia spp. nauplii.21 Since 2009, more than 50 studies have been published on Artemia spp. used as an organism model for the assessment of the ecotoxicological impact of nanomaterials at the aquatic level, including silver, titanium dioxide, and various carbon-based materials, such as fullerene, carbon black, carbon nanodots, and graphene.21 For example, Pretti et al. found no mortality in A. salina nauplii exposed to pristine graphene monolayer flakes (up to 10 μg mL−1) for 24 h; however, after 48 h of exposure, lower concentrations induced oxidative stress, detected by an increased activity of catalase and glutathione peroxidase.22 In addition, reduced graphene oxide quantum dots induced dose- and time-dependent mortality of A. salina nauplii, with a maximum effect (about 50% mortality) after 48 h exposure to the highest concentration (160 μg mL−1).23
Considering the possible future GO release into the aquatic environment during its industrial life-cycle, herein we investigated the effects of GO on A. franciscana. The GO effect was evaluated on both nauplii and adults, determining the mortality after 24, 48 and 72 h exposure, as well as other biomarkers of toxicity, including ROS production, growth rate, and activity of selected enzymes (glutathione S-transferase, as a xenobiotic detoxifying and antioxidant enzyme, and cholinesterase, as a marker of neurotoxicity). In addition, the uptake and accumulation of GO in A. franciscana were investigated.
2. Methods
2.1 Chemicals
GO (batch #GOB067) was supplied by Graphenea (San Sebastian, Spain). The complete characterization of the material was previously reported:24 briefly, the elemental analysis showed average values of 59.40 ± 0.10% C, 1.40 ± 0.10% H, 0.07 ± 0.02% N and <36.6% O. The average lateral dimension, evaluated by laser diffraction in the GO slurry, was 15
100 ± 400 nm with a lateral size distribution located between 6000 and 30
000 nm. X-ray diffraction (XRD) analysis performed on a GO dry film revealed six layers. Representative Raman spectra, high resolution electron transmission microscopy (HR-TEM) and scanning electron microscopy (SEM) images are reported in the ESI† (Fig. S1). For the complete GO physicochemical characterization, refer to our previous study performed with the same batch of GO.24 The synthesis of GO was done in acidic media (H2SO4) and the final dispersion of the material was in water. The purification was carried out by repeated washings in distilled water, but elemental analysis revealed that a small percentage of S (around 2%) remained in the final material, mostly as SO42−.
2.2 Model organism
Dehydrated cysts of A. franciscana and products for the hatching and breeding of this crustacean were purchased from Hobby (Gelsdorf, Germany). To obtain instar I stage larvae, approximately 300 mg cysts were hatched in a specific Artemia hatchery dish in 750 mL artificial seawater (for each litre of deionized H2O, 36 g of Optimum Sea basic salt; Wave) in the constant presence of artificial light at 25 °C for 24 h. Optimum Sea basic salts are specific for marine organisms and, as declared by the supplier, they are composed of over 70 elements with the following main characteristics: Ca2+ = 440 mg L−1, Mg2+ = 1300 mg L−1, and absence of nitrates and phosphates. Subsequently, the larvae were separated from unhatched cysts, transferred to fresh artificial seawater and (i) used for the mortality test or (ii) transferred into a beaker and bred in artificial seawater for 21 days to obtain A. franciscana adults. During the first 10 days from hatching, Artemia were fed three times a week with liquid (Liquizell, Hobby; Germany), and subsequently, solid food (Mikrozell, Hobby; Germany), specific for Artemia culturing. The organisms were maintained at 25 °C under a 16
:
8 h light/dark cycle.
2.3 Genetic identification of Artemia franciscana
The identification of the species Artemia franciscana was made using gDNA extracted from 5 organisms with the GenElute™ Blood Genomic DNA kit (Sigma-Aldrich, Milan, Italy). A fragment of the cytochrome c oxidase subunit I (COI) gene was amplified using LCO1490: 5′-ggtcaacaaatcataaagatattgg-3′ and HC02198: 5′-taaacttcagggtgaccaaaaaatca-3′ primers.25 PCR, carried out in a final volume of 50 μL, was performed with 1× PerfeCTa SYBR Green SuperMix (Quantabio Beverly, MA, USA), 0.3 μM of each primer, and 18 ng of gDNA, following the touchdown thermal profile as follows: initial denaturation 95 °C for 60 s; 35 cycles with denaturation at 95 °C for 10 s, annealing at 56 °C for 30 s and elongation at 72 °C for 20 s plus a final extension at 72 °C for 60 s. The PCR was checked using 1.5% TAE electrophoretic gel and the expected band of 710 bp was excised and purified with the EZNA® Gel extraction kit (Omega Bio-tek; Norcross, USA). The purified PCRs were sent to an external service to be Sanger sequenced (Eurofins; Hamburg, Germany).
2.4 Mortality test
The mortality test was performed in triplicate on nauplii collected after 24 h from hatching that correspond approximately to first instar (stage I), and on adult organisms (21 days). For each treatment, 5 instar nauplii were transferred into each well of a 96-well polystyrene plate containing 200 μL of GO suspended in seawater (1, 10 and 100 μg mL−1) and incubated for 24, 48 and 72 h at 25 °C under a 16
:
8 h light/dark cycle, whereas for adults, 50 organisms were transferred into each well of a 6-well plate containing 4.5 mL GO suspension under the same conditions. Nauplii were not fed during the exposure to GO, while Artemia adults received solid feed 24 h before and 24 h after exposure to GO. After exposure to GO, plates were examined under a binocular stereomicroscope (Kyowa, Tokyo, Japan) at 3× magnification for the nauplii and 1× magnification for the adults. The number of dead shrimps was evaluated defining mortality in the absence of any movement during 10 seconds of observation according to Zulkifli et al.26 Data are reported as % of dead organisms with respect to the total number of organisms exposed to the same concentration of GO.
2.5 ROS detection
ROS production in A. franciscana adults was evaluated after 24 and 72 h exposure to GO (1, 10 and 100 μg mL−1) using the 2′,7′-dichlorofluorescin diacetate (DCFDA) assay (Sigma-Aldrich; Milan, Italy) able to fluorimetrically measure hydroxyl/peroxynitrite radicals according to Zhu et al.13 Briefly, for each determination, 5 organisms were washed 3 times in TRIS-HCl (100 mM, pH 7.5) and homogenized in 1 mL of the same buffer for 15 seconds using an immersion sonicator (ultrasonic processor UP50H; Hielscher, Teltow, Germany). The samples were centrifuged at 12
000g for 15 min at 4 °C, and the supernatant was collected and stored at −80 °C. To measure ROS production, in each well of a 96-well plate, 20 μL of supernatant were added and mixed with 160 μL of phosphate buffer saline (PBS) and 20 μL of DCFDA (final concentration 40 μM). The plate was incubated 30 min at 37 °C in the dark and the fluorescence was read using a microplate reader (Fluorocount, Packard; Germany) at 485 nm and 520 nm (the wavelength of excitation and emission, respectively). The protein content was measured using a NanoDrop 2000 (Thermo Scientific, Milan, Italy) and, for each sample, the final results were reported as relative fluorescence units (RFU) normalized on mg proteins.
2.6 Enzyme extraction
Enzymes (glutathione S-transferase and cholinesterase) were extracted from Artemia adults according to Jemec et al.27 Briefly, 50 adults were exposed to GO (1, 10 or 100 μg mL−1) for 24 and 72 h. Subsequently, living organisms were collected, washed three times with phosphate buffer (50 mM, pH 7 composed of Na2HPO4 and NaH2PO4) containing EDTA (5 mM), sonicated in 240 μL of phosphate buffer (50 mM, pH 7) and centrifuged at 15
000g for 25 min at 4 °C. The supernatants were collected and preserved at −80 °C after protein quantitation using the NanoDrop.
2.6.1 Glutathione S-transferase activity.
To evaluate the activity of glutathione S-transferase (GST), 25 μL of supernatant were added into each well of a 96-well plate, followed by 25 μL of 4 mM reduced L-glutathione (Sigma-Aldrich; Milan, Italy), 2 μL of 50 mM 1-chloro-2,4-dinitrobenzene (CDNB; Sigma-Aldrich; Milan, Italy) and 48 μL of potassium phosphate buffer (100 mM, pH 6.5, composed by K2HPO4 and KH2PO4). The blank was prepared in the same way, but the probe (CDNB) was replaced with 2 μL potassium phosphate buffer. The absorbance was read using a microplate reader (PowerWaveX, Bio-Tek Instruments Inc.; Vermont, USA) at 340 nm every 30 s for 4 min.
2.6.2 Cholinesterase activity.
To evaluate the activity of cholinesterase (ChE), in each well of a 96-well plate, 50 μL of supernatant were mixed with 10 μL of 10 mM acetylthiocholine iodide (Sigma-Aldrich; Milan, Italy), 10 μL of 5 mM 5,5′-dithiobis(2-nitrobenzoic acid) (DTNB) (Sigma-Aldrich; Milan, Italy) and 30 μL of potassium phosphate buffer (100 mM, ph 7.4). In the case of blank, DTNB was replaced with potassium phosphate buffer. The absorbance was measured at 405 nm every minute for 3 min with a microplate reader (TECAN; Männedorf, Switzerland).
2.6.3 Quantitation of the enzymatic activity.
The enzymatic activity was expressed as enzymatic units (EU), calculated as follows:
where ε was 9600 M−1 cm−1 and 13
600 M−1 cm−1 for GST and ChE, respectively.
The enzymatic activity was reported as the EU per number of organisms in the sample.
2.7 Protein content
The protein content was analysed to investigate the GO effect on A. franciscana growth. Briefly, after exposure to GO, organisms were collected and processed as reported for enzyme extraction. The protein content in the supernatants was measured using a NanoDrop instrument (NanoDrop 2000; Thermo Scientific; Milan, Italy) at a wavelength of 280 nm. The results were reported as mg proteins normalized on the number of organisms in each sample.
2.8 Uptake of GO
To evaluate the uptake of GO by A. franciscana, 10 organisms, fed 24 h before treatment, were exposed to GO (1, 10 and 100 μg mL−1) in the presence or absence of food (added 24 h after exposure to GO). After 24, 48 and 72 h exposure, the organisms were fixed with 4% p-formaldehyde (Sigma-Aldrich; Milan, Italy) for 20 min at room temperature. After three washes with distilled water, the organisms were observed under a binocular stereomicroscope (Kyowa; Tokyo, Japan) and the presence of GO and/or feed in the lumen of the intestinal tract was optically evaluated (black and green colour, respectively).
2.9 Statistical analysis
Statistical analyses were provided using GraphPad Prism software version 6.00. Means and standard errors of the means (SE) of independent experiments were calculated and analysed by the Student's t-test. For each analysis, statistical significance was considered for p values <0.05.
3. Results
3.1 Genetic identification of Artemia franciscana
Since inter-species sensitivity variations toward various toxins and environmental stressors have been shown among Artemia species (i.e. A. franciscana and A. salina),28 to avoid taxonomic inaccuracies, genetic identification of the organism employed in this study was initially carried out. Indeed, despite they belong to the same genus, correct species identification is of primary importance to outline specific responses to GO that could vary from species to species. The PCR sequencing of the COI gene region confirmed the identity of Artemia franciscana by BLASTn comparison with A. franciscana isolates (GenBank IDs: DQ119645 and MK393317), which resulted in 99% sequence identity. The sequence shared with that of Artemia salina ranged between 84.8 and 84.2%, allowing the exclusion of the species A. salina.
3.2 Effect of GO on A. franciscana viability
To evaluate the toxicity of GO toward A. fanciscana, nauplii or adults were exposed to different concentrations of GO (1, 10 and 100 μg mL−1) for 24, 48 and 72 h, or left untreated (0 μg mL−1; controls), and the mortality was evaluated under a stereomicroscope (Fig. 1). While no significant GO toxicity was observed towards nauplii at any concentration and exposure time (Fig. 1a), a significant increase of mortality (25%) was observed for A. franciscana adults after 72 h exposure to the highest GO concentration (100 μg mL−1), with respect to controls (p < 0.05) (Fig. 1b). The mortality of A. franciscana controls in adults was low (<5%) under each experimental condition, indicating the wellness of the organisms all throughout the assays.
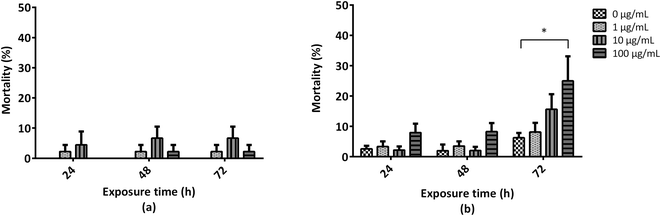 |
| Fig. 1 Mortality of A. franciscana nauplii (a) and adults (b) exposed to GO (1, 10 and 100 μg mL−1) for 24, 48 and 72 h. Data are presented as % of dead organisms with respect to the total number of organisms in the sample and are the means ± SE of at least three experiments. Statistical differences: * p < 0.05, Student's t-test as compared to controls (0 μg mL−1). | |
3.3 Effect of GO on A. franciscana as ROS production and glutathione S-transferase activity
To elucidate the mechanism underlying GO-induced mortality observed in A. franciscana adults, GO ability to induce oxidative stress was evaluated by means of increased ROS production, using the DCFDA assay. In addition, the activity of glutathione S-transferase (GST), one of the major xenobiotic detoxifying and antioxidant enzymes, was evaluated. As compared to the untreated controls, exposure of A. franciscana adults to GO (1, 10 and 100 μg mL−1) for 24 and 72 h did not induce any significant increase of ROS production (Fig. 2a). However, exposure of A. fanciscana adults to the highest GO concentration (100 μg mL−1) induced a significant time-dependent increase of GST activity with respect to untreated controls (156% and 264% increases after 24 and 72 h, respectively; p < 0.05) (Fig. 2b).
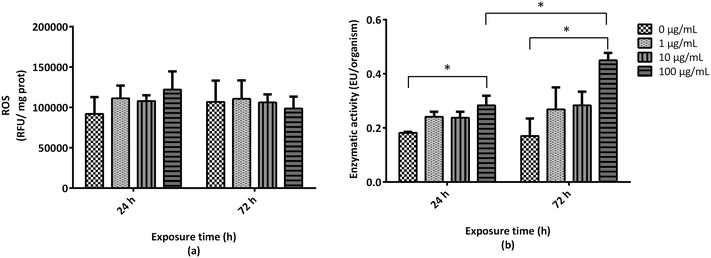 |
| Fig. 2 Oxidative stress induced by GO in A. franciscana adults. ROS production (a) and GST activity (b) in A. franciscana adults exposed to GO (1, 10 and 100 μg mL−1) for 24 and 72 h. Data are presented as relative fluorescent units (RFU) normalized on mg of proteins (a) and enzymatic units (EU) normalized on the total number of organisms (b), and are the means ± SE of at least three experiments. Statistical differences: * p < 0.05, Student's t-test. | |
3.4 Effects of GO on A. franciscana motility and cholinesterase activity
During the mortality test, reduced A. franciscana adults' motility was associated with exposure to GO, in particular at the highest concentration (100 μg mL−1). This finding could be related to physical impairment due to the presence of GO aggregates and/or agglomerates above the swimming appendages, as observed after 72 h exposure to the material (Fig. 3a). However, it could be related also to biochemically-mediated impairment of movements. Therefore, the activity of cholinesterase (ChE), a key enzyme involved in the regulation of acetylcholine reception at the neuromuscular sites, was evaluated. As compared to the untreated controls, no significant effects were observed on ChE activity after 24 or 72 h exposure to GO (1, 10, and 100 μg mL−1) (Fig. 3b).
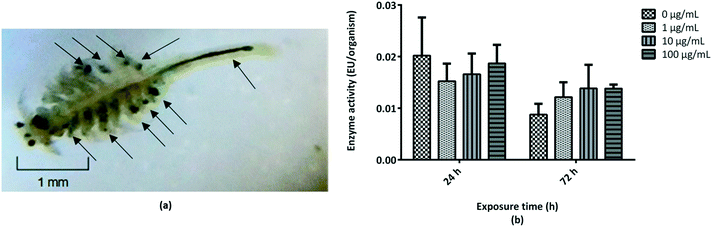 |
| Fig. 3 Representative picture of A. fanciscana adult exposed to 100 μg mL−1 GO for 72 h under a stereomicroscope (a); arrows indicate the presence of GO aggregates/agglomerates. Cholinesterase activity (b) in A. franciscana adults exposed to GO (1, 10 and 100 μg mL−1) for 24 and 72 h. Data of cholinesterase activity are presented as enzymatic units (EU) normalized on the total number of organisms and are the means ± SE of three experiments. | |
3.5 Effects of GO on A. franciscana adult growth
To evaluate the effects of GO on the growth rate of A. franciscana adults, the organisms were exposed to the material (1, 10 and 100 μg mL−1) for 24 and 72 h. Subsequently, the growth rate was evaluated measuring the protein content in the supernatant collected after centrifugation of the homogenized organisms (Fig. 4). As compared to the untreated controls, exposure to GO did not induce any significant difference in the protein content at any time of exposure, suggesting no effect on the shrimps' growth rate. Moreover, the protein content recorded after 72 h GO exposure was significantly higher than that recorded after 24 h both in the untreated controls and GO-treated organisms, confirming a physiologic growth rate of A. franciscana adults in the time frame.
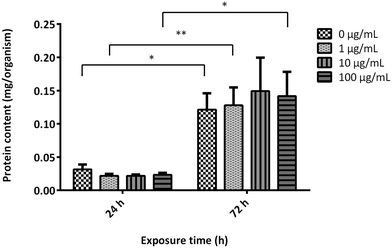 |
| Fig. 4 Protein content in A. franciscana adults exposed to GO (1, 10 and 100 μg mL−1) for 24 and 72 h as index of growth rate. Data are presented as mg of proteins normalized on the total number of organisms and are the means ± SE of five experiments. Statistical differences 24 vs. 72 h: * p < 0.05, ** p < 0.01, Student's t-test. | |
3.6 Uptake of GO by A. franciscana adults
The bioaccumulation of GO in A. franciscana adults after 24, 48 and 72 h exposure to 1, 10 and 100 μg mL−1 of the material was evaluated using a stereomicroscope, both in the presence and absence of food (it was added 24 h after exposure to GO). As shown in Fig. 5, adults exposed to the highest GO concentration (100 μg mL−1) accumulated black GO aggregates/agglomerates into the lumen of the gut (black arrows). GO accumulation into the gut appears to be higher in the organisms deprived of food as compared to the organisms with free access to food. The latter showed the presence of green food particles (green arrows) and only a slight accumulation of GO into the terminal part of the intestinal tract (black arrows). As expected, the untreated controls presented an empty intestine in the absence of food or green particles in the lumen after addition of food (green arrows). Similar findings were recorded also after 48 h exposure to all the GO concentrations. The uptake of GO by A. franciscana adults after 24 and 48 h exposure can be seen in the ESI† (Fig. S2).
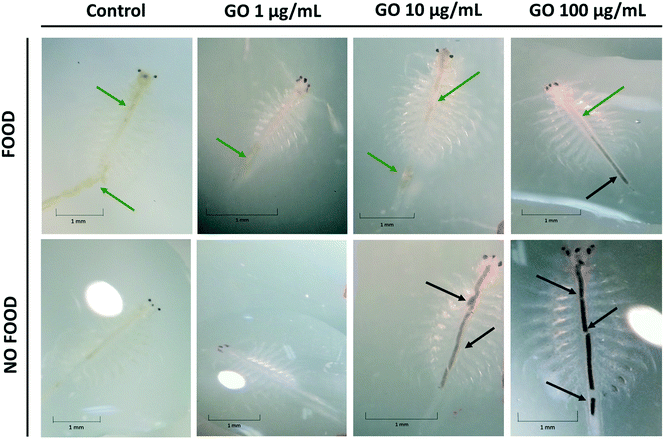 |
| Fig. 5 Uptake of GO by A. franciscana adults. Representative images of A. franciscana adults exposed to GO for 72 h, in the absence and in the presence of food, as well as of untreated A. franciscana controls (0 μg mL−1). The black and green arrows indicate GO and food into the lumen of the digestive tract, respectively. Pictures were acquired under a stereomicroscope at 1× magnification. Scale bars: 1 mm. | |
4. Discussion
GO is a GBM having a large spectrum of possible applications due to its peculiar physicochemical properties. However, some evidences of negative effects in different invertebrate and vertebrate organisms29 suggest a possible ecotoxicological impact of GBMs. In the aquatic environment, a negative impact has been observed for microalgae,30,31 the crustaceans Daphnia magna,32Ceriodaphnia dubia33 and Amphibalanus amphitrite,11 polychaete,9 and protozoa,34 as well as zebrafish embryos35,36 and adults.37 In general, carbon-based nanomaterials, including GBMs, are slightly toxic to most aquatic organisms and their half-maximal effect (EC50) ranges between 10 and 100 μg mL−1. However, their toxic effects depend on (i) the type of material, since the physicochemical properties and production methods can modulate the toxicity (e.g., size, functionalization and solvent used for the preparation); (ii) the sensitivity of each species (the most sensitive appeared to be algae, followed by crustaceans, fishes and bacteria) and (iii) time of exposure.38 Furthermore, it has to be considered that GO easily agglomerates in saline water and that an increased salinity over 10‰ decreases the sedimentation rate due to the formation of ramified agglomerates. Thus, in saline waters, GO could undergo some transformations affecting its potential effects on aquatic organisms.39
In the present work, A. franciscana was used as a model organism to study the possible ecotoxicological effect of GO, prospectively to its increasing future release into the environment. A. franciscana was exposed to GO at concentrations (1, 10 and 100 μg mL−1) selected on the basis of literature data on the toxicity of carbon-based nanomaterials to aquatic organisms.38
Initially, to evaluate the most sensitive developmental stage to GO, mortality was investigated exposing nauplii (instar I) or adults of A. franciscana to GO (1, 10 and 100 μg mL−1) for 24, 48 and 72 h. GO induced a weak but significant increase of mortality (25%) only in A. franciscana adults exposed to the highest material concentration for 72 h, demonstrating that the sensitivity of this species to GO is influenced by the developmental stage. In literature, a low but significant mortality (about 10%) is reported for A. franciscana stage I nauplii exposed for 24 h to carbon black, starting from a concentration of 100 μg mL−1.16 A similar mortality rate was observed under the same conditions for A. salina stage I nauplii exposed to oxidized multi-walled carbon nanotubes, with a lethal concentration for 50% of the exposed organisms (LC50) higher than 600 μg mL−1.40 However, our study showed no significant mortality of A. franciscana stage I nauplii exposed up to 100 μg mL−1 GO for 72 h, suggesting a lower toxicity of GO as compared to that of other carbon-based materials, such as carbon black or oxidized multi-walled carbon nanotubes. In addition, we cannot exclude that the low mortality of nauplii exposed to GO can be due, at least in part, to the fasting conditions under which they were exposed to the GBM. On the other hand, it cannot be excluded that this condition can imply an overestimated mortality, since fasting might increase the organism sensitivity to stressful stimuli. Therefore, it is reasonable to hypothesize that nauplii are less sensitive to GO toxic effects than adults.
To the best of our knowledge, no literature data comparing GO effects towards larval and adult stage of Artemia are reported. Our finding is in agreement with previous studies showing that Artemia stage I nauplii are more resistant to some xenobiotics, such as inorganic chemical reagents or elements (copper), with respect to more developed stages.41,42 This finding was recorded also for A. salina exposed to fullerene (C60): stage I nauplii were more resistant to this carbon material, while adults were more sensitive than the organisms at lower developmental stages, such as stage 2 nauplii, metanauplii (up to 96 h after hatching) and zoea (up to 7 days).43 In particular, in line with our observation, the few literature data on GO toxicity towards A. salina, limited to the larval stage, show an increased mortality of Artemia instar I nauplii only at GO concentrations higher than 100 μg mL−1.12–14 The resistance of larvae compared to adults is probably due to morphological and functional differences and metabolic states. For instance, instar I larvae lack of critical anatomical formations (e.g., mouth and anus), which start to develop at stage II, limiting the possibility of ingestion and the consequent bioaccumulation of GO.
The mortality of Artemia spp. exposed to carbon-based nanomaterials depends not only on their stage of development, but also on the type of nanomaterial, the methods of its production and its functionalization. In fact, the lethal potency of carbon black toward A. franciscana stage II nauplii after 24 h exposure (LC50 = 370 μg mL−1) was higher than that of functionalized carbon black by introduction of aryl-carboxylate groups from para-amino benzoic acid (LC50 = 1000 μg mL−1).16 As for the production methods, Kim et al. observed differences in mortality of A. franciscana stage I nauplii exposed for 24 and 48 h to carbon nanodots produced by the same synthetic method and precursors, but using different solvents.44
Considering that, in our study, GO induced slight, but significant, mortality only in A. franciscana adults, only the organisms at this developmental stage were investigated for their susceptibility to GO, evaluating other biomarkers and parameters of toxicity. One of the main mechanisms of GBM toxicity appears to be an increased ROS production and consequent oxidative stress, as previously demonstrated in human cells exposed to GO.45,46 Due to their chemical properties, ROS are reactive with different biological molecules, causing oxidative damage of proteins, lipids and DNA.47 Such effects were also recorded in A. salina and other organisms exposed to carbon-based nanomaterials, including GO, through the assessment of ROS production and the activity of antioxidant enzymes as biomarkers of oxidative stress.12,13,48 The antioxidant enzyme considered in this work is GST, a multifunctional enzyme involved in the detoxification of xenobiotics and ROS,49 also in Artemia.50,51 The activity of detoxification enzymes can be altered in response to xenobiotics, making them suitable markers of stress caused by xenobiotics52 and GST induction is part of an adaptive response mechanism to chemical stress, widely distributed in living organisms.53 In aquatic organisms, GST activity can be increased by exposure to different xenobiotics, including environmental contaminants such as pesticides and organophosphates.54
Hence, the possible role of oxidative stress in the mortality of A. franciscana adults induced by GO was evaluated. Despite GO did not induce any significant increase of ROS production up to 72 h exposure, its highest concentration (100 μg mL−1) caused a significant time-dependent increase of GST activity, detectable already after 24 h exposure and becoming more pronounced after 72 h. This result, and the evidence that the highest GST activity was recorded under the same GO exposure conditions inducing mortality of A. franciscana adults (100 μg mL−1 at 72 h), suggest a possible role of this enzyme in the attempt to detoxify the material and/or scavenge ROS. However, given that many antioxidant enzymes are present in Artemia (i.e. catalase, glutathione peroxidase, superoxide dismutase and others), we cannot exclude that also other intracellular antioxidant systems may contribute to ROS detoxification, besides GST. Notwithstanding, considering the increased GST activity induced by GO and the evidence that none of the exposure conditions (including those inducing mortality) caused a significant increase of ROS, we can hypothesize that Artemia death may not be associated with oxidative stress, therefore excluding ROS from the mechanisms of GO toxicity in this organism. Our results are in agreement with an increased GST activity induced by GO in other aquatic organisms, such as Crossostrea virginica after long exposure (14 days), which suggests up-regulation of detoxification enzymes as defence response to counteract the oxidative stress caused by GO.55 However, in contrast to our observations, Zhu et al. reported a slight but significant increase of ROS production and a decreased GST activity in the first larval stage of A. salina exposed to 100 μg mL−1 GO for 24 h.13 This discrepancy could be due to the use of different developmental stages or different Artemia species and/or to physicochemical differences between the two GOs. On the basis of the available physico-chemical characterization, the material used by Zhu was smaller and thinner (dimension up to 3 μm; number of layers < 3) than the GO used in this study (average lateral dimension of around 15 μm; number of layers = 6). However, no elemental analysis data, in particular the amount of O atoms, are available. Since the amount of O atoms is a key feature affecting GBM toxicity, further structure–activity relationship considerations cannot be extrapolated.
After GO exposure, decreased motility was also observed in A. franciscana adults, particularly at the highest concentration (100 μg mL−1). To evaluate if this effect was related to possible biochemically-mediated neurotoxicity leading to impaired organism motility, the activity of ChE as a key enzyme involved in the hydrolysis of the neurotransmitter acetylcholine was evaluated. ChE, in particular acetylcholinesterase, plays a key role in the regulation of the cholinergic signalling system and the maintenance of a regular neuromuscular function. This enzyme can be inhibited by neurotoxic xenobiotics, leading to an increased level of acetylcholine in the synapse with a consequent impaired neuromuscular function.56 However, no significant differences in this enzyme activity were observed after exposure of A. franciscana adults to GO. This finding is in line with a previous study showing that GO (100 μg mL−1) did not change ChE activity in Artemia nauplii after 48 h of exposure.12 These observations suggest that the decreased Artemia mobility is not related to impaired ChE activity as a biochemically-mediated effect triggered by GO but rather to physical blockage of the organisms by GO. This hypothesis is corroborated by the presence of GO aggregates/agglomerates above the Artemia body, likely hampering the motility of these organisms.
Further experiments investigating GO effects on A. franciscana growth were carried out evaluating the protein content in the organisms exposed to the material. In fact, in the crustacean Daphnia magna the protein content is considered a good indicator of the nutritional status and reflects the physiological conditions of the organism. For this reason, this parameter is used as an indicator of stress conditions and can be used to assess the toxic effects of environmental contaminants.57 The total protein content in Artemia reflects the metabolic conditions of the organism, and consequently the exposure to a xenobiotic could influence the protein level in response to stress and loss of energy, as shown after exposure to polystyrene nano-spherules.58 However, our results show that the protein content of A. franciscana was not significantly influenced by the exposure to any GO concentration for 24 and 72 h, suggesting no significant effect on the growth rate of this organism.
As a final step, we investigated if the toxic effects of GO on A. franciscana adults could be related to the ingestion of this material and if food could have a role in modulating GO ingestion. The ability of Artemia sp. to ingest several types of nanomaterials, such as carbon nanotubes,40 pristine graphene monolayer flakes,22 graphene oxide quantum dots,23 carbon black16 and GO (ref. 13) was already described. However, it should be noted that, in natural environments, A. franciscana is not exclusively exposed to GO, but more likely, also to its physiological feed (e.g. microplankton). This assumption led us to hypothesize that the low GO toxicity observed in our study could be related, at least in part, to its reduced intake and consequently to a reduced exposure level to this material, due to the presence of food. Thus, the presence of GO into the digestive tract of Artemia adults was verified in the presence or absence of food. The shrimps were able to ingest GO, but when the organisms were fed with their physiological nutrients, the presence of GO in the lumen of the digestive tract was decreased in relation to food. Therefore, the presence of food could reduce the organism exposure to GO by ingestion with consequent attenuation of its toxic effect.
Conclusions
The present study showed a weak toxic effect of GO on A. franciscana, an aquatic model organism used for ecotoxicological studies. The organisms at the adult stage were more sensitive than those at the nauplii stage I. Specifically, GO significantly increased the mortality of adults by 25% only at the highest concentration (100 μg mL−1) and 72 h exposure. The same GO concentration induced a time-dependent increase of GST activity, suggesting activation of the detoxification enzyme. However, other biochemical parameters, such as ROS production, ChE activity and protein content, were not affected in Artemia adults exposed to GO up to 72 h. Therefore, GO has a negative, albeit weak, impact on A. franciscana adults, lower than that of other carbon-based materials. Considering that the toxic effects of GO are observed only at very high concentrations and long exposure times, we can hypothesize a low ecotoxicological impact of the nanomaterial on this target organism. In fact, 100 μg mL−1 is a pretty high GO concentration and the lack of reliable data on GBM contamination level in the aquatic environment does not allow it to be considered a possible exposure level for aquatic organisms. Anyway, it cannot be excluded that such a level of exposure to GO could accidentally occur, such as in the case of sudden release of huge amounts of the nanomaterial within a limited timeframe. This hypothesis suggest that even if the ecotoxicological impact of GO on A. franciscana may be low in particular situations, this nanomaterial could affect this organism as part of the food chain in the aquatic system. Hence, we cannot exclude that a decrease, even if small, of the Artemia population consequent on GO effects could negatively impact the availability of food for predators. In addition, possible accumulation of the nanomaterial in Artemia could also lead to toxic effects in other organisms consequently to GO bioaccumulation along the food chain. However, further studies on other aquatic organisms are needed to expand the knowledge of the ecotoxicological impact of GO.
Conflicts of interest
The authors declare no conflict of interest.
Acknowledgements
This work was supported by the Graphene Flagship Core 2 and Core 3 grant agreement (no. 785219 and 881603, respectively). Part of this work was performed under the Maria de Maeztu Units of Excellence Program from the Spanish State Research Agency – Grant No. MDM-2017-0720. M. Prato is a recipient of the Carbon Bionanotechnology AXA Chair (2016-2023). The authors are grateful to the Applied and Comparative Genomics group of Prof. Alberto Pallavicini (University of Trieste, Department of Life Sciences) for the precious help in the genetic analysis.
References
- D. Plachá and J. Jampilek, Graphenic Materials for Biomedical Applications, Nanomaterials, 2019, 9, 1758 Search PubMed.
- X. Gong, G. Liu, Y. Li, D. Y. W. Yu and W. Y. Teoh, Functionalized-Graphene Composites: Fabrication and Applications in Sustainable Energy and Environment, Chem. Mater., 2016, 28, 8082–8118 CrossRef CAS.
- D. R. Dreyer, S. Park, C. W. Bielawski and R. S. Ruoff, The chemistry of graphene oxide, Chem. Soc. Rev., 2010, 39, 228–240 RSC.
- F. Barroso-Bujans, S. Cerveny, A. Alegría and J. Colmenero, Sorption and desorption behavior of water and organic solvents from graphite oxide, Carbon, 2010, 48, 3277–3286 CrossRef CAS.
- X. Deng, L. Lü, H. Li and F. Luo, The adsorption properties of Pb(II) and Cd(II) on functionalized graphene prepared by electrolysis method, J. Hazard. Mater., 2010, 183, 923–930 CrossRef CAS.
- C. Petit, B. Mendoza and T. J. Bandosz, Reactive adsorption of ammonia on Cu-based MOF/graphene composites, Langmuir, 2010, 26, 15302–15309 CrossRef CAS.
- T. S. Sreeprasad, S. M. Maliyekkal, K. P. Lisha and T. Pradeep, Reduced graphene oxide-metal/metal oxide composites: facile synthesis and application in water purification, J. Hazard. Mater., 2011, 186, 921–931 CrossRef CAS.
- L. J. Hazeem, M. Bououdina, E. Dewailly, C. Slomianny, A. Barras, Y. Coffinier, S. Szunerits and R. Boukherroub, Toxicity effect of graphene oxide on growth and photosynthetic pigment of the marine alga Picochlorum sp. during different growth stages, Environ. Sci. Pollut. Res., 2017, 24, 4144–4152 CrossRef CAS.
- L. De Marchi, V. Neto, C. Pretti, E. Figueira, L. Brambilla, M. J. Rodriguez-Douton, F. Rossella, M. Tommasini, C. Furtado, A. M. V. M. Soares and R. Freitas, Physiological and biochemical impacts of graphene oxide in polychaetes: The case of Diopatra neapolitana, Comp. Biochem. Physiol., Part C: Toxicol. Pharmacol., 2017, 193, 50–60 CAS.
- A. Katsumiti, R. Tomovska and M. P. Cajaraville, Intracellular localization and toxicity of graphene oxide and reduced graphene oxide nanoplatelets to mussel hemocytes in vitro, Aquat. Toxicol., 2017, 188, 138–147 CrossRef CAS.
- T. Mesarič, K. Sepčič, V. Piazza, C. Gambardella, F. Garaventa, D. Drobne and M. Faimali, Effects of nano carbon black and single-layer graphene oxide on settlement, survival and swimming behaviour of Amphibalanus amphitrite larvae, Chem. Ecol., 2013, 29, 643–652 CrossRef.
- T. Mesarič, C. Gambardella, T. Milivojević, M. Faimali, D. Drobne, C. Falugi, D. Makovec, A. Jemec and K. Sepčić, High surface adsorption properties of carbon-based nanomaterials are responsible for mortality, swimming inhibition, and biochemical responses in Artemia salina larvae, Aquat. Toxicol., 2015, 163, 121–129 CrossRef.
- S. Zhu, F. Luo, W. Chen, B. Zhu and G. Wang, Toxicity evaluation of graphene oxide on cysts and three larval stages of Artemia salina, Sci. Total Environ., 2017, 595, 101–109 CrossRef CAS.
- J. Lu, X. Zhu, S. Tian, X. Lv, Z. Chen, Y. Jiang, X. Liao, Z. Cai and B. Chen, Graphene oxide in the marine environment: Toxicity to Artemia salina with and without the presence of Phe and Cd2, Chemosphere, 2018, 211, 390–396 CrossRef CAS.
- B. S. Nunes, F. D. Carvalho, L. M. Guilhermino and G. Van Stappen, Use of the genus Artemia in ecotoxicity testing, Environ. Pollut., 2006, 144, 453–462 CrossRef CAS.
- A. L. Rodd, M. A. Creighton, C. A. Vaslet, J. R. Rangel-Mendez, R. H. Hurt and A. B. Kane, Effects of surface-engineered nanoparticle-based dispersants for marine oil spills on the model organism Artemia franciscana, Environ. Sci. Technol., 2014, 48, 6419–6427 CrossRef CAS.
- S. R. González and F. Vega-Villasante, Sensitivity of different stages of Artemia franciscana to potassium dichromate, Panam J. Aquat. Sci., 2019, 14, 8–12 Search PubMed.
- G. E. Belovsky, D. Stephens, C. Perschon, P. Birdsey, D. Paul, D. Naftz, R. Baskin, C. Larson, C. Mellison, J. Luft, R. Mosley, H. Mahon, J. V. Leeuwen and D. V. Allen, The Great Salt Lake Ecosystem (Utah, USA): long term data and a structural equation approach, Ecosphere, 2011, 2, art33 CrossRef.
- P. Léger, D. A. Bengtson, K. L. Simpson and P. Sorgeloos, The use and nutritional value of Artemia as a food source, Oceanogr. Mar. Biol., 1986, 24, 521–623 Search PubMed.
- G. Libralato, The case of Artemia spp. in nanoecotoxicology, Mar. Environ. Res., 2014, 101, 38–43 CrossRef CAS.
- S. A. Johari, K. Rasmussen, M. Gulumian, M. Ghazi-Khansari, N. Tetarazako, S. Kashiwada, S. Asghari, J.-W. Park and I. J. Yu, Introducing a new standardized nanomaterial environmental toxicity screening testing procedure, ISO/TS 20787: aquatic toxicity assessment of manufactured nanomaterials in saltwater Lakes using Artemia sp. nauplii, Toxicol. Mech. Methods, 2019, 29, 95–109 CrossRef CAS.
- C. Pretti, M. Oliva, R. D. Pietro, G. Monni, G. Cevasco, F. Chiellini, C. Pomelli and C. Chiappe, Ecotoxicity of pristine graphene to marine organisms, Ecotoxicol. Environ. Saf., 2014, 101, 138–145 CrossRef CAS.
- B. Murugesan, J. Sonamuthu, N. Pandiyan, B. Pandi, S. Samayanan and S. Mahalingam, Photoluminescent reduced graphene oxide quantum dots from latex of Calotropis gigantea for metal sensing, radical scavenging, cytotoxicity, and bioimaging in Artemia salina: A greener route, J. Photochem. Photobiol., B, 2018, 178, 371–379 CrossRef CAS.
- L. Fusco, M. Garrido, C. Martín, S. Sosa, C. Ponti, A. Centeno, B. Alonso, A. Zurutuza, E. Vázquez and A. Tubaro, Skin irritation potential of graphene-based materials using a non-animal test, Nanoscale, 2020, 12, 610–622 RSC.
- O. Folmer, M. Black, W. Hoeh, R. Lutz and R. Vrijenhoek, DNA primers for amplification of mitochondrial cytochrome c oxidase subunit I from diverse metazoan invertebrates, Mol. Mar. Biol. Biotechnol., 1994, 3, 294–299 CAS.
-
S. Z. Zulkifli, F. Z. A. Aziz, S. Z. M. Ajis and A. Ismail, in From Sources to Solution, ed. A. Z. Aris, T. H. Tengku Ismail, R. Harun, A. M. Abdullah and M. Y. Ishak, Springer, Singapore, 2014, pp. 233–237 Search PubMed.
- A. Jemec, T. Tisler, D. Drobne, K. Sepcić, P. Jamnik and M. Ros, Biochemical biomarkers in chronically metal-stressed daphnids, Comp. Biochem. Physiol., Part C: Toxicol. Pharmacol., 2008, 147, 61–68 Search PubMed.
- D. R. Ruebhart, I. E. Cock and G. R. Shaw, Brine shrimp bioassay: Importance of correct taxonomic identification of Artemia (Anostraca) species, Environ. Toxicol., 2008, 23, 555–560 CrossRef CAS.
- B. Fadeel, C. Bussy, S. Merino, E. Vázquez, E. Flahaut, F. Mouchet, L. Evariste, L. Gauthier, A. J. Koivisto, U. Vogel, C. Martín, L. G. Delogu, T. Buerki-Thurnherr, P. Wick, D. Beloin-Saint-Pierre, R. Hischier, M. Pelin, F. CandottoCarniel, M. Tretiach, F. Cesca, F. Benfenati, D. Scaini, L. Ballerini, K. Kostarelos, M. Prato and A. Bianco, Safety Assessment of Graphene-Based Materials: Focus on Human Health and the Environment, ACS Nano, 2018, 12, 10582–10620 CrossRef CAS.
- S. Du, P. Zhang, R. Zhang, Q. Lu, L. Liu, X. Bao and H. Liu, Reduced graphene oxide induces cytotoxicity and inhibits photosynthetic performance of the green alga Scenedesmus obliquus, Chemosphere, 2016, 164, 499–507 CrossRef CAS.
- J. Zhao, X. Cao, Z. Wang, Y. Dai and B. Xing, Mechanistic understanding toward the toxicity of graphene-family materials to freshwater algae, Water Res., 2017, 111, 18–27 CrossRef CAS.
- X. Guo, S. Dong, E. J. Petersen, S. Gao, Q. Huang and L. Mao, Biological uptake and depuration of radio-labeled graphene by Daphnia magna, Environ. Sci. Technol., 2013, 47, 12524–12531 CrossRef CAS.
- J. P. Souza, F. P. Venturini, F. Santos and V. Zucolotto, Chronic toxicity in Ceriodaphnia dubia induced by graphene oxide, Chemosphere, 2018, 190, 218–224 CrossRef CAS.
- C. Hu, Q. Wang, H. Zhao, L. Wang, S. Guo and X. Li, Ecotoxicological effects of graphene oxide on the protozoan Euglena gracilis, Chemosphere, 2015, 128, 184–190 CrossRef CAS.
- X. T. Liu, X. Y. Mu, X. L. Wu, L. X. Meng, W. B. Guan, Y. Qiang, S. U. N. Hua, C. J. Wang and X. F. Li, Toxicity of multi-walled carbon nanotubes, graphene oxide, and reduced graphene oxide to zebrafish embryos, Biomed. Environ. Sci., 2014, 27, 676–683 CAS.
- L. Chen, P. Hu, L. Zhang, S. Huang, L. Luo and C. Huang, Toxicity of graphene oxide and multi-walled carbon nanotubes against human cells and zebrafish, Sci. China: Chem., 2012, 55, 2209–2216 CrossRef CAS.
- J. P. Souza, J. F. Baretta, F. Santos, I. M. Paino and V. Zucolotto, Toxicological effects of graphene oxide on adult zebrafish (Danio rerio), Aquat. Toxicol., 2017, 186, 11–18 CAS.
- A. Freixa, V. Acuña, J. Sanchís, M. Farré, D. Barceló and S. Sabater, Ecotoxicological effects of carbon based nanomaterials in aquatic organisms, Sci. Total Environ., 2018, 619–620, 328–337 CAS.
- A. S. Adeleye, K. T. Ho, M. Zhang, Y. Li and R. M. Burgess, Fate and Transformation of Graphene Oxide in Estuarine and Marine Waters, Environ. Sci. Technol., 2019, 53, 5858–5867 CrossRef CAS.
- S. Zhu, F. Luo, X. Tu, W.-C. Chen, B. Zhu and G.-X. Wang, Developmental toxicity of oxidized multi-walled carbon nanotubes on Artemia salina cysts and larvae: Uptake, accumulation, excretion and toxic responses, Environ. Pollut., 2017, 229, 679–687 CrossRef CAS.
- P. Sorgeloos, C. Remiche-Van Der Wielen and G. Persoone, The use of Artemia nauplii for toxicity tests—A critical analysis, Ecotoxicol. Environ. Saf., 1978, 2, 249–255 CrossRef CAS.
- M. Toğulga, The short-term toxicity of two toxicants to Artemia nauplii, Turk. J. Zool., 1998, 22, 259–266 Search PubMed.
- S. R. R. Rajasree, V. G. Kumar, L. S. Abraham and N. Manoharan, Assessment on the toxicity of engineered nanoparticles on the lifestages of marine aquatic invertebrate artemia salina, Int. J. Nanosci., 2011, 10, 1153–1159 CrossRef CAS.
- T. H. Kim, J. P. Sirdaarta, Q. Zhang, E. Eftekhari, J. St. John, D. Kennedy, I. E. Cock and Q. Li, Selective toxicity of hydroxyl-rich carbon nanodots for cancer research, Nano Res., 2018, 11, 2204–2216 CrossRef CAS.
- M. Pelin, L. Fusco, C. Martín, S. Sosa, J. Frontiñán-Rubio, J. M. González-Domínguez, M. Durán-Prado, E. Vázquez, M. Prato and A. Tubaro, Graphene and graphene oxide induce ROS production in human HaCaT skin keratinocytes: the role of xanthine oxidase and NADH dehydrogenase, Nanoscale, 2018, 10, 11820–11830 RSC.
- J. Frontiñán-Rubio, M. V. Gómez, C. Martín, J. M. González-Domínguez, M. Durán-Prado and E. Vázquez, Differential effects of graphene materials on the metabolism and function of human skin cells, Nanoscale, 2018, 10, 11604–11615 RSC.
- M. Schieber and N. S. Chandel, ROS Function in Redox Signaling and Oxidative Stress, Curr. Biol., 2014, 24, R453–R462 CrossRef CAS.
- S. Zhu, B. Zhu, A. Huang, Y. Hu, G. Wang and F. Ling, Toxicological effects of multi-walled carbon nanotubes on Saccharomyces cerevisiae: The uptake kinetics and mechanisms and the toxic responses, J. Hazard. Mater., 2016, 318, 650–662 CrossRef CAS.
- K. D. Tew and Z. Ronai, GST function in drug and stress response, Drug Resist. Updates, 1999, 2, 143–147 CrossRef CAS.
- A. Vehovszky, H. Szabó, A. Acs, J. Gyori and A. Farkas, Effects of rotenone and other mitochondrial complex I inhibitors on the brine shrimp Artemia, Acta Biol. Hung., 2010, 61, 401–410 CrossRef CAS.
- A. I. Papadopoulos, E. Lazaridou, G. Mauridou and M. Touraki, Glutathione S-transferase in the branchiopod Artemia salina, Mar. Biol., 2004, 144, 295–301 CrossRef CAS.
- L. Lagadic, T. Caquet and F. Ramade, The role of biomarkers in environmental assessment (5). Invertebrate populations and communities, Ecotoxicology, 1994, 3, 193–208 CAS.
- S. Fernandes, M. Welker and V. M. Vasconcelos, Changes in the GST activity of the mussel Mytilus galloprovincialis during exposure and depuration of microcystins, J. Exp. Zool., Part A, 2009, 311, 226–230 CrossRef.
- I. Domingues, A. R. Agra, K. Monaghan, A. M. V. M. Soares and A. J. A. Nogueira, Cholinesterase and glutathione- S -transferase activities in freshwater invertebrates as biomarkers to assess pesticide contamination, Environ. Toxicol. Chem., 2010, 29, 5–18 CrossRef CAS.
- B. Khan, A. S. Adeleye, R. M. Burgess, S. M. Russo and K. T. Ho, Effects of graphene oxide nanomaterial exposures on the marine bivalve, Crassostrea virginica, Aquat. Toxicol., 2019, 216, 105297 CrossRef CAS.
- T. Olsen, L. Ellerbeck, T. Fisher, A. Callaghan and M. Crane, Variability in acetylcholinesterase and glutathione S-transferase activities in Chironomus riparius Meigen deployed in situ at uncontaminated field sites, Environ. Toxicol. Chem., 2001, 20, 1725–1732 CrossRef CAS.
- E. Sancho, M. J. Villarroel, E. Andreu and M. D. Ferrando, Disturbances in energy metabolism of Daphnia magna after exposure to tebuconazole, Chemosphere, 2009, 74, 1171–1178 CrossRef CAS.
- P. Mishra, S. Vinayagam, K. Duraisamy, S. R. Patil, J. Godbole, A. Mohan, A. Mukherjee and N. Chandrasekaran, Distinctive impact of polystyrene nano-spherules as an emergent pollutant toward the environment, Environ. Sci. Pollut. Res., 2019, 26, 1537–1547 CrossRef CAS.
Footnote |
† Electronic supplementary information (ESI) available. See DOI: 10.1039/d0en00747a |
|
This journal is © The Royal Society of Chemistry 2020 |
Click here to see how this site uses Cookies. View our privacy policy here.