Trophic transfer of CuO NPs from sediment to worms (Tubifex tubifex) to fish (Gasterosteus aculeatus): a comparative study of dissolved Cu and NPs enriched with a stable isotope tracer (65Cu)†
Received
2nd March 2020
, Accepted 23rd June 2020
First published on 26th June 2020
Abstract
Metal nanoparticles (NPs) released into the aquatic environment will likely accumulate in sediment and be available for sediment-dwelling invertebrates that serve as food for other organisms, such as fish. The aim of the present study was to investigate trophic transfer of copper oxide (CuO) NPs and dissolved Cu (CuCl2) from natural sediment to the sediment-dwelling worm Tubifex tubifex, and then to the predatory fish Gasterosteus aculeatus (three-spined stickleback). Cu enriched in the stable isotope 65Cu was used to increase detection and discriminate newly added/assimilated Cu from background Cu levels. Worms were exposed to sediment spiked with 65CuCl2 or 65CuO NPs (∼20 nm) at environmentally relevant concentrations for 7 days and subsequently fed to fish for 7 days. Worms accumulated 65Cu during sediment exposure to both 65CuCl2 and 65CuO NPs (0.7 and 1.1 μg 65Cu per g dw tissue, respectively), resulting in 65Cu body burdens significantly different from control. Furthermore, significantly more 65Cu was released from the sediment into the overlying water in the 65CuO NP exposures compared to the 65CuCl2 exposures. 65Cu accumulation in fish feeding on 65CuCl2 and 65CuO NP-exposed worms was limited (intestinal tissue: 80 and 65 ng g−1 dw; fish carcass: 7 and 10 ng g−1 dw, and liver: 50 and 10 ng g−1 dw, respectively). Glutathione peroxidase (gpx) mRNA expression was significantly higher in fish feeding on 65CuCl2-exposed worms compared to 65CuO NP-exposed worms (though 65Cu tissue concentrations were similar). No significant effects were detected for the other investigated genes (ctr1, mta, gcl, gr, sod, cat, zo-1). Our results show that NP-derived Cu can enter freshwater food webs, but bioaccumulation and trophic transfer under environmentally realistic exposures is low (detectable with a tracer) and not different from that of dissolved Cu.
Environmental significance
Engineered metal nanoparticles (NPs) released into the aquatic environment will likely accumulate in sediment, where they may be available for uptake by sediment-dwelling invertebrates such as T. tubifex that serve as food for higher trophic level organisms, such as fish. Through this dietary uptake NPs may be transferred in aquatic food webs with risk of biomagnification. In the present study, we compared transfer of 65CuO NPs with dissolved copper (added as 65CuCl2) in an experimental food chain involving sediment, sediment-dwelling worms, and predatory fish. Isotopically enriched 65Cu was used to enhance detection and discriminate between newly accumulated and background Cu, making it possible to use environmentally realistic Cu concentrations in sediment (100 μg Cu per g dw sediment). Our findings suggest that dietary transfer of metal NPs may occur to a limited extent in freshwater environments.
|
1. Introduction
Over the past few decades, there has been a dramatic increase in the industrial production and use of copper (Cu)-bearing nanoparticles (NPs), such as Cu, CuO, Cu2O and Cu(OH)2 NPs.1 For CuO NPs, the total production amount was estimated to be 20 tons in Europe in 2016, of which less than 1% was predicted to end up in the aquatic environment.2 Possible entry routes of these NPs into the aquatic environment include urban stormwater, untreated wastewater, wastewater treatment plants effluents,2–4 runoff from agricultural land treated with NP-containing biosolids and pesticides,3,5,6 and release from weathered surfaces treated with NP-containing wood coatings or antifouling paints.2,7 After release into natural water bodies, Cu-bearing NPs are likely to undergo a series of transformations that may affect their fate, bioavailability, and toxicity. For instance, they are likely to aggregate, settle out and accumulate in sediments.8–12 Furthermore, dissolution may occur in both water and sediment. CuO NPs tend to dissolve less than other Cu-bearing NPs and may thus prevail in the environment.8,12–17 Using a multimedia fate and transport model simulating ten years of release of manufactured nanomaterials including CuO NPs in the San Francisco Bay area, Garner et al. (2017) predicted that CuO NPs will accumulate in freshwater and marine sediment beds potentially reaching concentrations in the ng kg−1 to μg kg−1 range.4 Similarly, Caballero-Guzman and Nowack, estimated that alone the use of CuO NP-containing wood coatings in Europe could result in an increase of their concentration in sediments by about 400 ng kg−1 anually.2 The increasing occurrence of CuO NPs in sediment beds augments the likelihood for exposure of benthic deposit-feeders. Previous research has established that sediment-associated CuO NPs are available for endobenthic worms such as Nereis (Hediste) diversicolor and Lumbriculus variegatus living in estuarine and freshwater systems.9,18,19 Since benthic invertebrates represent a major food source for fish, it is hypothesized that CuO NPs may be further transferred along the food chain.
Today a range of studies have focused on the fate of manufactured nanomaterials in aquatic food webs (for reviews see ref. 20 and 21) but only a few on their transfer along food chains including predatory fish as a secondary consumer.22–32,33 Furthermore, most studies focused on pelagic food chains using, for instance, artemia,22,25,27,29,32 or daphnia23,24,28,31 as the model prey, and zebrafish (Danio rerio) as the model predator.23–28,31,32 An exception is published by Wang et al. (2016), who studied the trophic transfer of TiO2 NPs in a marine benthic food chain from the clamworm (Perinereis aibuhitensis) to juvenile turbot (Scophthalmus maximus).30 The authors reported trophic transfer, but no biomagnification of TiO2 NPs between trophic levels. Even though metal(oxide) NPs constitute the biggest group of manufactured nanomaterials that has been tested in food chains including fish,22,23,25,28–32 CuO NPs appear to have been used in only one study so far.22 The authors found that dietary exposure was a likely uptake route of CuO NPs and that accumulation primarily occurred in the intestine. There are only a few studies assessing dietary uptake of nanoparticulate Cu in fish, and findings concerning the intestinal absorption efficiency and accumulation in inner tissues compared to dissolved Cu species vary.34–36 Some information on NP transfer from invertebrate prey organisms to fish can be inferred from studies that examined intestinal uptake and accumulation of metal(oxide) NPs from spiked artificial diets.37–45 Altogether, the data from the few studies published on dietary exposure of fish to Cu(O) NPs suggest that Cu from NP-spiked diet is available, but uptake is limited compared to dissolved Cu.34,38,40,41 The present study is a continuation of such a study where we examined Cu uptake from Cu salt and CuO NPs in spiked artificial diets (worm homogenates).42 Such test design provides considerable logistical advantages, as well as the possibility of strict control of the exposure dose etc. However, it is important to bear in mind that these studies ignore physical, chemical, and biological processes occurring in the environment and the influence that the prey organisms may play in altering the availability of NPs to predators.
In a review, Tangaa et al. defined four processes that influence trophic transfer of metal NPs: environmental transformations of metal NPs, uptake and accumulation in the prey organism, internal fate and localization in the prey, and the digestive physiology of the predator.20 Therefore, in the present study, fish were exposed via live worms to increase environmental realism of the exposure scenario regarding both food type (live worms), complexation with biological tissue and realistic exposure dose (dependent on actual bioaccumulation of Cu in worms during sediment exposure).
The objective of this work was to study whether transfer of CuO NPs from sediment to worms (prey) to fish (predator) occurs at environmentally relevant exposure concentrations and conditions. In addition, the aim was to evaluate if potential changes in gene expression resulting from NP exposure could be detected. Dissolved Cu applied as CuCl2 was used as a reference (soluble Cu counterpart). CuO NPs and CuCl2 enriched in the stable isotope 65Cu (65CuO NP and 65CuCl2)34 were used to trace the transfer of Cu along the different compartments of the simulated food chain (sediment, worms, and fish). The model prey and predator organisms chosen for this study were the sediment-dwelling freshwater oligochaete Tubifex tubifex and the fish three-spined stickleback (Gasterosteus aculeatus). T. tubifex is a widely used standard test organism for bioaccumulation testing,46 and is, as a deposit-feeder, at particular risk of exposure to sediment-associated metal NPs and metals. In addition, previous research has established that T. tubifex is relatively insensitive to Cu-induced toxicity and can accumulate high amounts of the metal before lethality occurs.47–51 This makes it a good model prey organism for studying trophic transfer of Cu including Cu-bearing NPs. Three-spined stickleback (Gasterosteus aculeatus) is a widely used fish model in ecotoxicology and as a bottom-feeder a natural predator of tubificid worms.52–54
2. Methods
2.1 Synthesis and characterization of 65CuO NPs
The synthesis of 65CuO NPs were described previously.34 In brief, the metallic precursor 65Cu (isotope purity >99%, Trace Sciences International, USA) was dissolved in a mixture of HCl and H2O2. Solvent removal on rotatory evaporator under vacuum at 90 °C yielded a powder of 65CuCl2. 65Cu NPs were synthesized by thermolysis of 65Cu-oleate obtained by reflux of a mixture of 65CuCl2 and sodium-oleate in water, ethanol and hexane. 65CuO NPs were produced by oxidation of the 65Cu NPs, and were finally dispersed in 1 mmol L−1 sodium citrate solution. All data on the characteristics of the 65CuO NPs including size (20 nm; TEM; JEOL2100F transmission electron microscope), shape (spherical) and surface chemistry (mixture phase of CuO, Cu2O, and Cu; X-ray diffraction) are shown in Lammel et al. (2020).34
2.2 General experimental design of trophic transfer study
An overview of the overall experimental design is given in Fig. 1. Trophic transfer of 65CuO NPs and dissolved 65Cu (65CuCl2) from sediment to worms to fish was examined by conducting two successive dietary exposures, each lasting 7 days. In the first exposure, T. tubifex were exposed to clean sediment and sediment spiked with 65CuO NPs or 65CuCl2. At the end of this exposure, the worms were recovered from the exposure vessels and used as live food for three-spined sticklebacks (second exposure). In total, seven separate repetitions of the worm exposure were carried out. The repetitions were staggered by one day to ensure that, each day, fish received worms that had been exposed for the same duration (Fig. 1B).
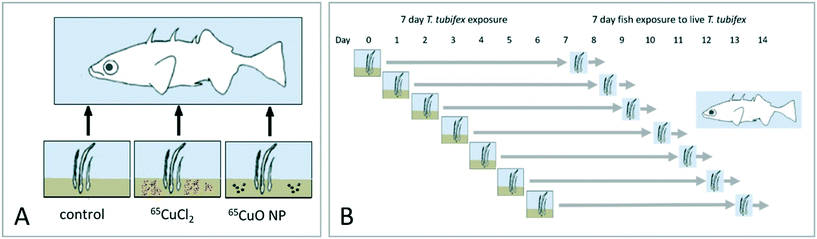 |
| Fig. 1 Overall experimental design. A. Illustration of the overall approach where worms were exposed to sediment and subsequently fed to fish. B. New T. tubifex exposures were initiated daily for seven days. Each worm exposure was terminated after seven days and worms were fed to fish. Thus, dietary fish exposures were initiated seven days after the first worm exposure was initiated. | |
2.3 Worm exposure
2.3.1 Experimental organism, T. tubifex.
T. tubifex (EAN 4038358100154, Batch-No.: 1518413 produced by Live is Life for Zooschatz, Berlin, Germany) were purchased through ZooCenter Bäckebol (Hisings Backa, Göteborg, Sweden). The worms were reared in the laboratory in glass beakers (250 mL Schott bottles) containing 150 mL T. tubifex medium (described below) continuously aerated by a glass Pasteur pipette connected by silicon tubing to the in-house pressurized air system. The culture was kept at 17 °C at a 12 h/12 h darkness/light cycle (conditions in the aquarium facility).
2.3.2 Tubifex medium preparation.
Tubifex medium (artificial freshwater) was prepared as specified in OECD Guideline 203. In brief, 25 mL of a calcium chloride stock solution (11.76 g L−1 CaCl2–2H2O, 10043-52-4, Merck), a magnesium sulfate stock solution (4.93 g L−1 MgSO4–7H2O, 10034-99-8, Sigma-Aldrich), a sodium bicarbonate stock solution (2.59 g, NaHCO3, 144-55-8, Merck) and a potassium chloride stock solution (0.23 g L−1 KCl, 7447-40-7, Merck) were mixed and the total volume made up to 1 L with deionized water. The final solution was kept at 17 °C and aerated for at least 24 h before being used.
2.3.3 Sediment preparation.
Sediment was collected from Roskilde Fjord, Munkholmbroen (55°40′25′′N 11°48′44′′E) in October 2016 by scraping of the top few centimeters of the sediment surface and subsequently sieved at the site (<0.5 mm). After settling, the overlying water was removed and the sediment was frozen at −20 °C to kill macro- and meiofauna. Thereafter, the sediment was thawed, sieved to <125 μm, rinsed with fresh T. tubifex medium and stored at 4 °C until experimental use. Dry weight (dw) to wet weight (ww) ratio determined using a Metler Toledo moisture analyzer was 0.374 ± 0.001 (mean ± sd, n = 2). The background Cu concentration of the sediment (<125 μm) was 29.4 ± 3.7 μg Cu per g dw sediment (n = 7; Cu concentrations inferred by either of the two naturally occurring stable Cu isotopes, 63Cu or 65Cu, as described below).
For exposures, sieved sediment (about 240 g ww sediment per treatment) was spiked with 65CuO NPs or 65CuCl2 at a nominal concentration of 100 μg 65Cu per g dw sediment (the measured concentrations were 88 and 95 μg 65Cu per g dw sediment, respectively; see Table 2). In addition, control sediment was prepared. This sediment was spiked only with the solvent in which 65CuO NPs and 65CuCl2 spiking solutions were prepared (2 mM citrate solution, see above). To obtain a homogenous Cu distribution, the sediment was first stirred by hand and then by automated shaking at 300 rpm and room temperature overnight (Heidolph Unimax 1010 Orbital Shaker). Thereafter, the sediment was kept at 4 °C until use for exposures (one week).
2.3.4 Exposures of T. tubifex to 65CuO NP and 65CuCl2-spiked sediment.
Initiation of T. tubifex exposures.
Beakers (100 mL Schott bottles, 56 mm in diameter) were loaded with 3 g of natural non-contaminated (control), 65CuCl2- or 65CuO NP-spiked sediment (100 μg 65Cu per g dw sediment; see above). Subsequently, 100 mL of aerated tubifex medium was added to the beakers and the sediment left to settle for 24 h. Before experimental start, the overlying water was carefully removed and replaced with fresh aerated tubifex medium. Then, ∼1.2 g ww healthy and intact T. tubifex of similar size were selected, sub-divided into three portions of ∼0.4 g ww each, and added to the exposure beakers. A random sub-sample of ten worms was taken and their ww determined to ensure that worm sizes were comparable between treatment groups and independent repetitions (Table S1†). T. tubifex exposures lasted seven days and were carried out in a room with controlled air temperature (17 °C). The overlying water was gently aerated (∼1–2 bubbles per second) throughout the experiment via a glass Pasteur pipette positioned 2 cm above the sediment surface. The temperature, dissolved oxygen content and conductivity of the water overlying the sediment was 17.0 ± 0.1 °C, 94.3 ± 1.0% and 553.9 ± 6.2 μS cm−1, respectively.
Sampling of overlying water and sediment.
For three of the seven independent repetitions, sub-samples (1 mL) of overlying water of each exposure vessel were taken at experimental day 0, 1, 4, and 7 to determine 65Cu remobilization from sediment into water (determined by inductively coupled plasma-mass spectrometry, ICP-MS; see below). The samples were taken from four different locations (4 × 250 μl) around the beaker's circumference (staggered by 90°) and ∼1.5 cm above the sediment surface. In addition, representative samples of sediment (0.2–0.3 g ww) were taken of each exposure vessel at the end of the exposure. All samples were stored at −20 °C until analysis.
Sampling of worms.
At the end of experimental day 7, worms were carefully sampled from the exposure vessels using a 1 mm sieve. The worms were collected in a beaker with fresh aerated tubifex medium and all sediment associated with the outer surface of the worms was removed by repeatedly rinsing and transferring the worms into new beakers with clean aerated tubifex medium. Following determination of the total number and weight of worms recovered from each exposure (data shown in Table S2†), the cleaned worms were incubated for additional 20 h to purge the gut from sediment particles and unassimilated Cu, and then fed to fish (see below). A sub-sample of ten purged worms of each group was used to determine the average body ww of individual worms (data shown in Table S3†) needed to calculate the number of worms corresponding to the fish' food ration (representative images of proportionated purged T. tubifex fish were fed during exposures are shown in Fig. S2†). Moreover, two purged worms of each treatment group and independent repetition were set aside for quantification of 65Cu body burden using ICP-MS (n = 14).
2.4 Fish exposure
2.4.1 Experimental organism: three-spined stickleback (Gasterosteus aculeatus).
Three-spined sticklebacks (G. aculeatus) were caught from a small channel (58° 13′ 57.4′′ N, 11° 28′ 22.9′′E) close to the marine research station Kristineberg, Sven Lovén Centre for Marine Infrastructure, on the island of Skaftö on the Swedish west coast in September 2018. The fish were transported to the animal facility at the Department of Biological and Environmental Sciences, University of Gothenburg, where they were transferred to an acclimatization tank with environmental enrichment, aeration, and ∼14 °C-cold, brackish water (∼18 ppt). After one week, the fish were slowly acclimatized to freshwater by gradually replacing the brackish water with filtered, UV-treated tab water (∼155 μS cm−1, ∼10 °C) originating from the in-house semi-recirculating system. The fish were maintained at these conditions under continuous flow-through and were fed ad libitum with frozen blood worms (Röda Mygglarver, AkvarieTeknik) until used for experimentation in November 2018. All animal procedures were performed in accordance with national and international guidelines for care and use of laboratory animals following the policy for animal experimentation of the University of Gothenburg and were approved by the Animal Ethics Committee of the Swedish Board of Agriculture (Ethical permit 15986-2018).
2.4.2 Dietary exposure of three-spined sticklebacks to live 65CuO NP and 65CuCl2-exposed T. tubifex.
Two weeks before experimental start, 45 fish with similar weight were selected and transferred to an experimental flow-through aquaria system (fish were of male and female sex; see Table S5† for sex distribution of analyzed fish). The experimental tanks contained 1.5 L of filtered, UV-treated tap water (10 °C) supplied by the in-house semi-recirculating system. The water turnover in the experimental tanks was ∼0.1 L min−1. Each tank contained one fish and was assigned to one of the three treatment groups (control, 65CuCl2, 65CuO NP). During the first seven days of the study (experimental day 1–7) all fish were fed live, uncontaminated T. tubifex collected from the T. tubifex culture (see above). On experimental day 8, feeding was suspended so that fish could empty their guts and the tanks be cleaned from feces before starting with the dietary exposure. From experimental days 9 to 15, the fish were fed live T. tubifex, which were previously exposed to non-contaminated (control), 65CuCl2-spiked or 65CuO NP-spiked sediment for seven days (see above). Each fish was fed six to seven worms each day corresponding to an average daily food ration of approximately 26 ± 0.4 mg and 4% of the fish body ww (0.627 ± 0.114 g, n = 45; the average body ww between treatment groups was similar). More detailed information on the number, weight and 65Cu body burden of worms fed to each fish on each day is shown in Table S5.† In addition, feces egested by each fish were collected at the end of each experimental day (i.e., prior to feeding) and stored at −20 °C until ICP-MS analysis. Furthermore, water samples (50 mL) were taken from three experimental tanks of each treatment group on a daily basis. Water samples originating from tanks belonging to the same treatment group were pooled and analyzed by ICP-MS to determine Cu background levels and control for potential 65Cu release from feces. The temperature, dissolved oxygen content and conductivity of the water in the flow-through system were monitored daily and were 10.0 ± 0.2 °C, 79.3 ± 3.3% and 159.3 ± 4.3 μS cm−1, respectively. Furthermore, acidity (pH), general hardness (GH), carbonate hardness (KH), nitrite (NO2−), nitrate (NO3−) and chlorine (Cl) levels of the water in the system were controlled at the beginning of the experiment and the end of the exposure. All parameters were within the quality criteria (pH: 6.8 to 7.2, GH: >4°, KH: 3 to 6°, NO2−: 0 mg L−1, NO3−: 0 to 10 mg L−1, Cl: 0 to 0.8 mg L−1). There were no significant differences among treatment groups.
2.4.3 Sampling and tissue dissection.
After the 7 day exposure period (end of experimental day 15), all fish were stunned by a sharp blow to the head and killed by transection of the spinal cord. Following determination of the body ww and standard length, liver and intestine were excised. The intestine was gently flushed with a physiological buffer solution to remove any remaining content (chyme). Liver and intestinal tissue samples of each fish were split into a small tissue sample for gene expression analysis (abscised from the proximal end of the intestine and the distinct, pointy end of the liver) and a large tissue sample for ICP-MS analysis. Tissue samples were placed in cryotubes, snap-frozen and stored in liquid nitrogen. The remainders of the fish (in the following referred to as carcass) were frozen on dry ice and stored at −80 °C. Tissue samples of 8 of the 15 fish in each treatment group were analyzed (see below).
2.5 Gene expression analysis
Liver and intestine samples were homogenized in RLT plus buffer using a TissueLyser II apparatus and stainless-steel beads (5 mm) according to the manufacturer's instructions (all stated materials and instruments were from Qiagen). Total RNA was isolated using RNeasy Mini Kit (Qiagen). RNA concentration and purity were measured using a NanoDrop 2000c spectrophotometer (ThermoFisher). cDNA was synthesized using the iScript™ cDNA synthesis kit from BioRad. Quantification of relative mRNA expression levels (cDNA copy numbers) was carried out using SsoAdvanced™ Universal SYBR Green Supermix (BioRad) and appropriate primer pairs (Eurofins Genomics, Ebersberg, DE).42 The sequence and concentrations of the oligonucleotide primers, and the efficiencies of the respective qPCR (quantitative polymerase chain reaction) assays are presented in Table 1. All qPCR reactions were run in duplicate on a Bio-Rad CFX Connect™ Real-Time System (one cycle of 3 min-cycle at 95 °C followed by 40 cycles of 10 s at 95 °C (denaturation) and 30 s at 60 °C or 62 °C (annealing and extension)). ΔCq values were calculated subtracting the mean Cq value of each target gene by the average of the mean Cq values of the two reference genes.
Table 1 List of analyzed reference and target genes and information on primers used for qPCR including sequence, concentration, annealing temperature and efficiencies of optimized assays
Gene name and abbreviation |
Sequence (5′ ≥ 3′) of forward (fw) and reverse (rv) primers |
Conc. [nM] |
Temp. [°C] |
Eff. [%] |
Ref. |
Oligonucleotide sequence was shortened.
|
β-Actin (β-act) |
fw |
CTGTCTTTCCCTCCATCGTC |
500 |
60 |
103.4 |
55
|
rv |
CTCTTGCTCTGGGCTTCATC |
500 |
Ubiquitin (ubq) |
fw |
AGACGGGCATAGCACTTGC |
500 |
60 |
93.4 |
56
|
rv |
CAGGACAAGGAAGGCATCC |
500 |
Tight junction protein 1a (zo-1) |
fw |
CTCTCTTAGGAGGCCCACCA |
250 |
62 |
98.6 |
42
|
rv |
TCTCCCCGTGTTTTCTACGC |
250 |
High affinity copper transporter (ctr1) |
fw |
TCAACGTCCGCTACAACTCC |
500 |
60 |
103.0 |
42
|
rv |
ACCTGGACGATGTGCAACAG |
500 |
Metallothionein-A (mta) |
fw |
CCCCTGCTGCCCGACTG |
500 |
60 |
102.4 |
57
|
rv |
TGTTCAAACTGCCGCCATCTC |
500 |
Glutamate-cysteine-ligase, catalytic subunit (gcl) |
fw |
CGTGTTGAAATGGGGCGATG |
250 |
60 |
104.8 |
42
|
rv |
TCCAAAGGGTGGGGTGATTG |
250 |
Glutathione reductase (gr) |
fw |
GCTGCAAAACTCTGGTGTGG |
500 |
60 |
98.0 |
42
|
rv |
CATTTCCAAACCCATGGCGG |
500 |
Glutathione peroxidase (gpx) |
fw |
ATCAGGAGAACTGCAAGAATGAAG |
100 |
60 |
110.3 |
58
|
rv |
GTTCACCTTCTCAAGGAGCTG |
100 |
Superoxide dismutase-1 (sod-1) |
fw |
AGCAGGAGAGCGATAAAGCG |
250 |
60 |
102.5 |
42
|
rv |
TCATCATTAGGGCCTGCGTG |
250 |
Catalase (cat) |
fw |
ACCAAGGTTTGGTCCCACAAAG |
500 |
60 |
102.4 |
58
|
rv |
TGCTCCACCTCTGCAAAGTAG |
500 |
2.6 Concentrations of background Cu and newly accumulated 65Cu
2.6.1 Sample analysis with ICP-MS.
Cu concentrations in fish and worm tissues, sediment, water, and fish feces were measured by ICP-MS (7900, Agilent). Samples were dried in an oven at 40 °C for at least 24 h. Larger samples (fish carcass and sediment samples) were weighed into Weflon™ vials (Milestone, Germany) and dissolved with 65% ultrapure nitric acid (HNO3) and Milli-Q water (1
:
1) in a volume of 6.25 mL. All smaller sized samples were weighted into Teflon™ inserts, which made it possible to dissolve samples in smaller volumes of HNO3 and Milli-Q water (1
:
1) (0.625 mL). Three Teflon™ inserts were placed in each Weflon™ vial containing 10 mL Milli-Q water and 2 mL 30% H2O2. All samples were subsequently digested according to ISO15587-2. Briefly, samples were heated in a microwave oven (Start D microwave digestion system, Milestone, Germany). All samples were then transferred into volumetric flasks, resulting in a known volume resulting in 8% HNO3. Finally, the Cu concentration in each sample was determined directly after digestion or after a short storage period (<48 h). A series of standard Cu solutions (8% HNO3) was used to calibrate Cu concentrations (six standards were selected from 0, 0.1, 2, 5, 10, 50, 100, and 1000 μg Cu per L to cover the range of expected Cu concentrations in the sample batch). During each analytical run, at least one of these standards were re-analyzed (approximately for every ten samples analyzed) to check for analytical drift and were all in agreement with expected Cu concentrations. Samples were analyzed in duplicate (each analysis averaged 32 measurements) for the naturally occurring stable isotopes 63Cu and 65Cu by ICP-MS (Agilent) as described in Lammel and Thit et al. (2019).42 Samples were set to zero if their Cu concentrations inferred from 63Cu were higher than those of 65Cu.
Cu recovery was examined using certified mussel tissue (European reference materials, ERM® – CE278k; 5.98 μg Cu per g dw tissue), and certified freshwater sediment (RIZA, Trace metals WD CRM-CNS301-050; 44.2 μg Cu per g dw sediment), which were digested and analyzed together with different sample batches (at least one reference sample per batch). Results of certified tissue and sediment were in good agreement with the certificate of analysis (5.54 ± 0.27 μg Cu per g dw tissue, n = 4 and 41.45 ± 2.30 μg Cu per g dw sediment, n = 4, respectively). All equipment used for sample digestions was thoroughly acid-washed before use.
2.6.2 Calculation of concentrations of newly accumulated 65Cu.
Concentrations of newly accumulated or added 65Cu (referred to as 65Cu in the following) were calculated based on ICP-MS measurements of the two naturally abundant stable isotopes, 65Cu, and 63Cu, as described in Croteau et al. (2004).59 Briefly, the relative abundance of the two isotopes in natural Cu samples in the absence of a spike (p65) was set to 0.309.60 Concentrations of newly accumulated 65Cu in the experimental organisms ([65Cu]org) were calculated as the product of p65 and the total Cu concentrations inferred by the ICP-MS software from 65Cu intensity ([T65Cu]). | [65Cu]org = p65 × [T65Cu] | (1) |
The original load of tracer ([65Cu]org0) that occurred in each sample in the absence of a 65Cu spike was calculated as the product of p65 and the total Cu concentrations inferred from the intensity of the most abundant Cu isotope, (i.e., [T63Cu]): | [65Cu]org0 = p65 × [T63Cu] | (2) |
The net tracer uptake ([65Cu]org) was derived from the total Cu concentration inferred from 65Cu signal ([65Cu]org minus the pre-existing concentration of tracer ([65Cu]org0)): | Δ[65Cu]org = [65Cu]org − [65Cu]org0 = p65 × ([T65Cu] − [T63Cu]) | (3) |
2.7 Statistical analysis
In general, data are presented as mean ± sd (standard deviation) unless otherwise stated. One-way Analysis of Variance (one-way ANOVA) was conducted when data met the test's requirements for normality and equal variances. When requirements for parametric analysis were not met, non-parametric Kruskal–Wallis Analysis on ranks was conducted. The strength of the association between pairs of variables (for instance, 65Cu concentration and target gene expression level) was measured by Pearson product–moment correlation analysis. Student's t-test were conducted to test the null hypothesis that the mean 65Cu concentration in the overlying water of 65CuCl2 and 65CuO NP sediment exposures are equal. Data for 65Cu concentrations in control treatment were zero/close to zero and hence failed the normality test. Thus, comparison by one-way ANOVA could not be performed. Kruskal–Wallis Analysis on ranks had too little statistical power to detect significant differences between treatments because of the low sample size and a Student's t-test was conducted. Statistical analysis was conducted using SigmaPlot for Windows Version 14.0 (Systat Software, Inc., San Jose, CA, USA).
3. Results and discussion
3.1 Bioaccumulation study of sediment-associated 65Cu and 65CuO NP in worms
65Cu concentrations in sediment and water.
The nominal sediment exposure concentrations (∼100 μg 65Cu per g dw sed) were selected on the basis of a worst-case scenario, where CuO NP concentrations in the sediment were assumed to be as high as the concentrations of non-particulate Cu in some sediments in Europe today (often 5–50, but reaching up to 4000 μg Cu per g dw sed at contaminated sites).48,61 There was no significant difference between the measured sediment 65Cu concentrations of the two 65Cu treatments, though they were slightly higher in the sediment spiked with 65CuCl2 compared to 65CuO NP (by approximately 7%; Table 2). The bioturbation activity of T. tubifex resulted in resuspension of sediment particles (Fig. S1†), but only little 65Cu was remobilized into the overlying water. Hence, it can be assumed that exposure to waterborne 65Cu was negligible during the experiment (Table 2). Interestingly, the mean 65Cu concentration measured in the overlying water at day 7 was significantly higher in the 65CuO NP exposure than in the 65CuCl2 exposure (p = 0.018, Student's t-test). Several processes leading to remobilization of Cu (as nanoparticulate or dissolved form) may have been affected differently by the two 65Cu treatments. 1) The conveyer-belt feeding behavior of T. tubifex, where sediment is ingested at depth and egested as fecal matter above the sediment surface,62 can increase the concentration of metals in top sediment layers facilitating their remobilization into the overlying water.63,64 Thus, differences in remobilization between treatments may be owed to differences in feeding activity (resulting in a lower amount of fecal pellets deposited on the surface in 65CuCl2-treatment) or differences in gastrointestinal 65Cu absorption18,42 (resulting in lower 65Cu concentration in fecal pellets egested in 65CuCl2-treatment). 2) Cu stress can induce caudal autotomy (tail loss),49 which precludes worms from defecating until the posterior end has been regenerated and is operational again (often after more than five days).65 We did not specifically investigate this endpoint, but if exposure to dissolved Cu had caused caudal autotomy, egestion would have been decreased, resulting in decrease Cu remobilization. 3) Cu exposure has been reported to reduce locomotion and bioturbation activity of tubificid worms at sublethal concentrations (down to 10 μg Cu per g dw sediment),66,67 and may affect remobilization of Cu. Bioturbation by T. tubifex likely increases the transport of metals from sediment to overlying water; thus, a reduced bioturbation activity will depress the concentration in the overlying water.
Table 2 Exposure concentrations of 65Cu (i.e., all forms) in sediment and overlying water in T. tubifex exposures, presented as mean ± sd. Significant differences between the means of the 65CuCl2- and 65CuO NP-exposure are indicated by an asterisk (*) (t-test, p < 0.05)
Sample |
Sampling time |
n
|
Control |
65CuCl2 |
65CuO NP |
Unit |
Newly accumulated 65Cu |
Sediment |
7 d |
7 |
<0.01 ± 0.01 |
94.68 ± 27.26 |
88.24 ± 14.68 |
μg g−1 dw |
Water |
7 d |
3 |
0.00 ± 0.00 |
5.44 ± 1.28 |
*10.56 ± 2.48 |
μg L−1 |
Background Cu |
Sediment |
7 d |
7 |
29.35 ± 3.66 |
|
|
μg g−1 dw |
Water |
7 d |
7 |
2.18 ± 2.50 |
|
|
μg L−1 |
Body burden of 65Cu in T. tubifex.
The mean weight-specific body burden (WSBB) of 65Cu in T. tubifex was higher after exposure to 65CuO NP than after exposure to 65CuCl2 (1.1 ± 1.0 vs. 0.6 ± 0.4 μg g−1 dw tissue, respectively; n = 14), though not statistically significant (Fig. 2). The amount of newly accumulated Cu (measured as 65Cu) was relatively low compared to Cu background concentrations (22.3 ± 9.6 μg Cu per g dw tissue) and would likely have been indistinguishable from background without the use of the tracer, as previously reported by Misra et al. (2012)68 and Lammel and Thit et al. (2019).42 Our findings suggest that 65Cu from both the dissolved and nanoparticulate Cu forms in the sediment was bioavailable and accumulated to a similar extent in the worms. This observation is consistent with several other studies reporting that the difference in WSBB of worms (N. diversicolor, L. variegatus, T. tubifex) exposed to different Cu forms is marginal.9,19,42 It should be noted that worms in the NP treatment were not only exposed to 65CuO NPs but also dissolved 65Cu (i.e., non-particulate 65Cu). The proportion of dissolved (i.e., non-particulate) 65Cu in the 65CuO NP dispersion used to prepare the dispersion spiked into sediment was ∼34%.34 The relative amount of dissolved (i.e., non-particulate) 65Cu in the exposure sediment may even have been higher, since the presence of chelating organic ligands (e.g., humic acids) can increase CuO NP dissolution.42,69,70 Hence, it is likely that accumulation of dissolved 65Cu by worms in 65CuO NP-spiked sediment occurred, partly concealing potential differences between treatments.
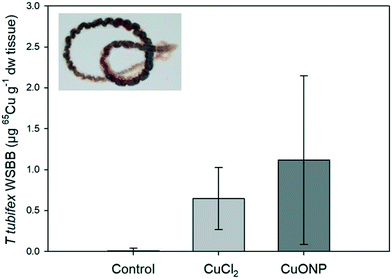 |
| Fig. 2
65Cu WSBB in T. tubifex. Worms were exposed for 7 days in clean sediment or sediment with 65CuCl2 or 65CuO NPs at about 100 μg 65Cu per g dw sediment. Mean ± sd (n = 14). | |
3.2 Transfer of 65Cu and 65CuO NPs from worms to fish
Tissue concentrations of 65Cu in fish.
Due to limited 65Cu concentrations in worms, the dietary exposures doses of 65Cu that fish received were very low (∼4 ± 1 and 7 ± 1 ng g−1 body ww per day in the 65CuCl2- and 65CuO NP-treatment, respectively; for more details see Tables S5 and S6†). Furthermore, in both treatments, the dose of 65Cu represented only a small portion (∼5%) of the total dietary Cu dose fish received (the Cu background concentration was ∼22 μg g−1 dw, which is in the range of nutritional dietary Cu requirements of fish71). At such low exposure doses, low dietary uptake is expected,71,72 and overall 65Cu concentrations in fish tissues were low (Table S6†). Generally, highest concentrations were found in intestine, followed by liver and carcass (Fig. 3). No statistically significant differences in mean 65Cu tissue concentrations were observed between treatments, though they were generally higher in fish exposed to 65CuCl2 than in fish exposed to 65CuO NPs (Table S6† and Fig. 3). However, it must be noted that de facto hepatic 65Cu accumulation was detected in only two of the fish feeding on 65CuCl2-exposed worms, and in only one of the fish feeding on 65CuO NP-exposed worms. Similar to our previous findings,42 Cu background concentrations were highest in liver tissue and Cu accumulation highest in the intestine of fish fed with CuCl2-exposed worms or CuCl2-spiked food packages.
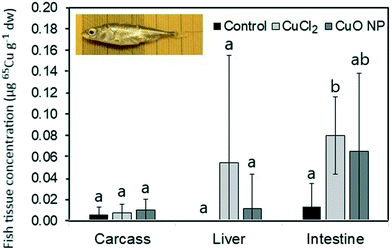 |
| Fig. 3
65Cu accumulation in three-spined stickleback after seven days of dietary exposure to T. tubifex exposed in non-contaminated sediment (control) or sediment spiked with 65CuCl2 or 65CuO NPs for seven days. Bars represent the mean tissue concentrations of 65Cu ± sd (n = 8). Different letter combinations indicate statistically significant differences (p < 0.05). | |
Egestion of 65Cu in feces.
The mean 65Cu concentration in the feces of fish fed 65CuCl2-exposed worms was about half of that measured in feces of fish fed 65CuO NP-exposed worms 65CuCl2 and 65CuO NP exposure, respectively (see Table S6† and Fig. 4). This result is largely consistent with the differences in the received dietary exposure dose (i.e., 65Cu WSBB of 65CuCl2- and 65CuO NP-exposed worms) and the differences in gastrointestinal 65Cu assimilation between the two treatments (see Table S6† and Fig. 3). Altogether, 65Cu concentrations in fish tissues and feces suggest that 65Cu assimilation from 65CuO NP-exposed worms might be lower compared to that from 65CuCl2-exposed worms, albeit not significantly.
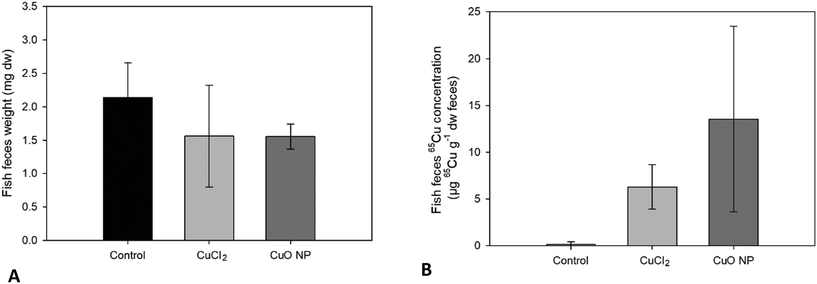 |
| Fig. 4 Fish feces collected during seven days of dietary exposure to sediment exposed T. tubifex. Weight (A) and 65Cu concentration (B) in fish feces produced by individual fish during seven days of exposure and collected daily. Data are presented as mean ± sd, n = 8. | |
3.3 Effects of dietary 65Cu and 65CuO NP exposure in fish
In accordance with the low 65Cu tissue concentrations, limited alterations in the expression of investigated target genes were observed (Fig. 5). Only in intestinal tissue of fish feeding on 65CuCl2-exposed worms, a statistically significant upregulation of one of the assessed target genes, namely gpx, was evident (compared to control fish and fish feeding on 65CuO NP exposed worms) (Fig. 5A). Furthermore, gpx expression levels were found to be positively correlated with intestinal 65Cu concentrations (r = 0.9, p = 0.002), showing that the effect was related to Cu exposure (Table S7†). These results suggest that even minimally elevated dietary Cu levels can induce a disturbance in the cellular redox balance in intestinal tissue. This is in general agreement with previous research reporting Cu-induced oxidative stress and the induction of antioxidant defense mechanisms in intestinal cells and tissues.73,74 Similarly, gpx appeared to be upregulated (though not significantly) in our previous study where three-spined sticklebacks were exposed to a practical diet prepared from CuCl2-spiked worm homogenate (though at higher exposure dose).42
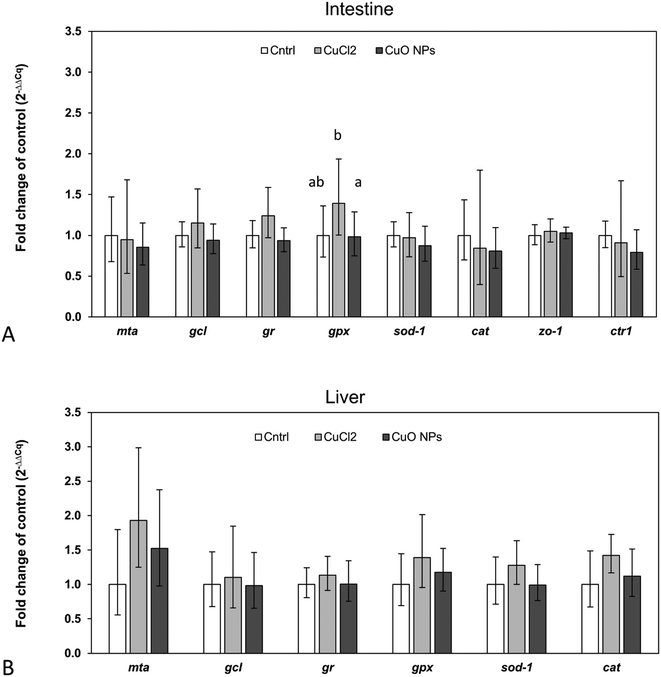 |
| Fig. 5 Relative expression levels of selected target genes in intestinal (A) and hepatic tissue (B) of three-spined stickleback fed T. tubifex exposed to control, 65CuCl2- or 65CuO NP-spiked sediment (white, grey and dark grey bars, respectively). Bars and error bars represent the mean and sd, respectively. Letters above bars indicate statistically significant differences between the means. Bars that do not share the same letter are different at a significance level of 0.05 (One Way ANOVA followed by all pairwise multiple comparison using Holm–Sidak method). | |
It must be noted that in intestine of fish fed with 65CuO NP-exposed worms, gpx mRNA levels were not upregulated (i.e., not different from the control), although mean 65Cu tissue concentrations were similar to those in fish fed with 65CuCl2-exposed worms. This may point to differences in the subcellular distribution19 and or form of 65Cu inside intestinal cells between the exposure groups: However, this hypothesis needs further investigation.
Trophic transfer studies using live worms as feed for fish
Often, dietary bioaccumulation and toxicity of Cu in fish are studied using practical diets, which allows incorporation of Cu in excess (dietary exposure doses in previous studies range from ∼0.4 μg to ∼185 μg Cu per g fish body ww per day71). This may lead to unrealistic exposure scenarios in regards to both food type, complexation with biological tissue, and exposure dose. In contrast, the use of live feed offers the possibility of environmentally realistic exposure in laboratory tests. However, there are two major challenges associated with the use of live feed in trophic transfer studies. The first challenge is to achieve similar WSBB of test compounds and reference compounds in prey organisms (and reproducible WSBBs between individuals and replicates). Here, we found high variation in 65Cu WSBB of sediment-exposed T. tubifex within treatments. However, the mean 65Cu fish exposure doses were not significantly different between the two Cu treatments.
The second challenge is to obtain a high enough WSBB of the test compound in the prey organism for it to be detectable in the predator organism. Here, we found limited 65Cu accumulation from sediment by T. tubifex worms, which translated into a very low dietary exposure dose for fish. Thus, demonstrating trophic transfer was only possible with the use of a stable isotope tracer. If such tracers are unavailable in future studies, higher dietary exposure doses may potentially (depending on when steady-state body burdens are reached) be achieved by extending the time of worm exposure and subsequent fish exposure, rather than increasing exposure concentrations in sediment to unrealistic levels. Yet, it needs to be taken into consideration that this strategy will increase the experimental complexity giving rise to logistical challenges such as the requirement for more spiked sediment and more time-consuming, labor-intensive exposures of worms and fish that need to be run in parallel.
Conclusion
Isotopically labeled NPs offer unique possibilities for studying NP bioaccumulation and trophic transfer under environmentally realistic conditions. The findings from this study show that exposure to Cu at environmentally relevant concentrations in sediment, either in dissolved or nanoparticulate form, leads to increased concentrations in T. tubifex and can be transferred to fish (three-spined stickleback). Increases in body burdens via dietary exposure and trophic transfer of dissolved Cu and CuO NPs appears to be largely similar, which may be in part explained by partial dissolution of the NPs. In quantitative terms, trophic transfer of dissolved/nanoparticulate CuO across two trophic levels seems to be negligible. Based on our data, an increased risk for biomagnification of CuO NPs within the aquatic food web compared to dissolved Cu seems unlikely, which is well in accordance with the published literature. However, considering the complexity and diversity of abiotic and biotic interactions in the environment, more research is required before general conclusions can be drawn with regard to their bioaccumulation and trophic transfer potential, as well as overall environmental risk.
Conflicts of interest
There are no conflicts to declare.
Acknowledgements
This research was financed by the Villum Foundation (grant reference number: 00010592). The authors acknowledge support from the Facility for Environmental Nanoscience Analysis and Characterisation (FENAC). Furthermore, we thank Astrid Mikaelsson, Britt Wassmur for technical assistance, and Mette Flodgaard for her substantial support in the laboratory.
References
- A. A. Keller, S. McFerran, A. Lazareva and S. Suh, Global life cycle releases of engineered nanomaterials, J. Nanopart. Res., 2013, 15, 1692 CrossRef.
- A. Caballero-Guzman and B. Nowack, Prospective nanomaterial mass flows to the environment by life cycle stage from five applications containing CuO, DPP, FeOx, CNT and SiO2, J. Cleaner Prod., 2018, 203, 990–1002 CrossRef CAS.
- A. A. Keller, A. S. Adeleye, J. R. Conway, K. L. Garner, L. Zhao, G. N. Cherr, J. Hong, J. L. Gardea-Torresdey, H. A. Godwin, S. Hanna, Z. Ji, C. Kaweeteerawat, S. Lin, H. S. Lenihan, R. J. Miller, A. E. Nel, J. R. Peralta-Videa, S. L. Walker, A. A. Taylor, C. Torres-Duarte, J. I. Zink and N. Zuverza-Mena, Comparative environmental fate and toxicity of copper nanomaterials, NanoImpact, 2017, 7, 28–40 CrossRef.
- K. L. Garner, S. Suh and A. A. Keller, Assessing the Risk of Engineered Nanomaterials in the Environment: Development and Application of the nanoFate Model, Environ. Sci. Technol., 2017, 51, 5541–5551 CrossRef CAS PubMed.
- A. M. Dietrich and D. L. Gallagher, Fate and Environmental Impact of Pesticides in Plastic Mulch Production Runoff: Field and Laboratory Studies, J. Agric. Food Chem., 2002, 50, 4409–4416 CrossRef CAS PubMed.
- P. Perez-Rodriguez, D. Soto-Gomez, I. De La Calle, J. E. Lopez-Periago and M. Paradelo, Rainfall-induced removal of copper-based spray residues from vines, Ecotoxicol. Environ. Saf., 2016, 132, 304–310 CrossRef CAS PubMed.
- A. S. Adeleye, E. A. Oranu, M. Y. Tao and A. A. Keller, Release and detection of nanosized copper from a commercial antifouling paint, Water Res., 2016, 102, 374–382 CrossRef CAS PubMed.
- A. S. Adeleye, J. R. Conway, T. Perez, P. Rutten and A. A. Keller, Influence of Extracellular Polymeric Substances on the Long-Term Fate, Dissolution, and Speciation of Copper-Based Nanoparticles, Environ. Sci. Technol., 2014, 48, 12561–12568 CrossRef CAS PubMed.
- A. Thit, A. Dybowska, C. Kobler, G. Kennaway and H. Selck, Influence of copper oxide nanoparticle shape on bioaccumulation, cellular internalization and effects in the estuarine sediment-dwelling polychaete, Nereis diversicolor, Mar. Environ. Res., 2015, 111, 89–98 CrossRef CAS PubMed.
- Y. L. Xiao, M. G. Vijver and W. Peijnenburg, Impact of water chemistry on the behavior and fate of copper nanoparticles, Environ. Pollut., 2018, 234, 684–691 CrossRef CAS PubMed.
- L. Z. Miao, C. Wang, J. Hou, P. F. Wang, Y. H. Ao, Y. Li, B. W. Lv, Y. Y. Yang, G. X. You and Y. Xu, Enhanced stability and dissolution of CuO nanoparticles by extracellular polymeric substances in aqueous environment, J. Nanopart. Res., 2015, 17, 12 CrossRef PubMed.
- J. R. Conway, A. S. Adeleye, J. Gardea-Torresdey and A. A. Keller, Aggregation, Dissolution, and Transformation of Copper Nanoparticles in Natural Waters, Environ. Sci. Technol., 2015, 49, 2749–2756 CrossRef CAS PubMed.
- I. A. Mudunkotuwa, J. M. Pettibone and V. H. Grassian, Environmental Implications of Nanoparticle Aging in the Processing and Fate of Copper-Based Nanomaterials, Environ. Sci. Technol., 2012, 46, 7001–7010 CrossRef CAS PubMed.
- Z. Y. Wang, A. von dem Bussche, P. K. Kabadi, A. B. Kane and R. H. Hurt, Biological and Environmental Transformations of Copper-Based Nanomaterials, ACS Nano, 2013, 7, 8715–8727 CrossRef CAS PubMed.
- K. L. Garner and A. A. Keller, Emerging patterns for engineered nanomaterials in the environment: a review of fate and toxicity studies, J. Nanopart. Res., 2014, 16, 2503 CrossRef.
- Z. Liu, C. Wang, J. Hou, P. Wang, L. Miao, B. Lv, Y. Yang, G. You, Y. Xu, M. Zhang and H. Ci, Aggregation, sedimentation, and dissolution of CuO and ZnO nanoparticles in five waters, Environ. Sci. Pollut. Res., 2018, 25, 31240–31249 CrossRef CAS PubMed.
- B. E. Vencalek, S. N. Laughton, E. Spielman-Sun, S. M. Rodrigues, J. M. Unrine, G. V. Lowry and K. B. Gregory, In Situ Measurement of CuO and Cu(OH)(2) Nanoparticle Dissolution Rates in Quiescent Freshwater Mesocosms, Environ. Sci. Technol. Lett., 2016, 3, 375–380 CrossRef CAS.
- A. Thit, G. T. Banta and H. Selck, Bioaccumulation, subcellular distribution and toxicity of sediment-associated copper in the ragworm Nereis diversicolor: The relative importance of aqueous copper, copper oxide nanoparticles and microparticles, Environ. Pollut., 2015, 202, 50–57 CrossRef CAS PubMed.
- A. Thit, T. Ramskov, M. N. Croteau and H. Selck, Biodynamics of copper oxide nanoparticles and copper ions in an oligochaete - Part II: Subcellular distribution following sediment exposure, Aquat. Toxicol., 2016, 180, 25–35 CrossRef CAS PubMed.
- S. R. Tangaa, H. Selck, M. Winther-Nielsen and F. R. Khan, Trophic transfer of metal-based nanoparticles in aquatic environments: a review and recommendations for future research focus, Environ. Sci.: Nano, 2016, 3, 966–981 RSC.
- A. Bour, F. Mouchet, J. Silvestre, L. Gauthier and E. Pinelli, Environmentally relevant approaches to assess nanoparticles ecotoxicity: a review, J. Hazard. Mater., 2015, 283, 764–777 CrossRef CAS PubMed.
- M. Ates, Z. Arslan, V. Demir, J. Daniels and I. O. Farah, Accumulation and toxicity of CuO and ZnO nanoparticles through waterborne and dietary exposure of goldfish (Carassius auratus), Environ. Toxicol., 2015, 30, 119–128 CrossRef CAS PubMed.
- Y. Chae and Y. J. An, Toxicity and transfer of polyvinylpyrrolidone-coated silver nanowires in an aquatic food chain consisting of algae, water fleas, and zebrafish, Aquat. Toxicol., 2016, 173, 94–104 CrossRef CAS PubMed.
- S. Dong, T. Xia, Y. Yang, S. Lin and L. Mao, Bioaccumulation of (14)C-Labeled Graphene in an Aquatic Food Chain through Direct Uptake or Trophic Transfer, Environ. Sci. Technol., 2018, 52, 541–549 CrossRef CAS PubMed.
- J. M. Lacave, A. Fanjul, E. Bilbao, N. Gutierrez, I. Barrio, I. Arostegui, M. P. Cajaraville and A. Orbea, Acute toxicity, bioaccumulation and effects of dietary transfer of silver from brine shrimp exposed to PVP/PEI-coated silver nanoparticles to zebrafish, Comp. Biochem. Physiol. C Toxicol. Pharmacol., 2017, 199, 69–80 CAS.
- W. M. Lee and Y. J. An, Evidence of three-level trophic transfer of quantum dots in an aquatic food chain by using bioimaging, Nanotoxicology, 2015, 9, 407–412 CrossRef CAS PubMed.
- N. A. Lewinski, H. Zhu, C. R. Ouyang, G. P. Conner, D. S. Wagner, V. L. Colvin and R. A. Drezek, Trophic transfer of amphiphilic polymer coated CdSe/ZnS quantum dots to Danio rerio, Nanoscale, 2011, 3, 3080–3083 RSC.
- L. M. Skjolding, M. Winther-Nielsen and A. Baun, Trophic transfer of differently functionalized zinc oxide nanoparticles from crustaceans (Daphnia magna) to zebrafish (Danio rerio), Aquat. Toxicol., 2014, 157, 101–108 CrossRef CAS PubMed.
- J. Wang and W. X. Wang, Low bioavailability of silver nanoparticles presents trophic toxicity to marine medaka (Oryzias melastigma), Environ. Sci. Technol., 2014, 48, 8152–8161 CrossRef CAS PubMed.
- Z. Wang, L. Yin, J. Zhao and B. Xing, Trophic transfer and accumulation of TiO2 nanoparticles from clamworm (Perinereis aibuhitensis) to juvenile turbot (Scophthalmus maximus) along a marine benthic food chain, Water Res., 2016, 95, 250–259 CrossRef CAS PubMed.
- X. Zhu, J. Wang, X. Zhang, Y. Chang and Y. Chen, Trophic transfer of TiO(2) nanoparticles from Daphnia to zebrafish in a simplified freshwater food chain, Chemosphere, 2010, 79, 928–933 CrossRef CAS PubMed.
- C. C. Piccinetti, C. Montis, M. Bonini, R. Laura, M. C. Guerrera, G. Radaelli, F. Vianello, V. Santinelli, F. Maradonna, V. Nozzi, A. Miccoli and I. Olivotto, Transfer of Silica-Coated Magnetic (Fe3O4) Nanoparticles Through Food: A Molecular and Morphological Study in Zebrafish, Zebrafish, 2014, 11, 567–579 CrossRef CAS PubMed.
- T. Nemati, M. Sarkheil and S. A. Johari, Trophic transfer of CuO nanoparticles from brine shrimp (Artemia salina) nauplii to convict cichlid (Amatitlania nigrofasciata) larvae: uptake, accumulation and elimination, Environ. Sci. Pollut. Res., 2019, 26, 9610–9618 CrossRef CAS PubMed.
- T. Lammel, A. Thit, X. Cuic, C. Mouneyrac, A. Baun, E. Valsami-Jones, J. Sturve and H. Selck, A study of the dietary uptake and effects of nanoparticulate and dissolved copper in fish (three-spined stickleback) at environmentally relevant exposure doses utilizing stable isotope-labeled 65CuO NPs and 65CuCl2,
, 2020 Search PubMed , submitted manuscript under review.
- S. A. Johari, M. Sarkheil, S. Asghari, F. Haghighat, L. Dekani and S. Keyvanshokooh, Comparative toxicity of nanoparticulate and ionic copper following dietary exposure to common carp (Cyprinus carpio), Comp. Biochem. Physiol., Part C: Toxicol. Pharmacol., 2020, 229, 10 Search PubMed.
- D. Boyle, N. J. Clark, T. L. Botha and R. D. Handy, Comparison of the dietary bioavailability of copper sulphate and copper oxide nanomaterials in ex vivo gut sacs of rainbow trout: effects of low pH and amino acids in the lumen, Environ. Sci.: Nano, 2020 10.1039/D0EN00095G.
- C. S. Ramsden, T. J. Smith, B. J. Shaw and R. D. Handy, Dietary exposure to titanium dioxide nanoparticles in rainbow trout, (Oncorhynchus mykiss): no effect on growth, but subtle biochemical disturbances in the brain, Ecotoxicology, 2009, 18, 939–951 CrossRef CAS PubMed.
- L. Chupani, H. Niksirat, J. Velisek, A. Stara, S. Hradilova, J. Kolarik, A. Panacek and E. Zuskova, Chronic dietary toxicity of zinc oxide nanoparticles in common carp (Cyprinus carpio L.): Tissue accumulation and physiological responses, Ecotoxicol. Environ. Saf., 2018, 147, 110–116 CrossRef CAS PubMed.
- M. Connolly, M. Fernandez, E. Conde, F. Torrent, J. M. Navas and M. L. Fernandez-Cruz, Tissue distribution of zinc and subtle oxidative stress effects after dietary administration of ZnO nanoparticles to rainbow trout, Sci. Total Environ., 2016, 551, 334–343 CrossRef PubMed.
- B. Geffroy, C. Ladhar, S. Cambier, M. Treguer-Delapierre, D. Brethes and J. P. Bourdineaud, Impact of dietary gold nanoparticles in zebrafish at very low contamination pressure: The role of size, concentration and exposure time, Nanotoxicology, 2012, 6, 144–160 CrossRef CAS PubMed.
- C. Ladhar, B. Geffroy, S. Cambier, M. Treguer-Delapierre, E. Durand, D. Brethes and J. P. Bourdineaud, Impact of dietary cadmium sulphide nanoparticles on Danio rerio zebrafish at very low contamination pressure, Nanotoxicology, 2014, 8, 676–685 CrossRef CAS PubMed.
- T. Lammel, A. Thit, C. Mouneyrac, A. Baun, J. Sturve and H. Selck, Trophic transfer of CuO NPs and dissolved Cu from sediment to worms to fish – a proof-of-concept study, Environ. Sci.: Nano, 2019, 6, 1140–1155 RSC.
- M. F. El Basuini, A. M. El-Hais, M. A. O. Dawood, A. E. Abou-Zeid, S. Z. El-Damrawy, M. M. E. Khalafalla, S. Koshio, M. Ishikawa and S. Dossou, Effect of different levels of dietary copper nanoparticles and copper sulfate on growth performance, blood biochemical profiles, antioxidant status and immune response of red sea bream (Pagrus major), Aquaculture, 2016, 455, 32–40 CrossRef CAS.
- H. Wang, H. Zhu, X. Wang, E. Li, Z. Du, J. Qin and L. Chen, Comparison of copper bioavailability in copper-methionine, nano-copper oxide and copper sulfate additives in the diet of Russian sturgeon Acipenser gueldenstaedtii, Aquaculture, 2018, 482, 146–154 CrossRef CAS.
- S. Lindh, P. Razmara, S. Bogart and G. Pyle, Comparative tissue distribution and depuration characteristics of copper nanoparticles and soluble copper in rainbow trout (Oncorhynchus mykiss), Environ. Toxicol. Chem., 2019, 38, 80–89 CrossRef CAS PubMed.
-
OECD, Test No. 315: Bioaccumulation in Sediment-dwelling Benthic Oligochaetes, 2008 Search PubMed.
- D. Milani, T. B. Reynoldson, U. Borgmann and J. Kolasa, The relative sensitivity of four benthic invertebrates to metals in spiked-sediment exposures and application to contaminated field sediment, Environ. Toxicol. Chem., 2003, 22, 845–854 CrossRef CAS PubMed.
- Y. E. Roman, K. A. C. De Schamphelaere, L. T. H. Nguyen and C. R. Janssen, Chronic toxicity of copper to five benthic invertebrates in laboratory-formulated sediment: Sensitivity comparison and preliminary risk assessment, Sci. Total Environ., 2007, 387, 128–140 CrossRef CAS PubMed.
- M.-L. Lucan-Bouché, S. Biagianti-Risbourg, F. Arsac and G. Vernet, An original decontamination process developed by the aquatic oligochaete Tubifex tubifex exposed to copper and lead, Aquat. Toxicol., 1999, 45, 9–17 CrossRef.
- Y. E. Roman, K. A. C. De Schamphelaere, L. T. H. Nguyen and C. R. Janssen, Chronic toxicity of copper to five benthic invertebrates in laboratory-formulated sediment: Sensitivity comparison and preliminary risk assessment, Sci. Total Environ., 2007, 387, 128–140 CrossRef CAS PubMed.
- M. Vecchi, T. B. Reynoldson, A. Pasteris and G. Bonomi, Toxicity of copper-spiked sediments to Tubifex tubifex (Oligochaeta, Tubificidae): Comparison of the 28-day reproductive bioassay with an early-life-stage bioassay, Environ. Toxicol. Chem., 1999, 18, 1173–1179 CAS.
-
R. G. Wotton, A Functional Biology of Sticklebacks, Springer, Boston, MA, 1984 Search PubMed.
- H. B. N. Hynes, The food of freshwater sticklebacks (gasterosteus-aculeatus and pygosteus-pungitius), with a review of methods used in studies of the food of fishes, J. Anim. Ecol., 1950, 19, 36–58 CrossRef.
-
I. Katsiadaki, in Biology of the Three-Spined Stickleback, ed. S. Ostlund-Nilsson, I. Mayer and F. A. Huntingford, CRC Press, Boca Raton, 2006, p. 408 Search PubMed.
- N. S. Hogan, C. A. Wartman, M. A. Finley, J. G. van der Lee and M. R. van den Heuvel, Simultaneous determination of androgenic and estrogenic endpoints in the threespine stickleback (Gasterosteus aculeatus) using quantitative RT-PCR, Aquat. Toxicol., 2008, 90, 269–276 CrossRef CAS PubMed.
- S. Hibbeler, J. P. Scharsack and S. Becker, Housekeeping genes for quantitative expression studies in the three-spined stickleback Gasterosteus aculeatus, BMC Mol. Biol., 2008, 9, 18 CrossRef PubMed.
- E. M. Santos, J. S. Ball, T. D. Williams, H. F. Wu, F. Ortega, R. Van Aerle, I. Katsiadaki, F. Falciani, M. R. Viant, J. K. Chipman and C. R. Tyler, Identifying Health Impacts of Exposure to Copper Using Transcriptomics and Metabolomics in a Fish Model, Environ. Sci. Technol., 2010, 44, 820–826 CrossRef CAS PubMed.
- P. Lubiana, J. M. Prokkola, M. Nikinmaa, T. Burmester, M. Kanerva and M. Gotting, The effects of the painkiller diclofenac and hypoxia on gene transcription and antioxidant system in the gills of three-spined stickleback, Comp. Biochem. Physiol., Part C: Toxicol. Pharmacol., 2016, 185, 147–154 Search PubMed.
- M.-N. Croteau, S. N. Luoma, B. R. Topping and C. B. Lopez, Stable Metal Isotopes Reveal Copper Accumulation and Loss Dynamics in the Freshwater Bivalve Corbicula, Environ. Sci. Technol., 2004, 38, 5002–5009 CrossRef CAS PubMed.
- T. B. Coplen, J. K. Bohlke, P. De Bievre, T. Ding, N. E. Holden, J. A. Hopple, H. R. Krouse, A. Lamberty, H. S. Peiser, K. Revesz, S. E. Rieder, K. J. R. Rosman, E. Roth, P. D. P. Taylor, R. D. Vocke and Y. K. Xiao, Isotope-abundance variations of selected elements - (IUPAC Technical Report), Pure Appl. Chem., 2002, 74, 1987–2017 CAS.
- B. Berthet, C. Mouneyrac, J. C. Amiard, C. Amiard-Triquet, Y. Berthelot, A. Le Hen, O. Mastain, P. S. Rainbow and B. D. Smith, Accumulation and soluble binding of cadmium, copper, and zinc in the polychaete Hediste diversicolor from coastal sites with different trace metal Bioavailabilities, Arch. Environ. Contam. Toxicol., 2003, 45, 468–478 CrossRef CAS PubMed.
- A. Ciutat, O. Weber, M. Gerino and A. Boudou, Stratigraphic effects of tubificids in freshwater sediments: a kinetic study based on X-ray images and grain-size analysis, Acta. Oecol., 2006, 30, 228–237 CrossRef.
- S. Lagauzere, M. Motelica-Heino, E. Viollier, G. Stora and J. M. Bonzom, Remobilisation of uranium from contaminated freshwater sediments by bioturbation, Biogeosciences, 2014, 11, 3381–3396 CrossRef CAS.
- Y. He, B. Men, X. Yang, Y. Li, H. Xu and D. Wang, Investigation of heavy metals release from sediment with bioturbation/bioirrigation, Chemosphere, 2017, 184, 235–243 CrossRef CAS PubMed.
- M. L. Bouche, F. Arnoult and G. Vernet, Caudal regeneration in Tubifex tubifex (Oligochaeta, Tubificidae) following copper exposure, Invertebr. Biol., 2003, 122, 42–51 CrossRef.
- E. R. Hunting, C. Mulder, M. H. S. Kraak, A. M. Breure and W. Admiraal, Effects of copper on invertebrate–sediment interactions, Environ. Pollut., 2013, 180, 131–135 CrossRef CAS PubMed.
- A. Gerhardt, Screening the Toxicity of Ni, Cd, Cu, Ivermectin, and Imidacloprid in a Short-Term Automated Behavioral Toxicity Test with Tubifex tubifex (Müller 1774) (Oligochaeta), Hum. Ecol. Risk Assess., 2009, 15, 27–40 CrossRef CAS.
- S. K. Misra, A. Dybowska, D. Berhanu, M. N. Croteau, S. N. Luoma, A. R. Boccaccini and E. Valsami-Jones, Isotopically Modified Nanoparticles for Enhanced Detection in Bioaccumulation Studies, Environ. Sci. Technol., 2012, 46, 1216–1222 CrossRef CAS PubMed.
- C. Peng, C. Shen and S. Zheng,
et al., Transformation of CuO Nanoparticles in the Aquatic Environment: Influence of pH, Electrolytes and Natural Organic Matter, Nanomaterials, 2017, 7(10), 326 CrossRef PubMed.
- R. D. Kent and P. J. Vikesland, Dissolution and Persistence of Copper-Based Nanomaterials in Undersaturated Solutions with Respect to Cupric Solid Phases, Environ. Sci. Technol., 2016, 50, 6772–6781 CrossRef CAS PubMed.
- S. J. Clearwater, A. M. Farag and J. S. Meyer, Bioavailability and toxicity of dietborne copper and zinc to fish, Comp. Biochem. Physiol., Part C: Toxicol. Pharmacol., 2002, 132, 269–313 CrossRef.
-
M. Grosell, in Fish Physiology, ed. C. M. Wood, A. P. Farrell and C. J. Brauner, Academic Press, 2011, vol. 31, pp. 53–133 Search PubMed.
- W.-D. Jiang, P. Wu, S.-Y. Kuang, Y. Liu, J. Jiang, K. Hu, S.-H. Li, L. Tang, L. Feng and X.-Q. Zhou, Myo-inositol prevents copper-induced oxidative damage and changes in antioxidant capacity in various organs and the enterocytes of juvenile Jian carp (Cyprinus carpio var. Jian), Aquat. Toxicol., 2011, 105, 543–551 CrossRef CAS PubMed.
- W.-D. Jiang, Y. Liu, J. Jiang, K. Hu, S.-H. Li, L. Feng and X.-Q. Zhou, In vitro interceptive and reparative effects of myo-inositol against copper-induced oxidative damage and antioxidant system disturbance in primary cultured fish enterocytes, Aquat. Toxicol., 2013, 132–133, 100–110 CrossRef CAS PubMed.
Footnotes |
† Electronic supplementary information (ESI) available. See DOI: 10.1039/d0en00227e |
‡ Shared first authorship. |
|
This journal is © The Royal Society of Chemistry 2020 |
Click here to see how this site uses Cookies. View our privacy policy here.