Controlled protein mediated aggregation of polystyrene nanoplastics does not reduce toxicity towards Daphnia magna†
Received
30th October 2019
, Accepted 1st April 2020
First published on 6th April 2020
Abstract
Microplastics have recently become a growing environmental issue, whereas the smaller fractions, nanoplastics, have received less attention. Due to their small size, nanoplastics may affect organisms differently and potentially more severely than larger microplastics. In natural environments nanoplastics also interact with organic material and form larger aggregates, which may, potentially, reduce their toxicity as they grow in size. We tested the change in toxicity towards Daphnia magna by controlling the size of the aggregates of positively charged 50 nm polystyrene nanoplastics, which are highly toxic as single particles. We show that although 200 to 500 nm nanoplastics are not toxic, aggregates of 50 nm nanoplastics in the same size range are at least as toxic as the free, 50 nm, nanoplastics. Hence, an increase in size through aggregation, a process likely to occur as nanoparticles enter natural ecosystems, does not reduce toxicity. In a broader context this finding provides a firm basis for societal decision making regarding the potency of nanoparticles as they enter natural ecosystems.
Environmental significance
Nanomaterial including nanoplastics that reach nature will interact with organic materials forming heteroaggregates. How well size dependent biological effects, for example toxicity, are retained after aggregation is not well known. We show that aggregates of small toxic nanoparticles retain their size dependent toxicity although particles of the same material and same size as the aggregates are non-toxic. The result has general impact on how we understand nanoparticle toxicity in nature as aggregation does not automatically lower the nanoparticle size dependent toxicity. Furthermore, the results suggest that surface curvature rather than small size itself carries the size dependent toxicity.
|
Introduction
The use of polymeric plastic products has increased enormously since the 1960s: the global production exceeded 348 million metric tonnes in 2017,1 and is expected to continue to increase. Plastic materials are immensely beneficial to society in our everyday life and likely also for future technical advancements.2 However, a considerable part of the produced plastics will find its way into natural ecosystems and recent estimates show that between 1.15 and 2.41 million metric tonnes of plastics are yearly released into lakes and waterways.3 Physical problems for wildlife from ingestion and entanglement are well documented and often reported in media. Smaller pieces of plastics, microplastics (<5 mm), are not as visible to the naked eye, but their widespread presence are known since long.4 These plastics can be roughly divided into two groups, 1) primary microplastics that are intentionally manufactured in this small form and that can then be accidentally released into nature or 2) secondary microplastics that are products from biotic or abiotic degradation of larger pieces of plastics.5,6 Microplastics in the nanoscale (1–100 nm) are yet to be confirmed in nature.7 Currently we only know of one study suggesting the presence of nanoplastics in the Atlantic Ocean8 and intense work is being performed to find reliable methods for nanoparticle detection.9 Another environmental indication of the continuous break-down of plastics in nature is the presence of styrene oligomers in the marine environment.10,11 Furthermore, it has been shown experimentally that plastics can be mechanically,12 and photo-chemically13,14 broken down to nanoplastics. Interestingly, nanoplastics released from biodegradable plastic, such as polyhydroxybutyrate, appear to have adverse effects on freshwater organisms.15
Nanoplastics can, due to their small size, potentially demonstrate different risks than larger pieces of plastics16,17 and their fate in nature may be different.18 The size of nanoplastics are of crucial importance for their biological effect on aquatic organisms. Uptake and bioaccumulation of polystyrene nanoplastics have been shown to be size,dependent in several aquatic species,19–25 and they can be transferred up along aquatic food chains.26–29 The toxicity of polystyrene nanoplastics are strongly size-dependent and nanoplastics between around 20 to 50 nm are often found to be more toxic than larger sizes.22,29–32
When entering a natural environment, the nanoparticles are interacting with the surrounding biomolecules forming large heteroaggregates,33–36 which may affect the toxicity of the nanoparticle.33,34,36 The interactions change the presented surface chemistry as the particles are covered with various biomolecules. This is interesting as positively charged model polystyrene nanoparticles are reported to be more toxic than negatively charged ones,29,34,36 although the effect of the interactions differ. Another outcome from the interactions with biomolecules is that nanoparticles will be part of larger aggregates,34–36 which possibly can diminish the nano-size properties. However, little is known on how aggregation of nanoplastics changes size dependent effects.
Therefore, the aim of our study was to examine how size-controlled protein driven aggregation of 50 nm polystyrene particles affects the toxicity towards Daphnia magna (D. magna) compared to the toxicity of free polystyrene particles of the sizes 50, 200 and 500 nm (Fig. 1). As it has previously been shown that 50 nm, but not 200 nm, amine modified particles are toxic to D. magna,29 we hypothesized that aggregates of 50 nm plastic particles would be less reactive (toxic) to aquatic organisms than non-aggregated 50 nm particles.
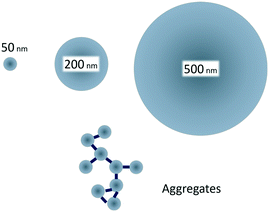 |
| Fig. 1 Small, 50 nm, amine modified polystyrene nanoparticles are toxic to D. magna, whereas larger, 200 and 500 nm, are not.29 By creating protein/particle aggregates in the same size range as the larger particles the effect of aggregation on toxicity can be studied. | |
Results and discussion
Protein PS-NH2 NPs aggregates
Reproducible aggregation with defined aggregate size was achieved using human immunoglobulin G (IgG), known to form concentration dependent aggregates with polystyrene nanoparticles,37 as a non-covalent linker between the 50 nm amine modified polystyrene nanoparticles PS-NH2 NPs. Several different conditions, e.g. substrate concentrations (Fig. S1 and S2†), pH (Fig. S3†), centrifugation speed and time influence the aggregation. After aggregation the aggregates were carefully pelleted. Dynamic light scattering (DLS) of the supernatant showed particle sizes of around 60 nm (Table 1), which indicates that the free 50 nm nanoparticles remain dispersed. The size distribution of the aggregate from the resuspended pellet was characterized by DLS (Table 1), differential centrifugal sedimentation (DCS), and nanoparticle tracking analysis (NTA) (Fig. 2). DCS showed a broad absorbance size distribution with a median peak size of 650 nm (Fig. 2a). NTA showed a variety of peaks, between 100–700 nm (Fig. 2b). The area under the curves represents the total number of aggregates at a specific size. The aggregate size of two particles or more must be above 100 nm. Therefore, for each of the data sets, the percent aggregates smaller or larger than 100 nm was calculated. This showed that, according to the DCS, 99.6% of the sample was ≥100 nm, compared to the NTA showing 85.4% of the sample ≥100 nm (Fig. 2c). The NTA data show a somewhat smaller size than DCS. Overall the data confirm that the PS-NH2 NPs together with IgG had formed large PS-NH2-IgG aggregates at a size range of 100 to 600 nm. It is also noteworthy that the NTA sample was diluted 1
:
200 before measurements, indicating that the PS-NH2-IgG aggregates are stable after dilution.
Table 1 The DLS data obtained for the supernatant and resuspended pellet after created aggregates (1.0 mg mL−1 IgG, 10 mg mL−1 NP, in 10 mM sodium phosphate buffer, pH 7.5, 20 mM NaCl) after centrifugation at 3000 rpm, at 16 °C, for 20 min. The mean values of eight samples were calculated together with standard deviation (SD)
Sample |
DLS |
D (nm) ± SD |
% Pda |
Percent polydispersity.
|
50 nm PS-NH2 |
52 ± 0.4 |
9.2 |
Supernatant |
58 ± 3 |
22 |
PS-NH2-IgG |
239 ± 98 |
Multimodal |
200 nm PS-NH2 |
189 ± 2 |
6.8 |
500 nm PS-NH2 |
493 ± 19 |
7.0 |
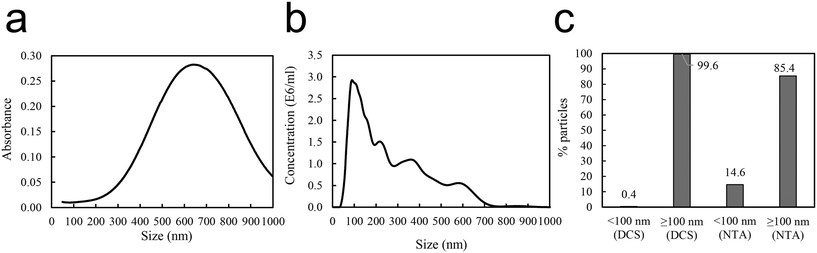 |
| Fig. 2 (a) DCS and (b) NTA data obtained for the created aggregates (in 10 mM sodium phosphate buffer, pH 7.5, 20 mM NaCl) with 10 mg mL−1 NP and 1.0 mg mL−1 IgG. (c) The percentage of particles of sizes either <100 or ≥100 nm, according to the DCS and NTA measurements. | |
Acute Daphnia magna toxicity test
A schematic overview shows the different samples tested in acute toxicity test on D. magna (Fig. 3). In the control samples – H2O, buffer, or IgG alone – as well as in 200 or 500 nm sized PS-NH2 NPs we recorded no mortality in D. magna during the 48 h exposure. However, there was a lethal effect of both the 50 nm particles and the PS-NH2-IgG aggregates (χ2(4) = 69.6, p < 0.0001; Fig. 4). Moreover, there was also a concentration effect showing faster mortality at 2.7 mg L−1 than 1.4 mg L−1 concentrations (Fig. 4). However, when comparing the lethality of single particles and PS-NH2-IgG aggregates using log-rank test (Mantel–Cox), no significant differences could be detected at neither the higher concentrations (χ2(1) = 0.196, p = 0.66), nor the lower (χ2(1) = 2.93, p = 0.087). At the end of the experiment, the survival rate in the control group was 100% and in the low concentration, 1.4 mg mL−1, of single particles 20% of the animals were still alive.
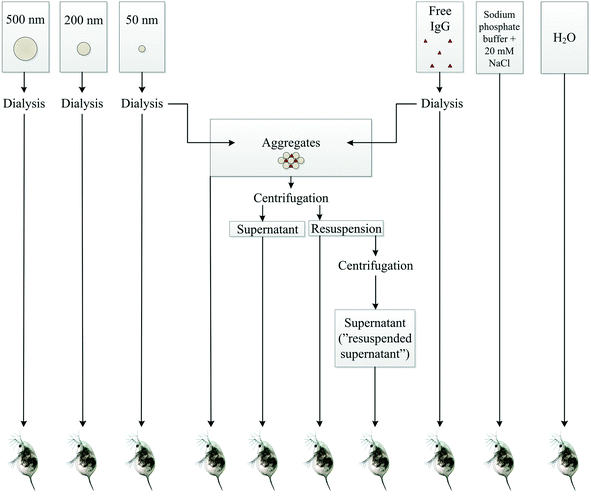 |
| Fig. 3 A schematic overview of the different samples tested on D. magna individuals. The number of replicates is 10 for each treatment. | |
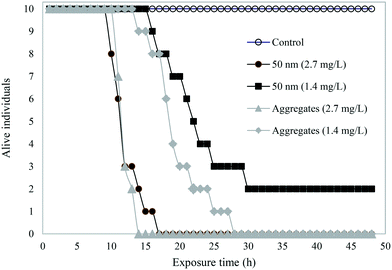 |
| Fig. 4 The mortality of D. magna individuals after 48 h exposure. | |
In a complementary experiment, the PS-NH2-IgG aggregate concentration was the same as above (2.7 mg L−1). However, the mass concentration of the 50 nm particles was adjusted to match the surface area – 400 cm2 L−1 – corresponding to 2.7 mg L−1 PS-NH2-IgG aggregates, (Fig. 5). Under these conditions only the PS-NH2-IgG aggregates showed toxicity. During the process of creating the aggregates the PS-NH2-IgG aggregates were collected by centrifugation. The single particles remained in the supernatant (Table 1). To rule out that there were single particles in the aggregates the dispersed PS-NH2-IgG aggregates were centrifuged again at the same speed and the supernatant collected. No toxicity was detected in this supernatant (Fig. 5).
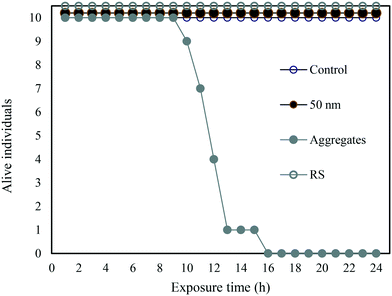 |
| Fig. 5 The mortality of D. magna after exposure to PS-NH2-IgG aggregates, 50 nm PS-NH2, or the supernatant of the resuspension (RS). The particle concentration of the aggregates was 2.7 mg L−1. The surface area of aggregates and 50 nm PS-NH2 was kept constant at 400 cm2 L−1. The RS volume added to the D. magna was chosen to match the volume of aggregates added. The 50 nm and RS data have been shifted upwards in order to show all data points. | |
The PS-NH2-IgG aggregates used in our study were stable when not exposed to organisms. However, the size of the protein aggregates decreased considerably already after 24 h exposure to D. magna feeding activity, leading to a reduced frequency of larger aggregate sizes, as well as to an increase in the concentration of 50 nm nanoparticles (Fig. S4†). In contrast, the size of the 200 and 500 nm PS-NH2 were unaffected. However, it should be noted that measuring the size of nanoparticles at low concentrations in a complex biological environment is not trivial. D. magna themselves may induce particle aggregation during the incubation with the PS-NH2.34,36 Therefore, the PS-NH2 particles were stabilized with albumin before presented to the D. magna. The diameter of the albumin coated particles was about 10 nm longer than uncoated particles, indicating that a monolayer of albumin was formed. The albumin content, according to SDS-PAGE after centrifugation, on the particles was unaffected after 48 h incubation indicating that the particles were stable throughout the experiment (Fig. S5†). There was no difference in the toxicity of the albumin stabilized PS-NH2 and the non-coated particles (Fig. S5†), suggesting that the effect of possible aggregation of the non-coated particles was negligible.
The aims of the study were (I) to investigate the effect on D. magna individuals after exposure to nanoplastic particles aggregates, and (II) if the toxicity in D. magna will decrease when free 50 nm PS-NH2 forms aggregates, which is expected to occur in natural ecosystems.
We here show lethal effects of both the single particles and the PS-NH2-IgG aggregates, showing that aggregation does not decrease the effect of 50 nm PS-NH2. No lethal effect was observed in the control groups or treatments of larger-sized (200 and 500 nm) nanoparticles (Fig. 4) suggesting that the acute toxicity is not related to the plastic material per se, but to the nano-size of the particles. The 50 nm PS-NH2 NPs and the PS-NH2-IgG aggregates composed of 50 nm NPs, showed a similar mortality rate when the two treatments were added to D. magna individuals at the same mass concentration. However, when instead the surface area was kept constant, i.e. the mass concentration of the free 50 nm PS-NH2 NPs was much lower, only the aggregates were toxic (Fig. 5).
One of the explanations for the retained toxicity is that the PS-NH2-IgG aggregates – when entering the D. magna – rapidly break down into toxic free 50 nm PS-NH2, rather than taking on the properties of the particles of the approximately same size, such as 200 or 500 nm. However, as the size, and thereby the surface curvature, is important for the toxicity, another explanation may be that the aggregates which have a surface made up by parts of many 50 nm particles present enough curvature to be toxic to D. magna. In future studies these possibilities could be discriminated from each other by making more chemically stable aggregates that are less likely to be broken down in D. magna. Furthermore, in nature, proteins are only one of several biomolecules that could aggregate with polystyrene particles. Other types of natural organic material may also interact differently with the polystyrene surface and the amine group, creating aggregates with different size, morphology and chemical stability and thereby affect the toxicity in different ways. For example, 53 nm amine modified polystyrene form large micrometer sized aggregates when mixed with alginate (polysaccharides),35 whereas 89 nm amine modified polystyrene particles increased little in size in algae conditioned media, but formed aggregates about 300 nm in medium conditioned by D. magna.34 However, as the concentration of particles and biomolecules will influence both the aggregation mechanism and final aggregation size, more data is needed to compare the fate of particles in different environments.
Polystyrene particles modified with negatively charged groups, with similar diameter as the amine modified particles, are not toxic in acute toxicity test to adult D. magna,29 suggesting that the positive charge on the surface makes the PS-NH2 toxic. Increased toxicity of amine modified polystyrene particles have been noted before.29,30,34,36 Incubating the PS-NH2 with negatively charged biomolecules will decrease the z-potential,36 indicating that the surface charge changes when the proteins bind, which generally reduces the effect of amine modified particles. Interestingly, the PS-NH2-IgG aggregates and the albumin stabilized particles were as toxic to D. magna as non-coated particles. This might indicate that it is the amine group itself that carry the toxicity and not the net positive charge of the surface.
The mass concentrations (1.4 and 2.7 mg L−1) used in our acute toxicity test were likely higher than those found in natural ecosystems. Although natural concentrations are yet unknown, they are rapidly increasing worldwide both through degradation of microplastics and through production of nanomaterials. Possibly, concentrations also differ between systems and some areas are likely hot spots, such as sewage treatment plants and waters downstream landfills. Hence, in such hot spots we may predict that effects similar to those identified in our study can occur. Furthermore, the effects from long term exposure of low concentrations of plastic nanoparticles on zooplankton are not well known and may initially be difficult to detect since it may not lead to mortality, but other metabolic effects,33,38 and notably even in the second generation.39 In any case, we here demonstrate that at similar concentrations the mortality of D. magna was similar between single 50 nm PS-NH2 particles and aggregates, i.e. aggregation will not render the particles less toxic than before. Hence, we can rule out the possibility that previously observed severe effects from free nanoparticles would diminish as the free particles aggregate when entering natural ecosystems.
Experimental
Materials
Amine modified polystyrene nanoparticles (PS-NH2 NPs) with diameter sizes of 50, 200, and 500 nm (Bangs Laboratories Inc., Fishers, Indiana, USA, www.bangslabs.com) were used in the present study, as well as human immunoglobulin G (IgG) (Meridian Life Science, Inc., Memphis, Tennessee, USA). The nanoparticles and the IgG were dialyzed against 10 L Millipore water for 48 h, with the water exchanged three times. The dialyzed nanoparticles were then stored at 4 °C, while the dialyzed IgG was aliquoted and frozen at −18 °C.
Aggregate formation
To achieve reproducible PS-NH2-IgG aggregates, several parameters, such as buffer strength and pH, NaCl concentration, and NP concentration, had to be optimized (see ESI†). The final aggregates were prepared by mixing 1.0 mg mL−1 IgG and 10 mg mL−1 50 nm NPs in 10 mM sodium phosphate buffer (pH 7.5), 20 mM NaCl. The solution was incubated for 1 h at 23 °C. Thereafter the aggregates were carefully pelleted by centrifugation at 3000 rpm, 16 °C, for 20 min, and resuspended. Dynamic light scattering (DLS) of the supernatant showed particle sizes in the supernatant of around 60 nm, suggesting that the centrifugation speed was low enough for free 50 nm particles in the sample to remain dispersed.
Samples of the aggregates were centrifuged at 3000 rpm for 20 min, at 16 °C, and the pellet freeze-dried and its weight measured, showing a concentration yield of approximately 54.8% (±7.2%) of the initial NPs concentration.
Characterization of NP size distribution
Dynamic light scattering (DLS) measurements were performed using a Wyatt DynaPro Plate Reader II (Wyatt Technology, Santa Barbara, USA) equipped with a laser at wavelength 830 nm and operating with a 158° scattering angle. Corning® 96 well non-binding plates (Corning 3881, Massachusetts, USA) were used, with a sample volume of 100 μL per well and controlled temperature of 25 °C. For each sample, 10 images were acquired and processed and the data were analyzed using the accompanying software Dynamics, version 7.2.1. Nanoparticle tracking analysis (NTA) measurements were performed using a NanoSight LM10 (Nanosight Ltd., Amesbury, UK) equipped with a 635 nm laser and the data analyzed using the software NTA 2.3 (Nanosight Ltd., Amesbury, UK). Samples of 400 μL volume were measured in triplicates, at room temperature, for 60 s. The differential centrifugal sedimentation (DCS) measurements were performed using a CPS Disc Centrifuge (DC24000 UHR Disc Centrifuge, CPS Instruments Europe, Oosterhout, Netherlands). Samples of 100 μL volume were loaded onto a 2–8% sucrose gradient and analyzed using the accompanying software. Finally, the aggregation of the NPs was examined by measuring the turbidity of the sample, by monitoring the absorbance at 400 nm using a ProbeDrum (Probation Labs, Lund, Sweden), samples of 800 μL were transferred to polystyrene cuvettes, and the absorbance monitored at 400 nm.
Study organism
The freshwater zooplankton species Daphnia magna, originating from Lake Bysjön (55° 40′ 31.3′′ N, 13° 32′ 41.9′′ E) were kept under controlled laboratory conditions for more than 100 generations, was used in the present study. The D. magna cultures were fed ad libitum 2–3 times a week with a culture of the green algae Scenedesmus sp. All cultures were maintained at 18 °C at a 8
:
16 h light/dark photoperiod.
Acute Daphnia magna toxicity test
Stock solutions of each NP and aggregate sample, with a final concentration of 5.5 mg mL−1, were prepared in 10 mM sodium phosphate buffer (pH 7.5), 20 mM NaCl, and incubated for 1 h. The aggregates were then centrifuged at 3000 rpm for 20 min at 16 °C. An aliquot of the stock solutions of the samples – either controls, particles, or aggregates – were added to the D. magna, with one individual in 25 mL tap water, with ten replicates of each treatment. In order to determine the effect of free particles left in the aggregate samples, one of these samples were centrifuged at 3000 rpm for 20 min at 16 °C, and the supernatant (referred to as resuspension supernatant) was added to the D. magna as well. The survival of D. magna was monitored every hour for 24 or 48 h. Water samples were collected at time 0, 12, and 24 h the samples collected after 24 h were analyzed by NTA. The statistical analysis for the Kaplan–Meier survival curves was performed with a Mantel–Cox log-rank test using the software GraphPad Prism version 8.4.2 for Windows, GraphPad Software, Inc., www.graphpad.com. In control experiments the pH was measured before and after exposure of the 50 nm particles. The pH changed from 6.3 to 6.0 in the experiments with or without particles.
Albumin coated particles
Bovine serum albumin (BSA, A7030) was from Sigma Aldrich (www.sigmaaldrich.com). Prior to the experiment, BSA were diluted to 10 mg mL−1 and dialyzed extensively in Standard RC Tubing, dialysis membrane (MWCO: 3.5 kD) for 24 h in 10 L of water, with a water exchange at least 4 times. Nanoparticles were incubated with ten folds the concentration of BSA and stored at the room temperature for 20 min to ensure a complete coating. Sizes of particles were measured in triplicates using DLS on DynaPro Plate Reader II (Wyatt instruments, USA) after particles preparation and after 24 h to ensure particles stability of both non-coated and coated particles. The diameter of non-coated particles was 46 nm and did not change with time, whereas the diameter of coated particles was 66 nm immediately after mixing and 56 nm after 24 h, indicating that a stable monolayer was formed with time.
Non-coated or coated nanoparticles, 2.7 mg L−1, and, 27 mg L−1 for BSA were used to test the effect on D. magna. One individual from the same population was placed into 15 mL tubes with 5 mL of water or water with particles (n = 10 for each treatment) and the survival was monitored for 48 h. The presence of BSA on the particles after filtration was evaluated by adding non-coated or coated particles to 15 D. magna individuals in 5 mL tap water. After 24 h exposure, individuals were removed from the water and the water samples were centrifuged in 1.5 mL Eppendorf tubes at 18
000 rpm for 20 min at 24 °C using a Mikro 220R Hettich centrifuge. The proteins from the pellet after repeated centrifugations were resorbed by SDS-PAGE sample buffer, 60 mM Tris, pH 6.8, 10% SDS, 10% glycerol, with bromophenol blue. The proteins were separated by electrophoresis and the bands visualized by Coomassie blue staining.
Conflicts of interest
There are no conflicts to declare.
Acknowledgements
Funding for the present study was provided by Swedish Council for the Environment (FORMAS), the Swedish Research Council (VR), the Swedish Environmental Protection Agency and the MISTRA Environmental NanoSafety program.
References
-
P. Europe, Plastics - the facts 2019 [Available from: https://www.plasticseurope.org/application/files/6315/4510/9658/Plastics_the_facts_2018_AF_web.pdf.
- R. C. Thompson, C. J. Moore, F. S. vom saal and S. H. Swan, Plastics, the environment and human health: current consensus and future trends, Philos. Trans. R. Soc. London, Ser. B, 2009, 364(1526), 2153–2166 CrossRef CAS PubMed.
- L. C. M. Lebreton, J. van der Zwet, J.-W. Damsteeg, B. Slat, A. Andrady and J. Reisser, River plastic emissions to the world's oceans, Nat. Commun., 2017, 8, 15611 CrossRef CAS PubMed.
- R. C. Thompson, Y. Olsen, R. P. Mitchell, A. Davis, S. J. Rowland and A. W. G. John,
et al. Lost at Sea: Where Is All the Plastic?, Science, 2004, 304(5672), 838 CrossRef CAS PubMed.
- J. P. da Costa, P. S. M. Santos, A. C. Duarte and T. Rocha-Santos, (Nano)plastics in the environment - Sources, fates and effects, Sci. Total. Environ., 2016, 566–567, 15–26 CrossRef PubMed.
- B. Gewert, M. M. Plassmann and M. MacLeod, Pathways for degradation of plastic polymers floating in the marine environment, Environ. Sci.: Processes Impacts, 2015, 17(9), 1513–1521 RSC.
- A. Jahnke, H. P. H. Arp, B. I. Escher, B. Gewert, E. Gorokhova and D. Kühnel,
et al. Reducing Uncertainty and Confronting Ignorance about the Possible Impacts of Weathering Plastic in the Marine Environment, Environ. Sci. Technol. Lett., 2017, 4(3), 85–90 CrossRef CAS.
- A. Ter Halle, L. Jeanneau, M. Martignac, E. Jardé, B. Pedrono and L. Brach,
et al. Nanoplastic in the North Atlantic Subtropical Gyre, Environ. Sci. Technol., 2017, 51(23), 13689–13697 CrossRef CAS PubMed.
- S. M. Mintenig, P. S. Bäuerlein, A. A. Koelmans, S. C. Dekker and A. P. van Wezel, Closing the gap between small and smaller: towards a framework to analyse nano- and microplastics in aqueous environmental samples, Environ. Sci.: Nano, 2018, 5(7), 1640–1649 RSC.
- B. G. Kwon, K. Amamiya, H. Sato, S. Y. Chung, Y. Kodera and S. K. Kim,
et al. Monitoring of styrene oligomers as indicators of polystyrene plastic pollution in the North-West Pacific Ocean, Chemosphere, 2017, 180, 500–505 CrossRef CAS PubMed.
- B. G. Kwon, K. Saido, K. Koizumi, H. Sato, N. Ogawa and S. Y. Chung,
et al. Regional distribution of styrene analogues generated from polystyrene degradation along the coastlines of the North-East Pacific Ocean and Hawaii, Environ. Pollut., 2014, 188, 45–49 CrossRef CAS PubMed.
- M. T. Ekvall, M. Lundqvist, E. Kelpsiene, E. Šileikis, S. B. Gunnarsson and T. Cedervall, Nanoplastics formed during the mechanical breakdown of daily-use polystyrene products, Nanoscale Adv., 2019, 1(3), 1055–1061 RSC.
- J. Gigault, B. Pedrono, B. Maxit and A. Ter Halle, Marine plastic litter: the unanalyzed nano-fraction, Environ. Sci.: Nano, 2016, 3(2), 346–350 RSC.
- S. Lambert and M. Wagner, Characterisation of nanoplastics during the degradation of polystyrene, Chemosphere, 2016, 145, 265–268 CrossRef CAS PubMed.
- M. González-Pleiter, M. Tamayo-Belda, G. Pulido-Reyes, G. Amariei, F. Leganés and R. Rosal,
et al. Secondary nanoplastics released from a biodegradable microplastic severely impact freshwater environments, Environ. Sci.: Nano, 2019, 6(5), 1382–1392 RSC.
- Y. Chae and Y. J. An, Effects of micro- and nanoplastics on aquatic ecosystems: Current research trends and perspectives, Mar. Pollut. Bull., 2017, 124(2), 624–632 CrossRef CAS PubMed.
- K. Mattsson, L. A. Hansson and T. Cedervall, Nano-plastics in the aquatic environment, Environ. Sci.: Processes Impacts, 2015, 17(10), 1712–1721 RSC.
- E. Besseling, J. T. K. Quik, M. Sun and A. A. Koelmans, Fate of nano- and microplastic in freshwater systems: A modeling study, Environ. Pollut., 2017, 220, 540–548 CrossRef CAS PubMed.
- S. Kashiwada, Distribution of nanoparticles in the see-through medaka (Oryzias latipes), Environ. Health Perspect., 2006, 114(11), 1697–1702 CrossRef CAS PubMed.
- W. S. Lee, H.-J. Cho, E. Kim, Y. H. Huh, H.-J. Kim and B. Kim,
et al. Bioaccumulation of polystyrene nanoplastics and their effect on the toxicity of Au ions in zebrafish embryos, Nanoscale, 2019, 11(7), 3173–3185 RSC.
- Y. Lu, Y. Zhang, Y. Deng, W. Jiang, Y. Zhao and J. Geng,
et al. Uptake and Accumulation of Polystyrene Microplastics in Zebrafish (Danio rerio) and Toxic Effects in Liver, Environ. Sci. Technol., 2016, 50(7), 4054–4060 CrossRef CAS PubMed.
- M. Manabe, N. Tatarazako and M. Kinoshita, Uptake, excretion and toxicity of nano-sized latex particles on medaka (Oryzias latipes) embryos and larvae, Aquat. Toxicol., 2011, 105(3–4), 576–581 CrossRef CAS PubMed.
- P. Rosenkranz, Q. Chaudhry, V. Stone and T. F. Fernandes, A comparison of nanoparticle and fine particle uptake by Daphnia magna, Environ. Toxicol. Chem., 2009, 28(10), 2142–2149 CrossRef CAS PubMed.
- M. van Pomeren, N. R. Brun, W. J. G. M. Peijnenburg and M. G. Vijver, Exploring uptake and biodistribution of polystyrene (nano)particles in zebrafish embryos at different developmental stages, Aquat. Toxicol., 2017, 190, 40–45 CrossRef CAS PubMed.
- S. Rist, A. Baun and N. B. Hartmann, Ingestion of micro- and nanoplastics in Daphnia magna - Quantification of body burdens and assessment of feeding rates and reproduction, Environ. Pollut., 2017, 228, 398–407 CrossRef CAS PubMed.
- T. Cedervall, L.-A. Hansson, M. Lard, B. Frohm and S. Linse, Food Chain Transport of Nanoparticles Affects Behaviour and Fat Metabolism in Fish, PLoS One, 2012, 7(2), e32254 CrossRef CAS PubMed.
- Y. Chae, D. Kim, S. W. Kim and Y.-J. An, Trophic transfer and individual impact of nano-sized polystyrene in a four-species freshwater food chain, Sci. Rep., 2018, 8(1), 284 CrossRef PubMed.
- K. Mattsson, M. T. Ekvall, L. A. Hansson, S. Linse, A. Malmendal and T. Cedervall, Altered behavior, physiology, and metabolism in fish exposed to polystyrene nanoparticles, Environ. Sci. Technol., 2015, 49(1), 553–561 CrossRef CAS PubMed.
- K. Mattsson, E. V. Johnson, A. Malmendal, S. Linse, L. A. Hansson and T. Cedervall, Brain damage and behavioural disorders in fish induced by plastic nanoparticles delivered through the food chain, Sci. Rep., 2017, 7(1), s11452 CrossRef PubMed.
- E. Bergami, S. Pugnalini, M. L. Vannuccini, L. Manfra, C. Faleri and F. Savorelli,
et al. Long-term toxicity of surface-charged polystyrene nanoplastics to marine planktonic species Dunaliella tertiolecta and Artemia franciscana, Aquat. Toxicol., 2017, 189, 159–169 CrossRef CAS PubMed.
- C. B. Jeong, E. J. Won, H. M. Kang, M. C. Lee, D. S. Hwang and U. K. Hwang,
et al. Microplastic Size-Dependent Toxicity, Oxidative Stress Induction, and p-JNK and p-p38 Activation in the Monogonont Rotifer (Brachionus koreanus), Environ. Sci. Technol., 2016, 50(16), 8849–8857 CrossRef CAS PubMed.
- K.-W. Lee, W. J. Shim, O. Y. Kwon and J.-H. Kang, Size-Dependent Effects of Micro Polystyrene Particles in the Marine Copepod Tigriopus japonicus, Environ. Sci. Technol., 2013, 47(19), 11278–11283 CrossRef CAS PubMed.
- E. Besseling, B. Wang, M. Lürling and A. A. Koelmans, Nanoplastic Affects Growth of S. obliquus and Reproduction of D. magna, Environ. Sci. Technol., 2014, 48(20), 12336–12343 CrossRef CAS PubMed.
- F. Nasser and I. Lynch, Secreted protein eco-corona mediates uptake
and impacts of polystyrene nanoparticles on Daphnia magna, J. Proteomics, 2016, 137, 45–51 CrossRef CAS PubMed.
- O. Oriekhova and S. Stoll, Heteroaggregation of nanoplastic particles in the presence of inorganic colloids and natural organic matter, Environ. Sci.: Nano, 2018, 5(3), 792–799 RSC.
- J. Saavedra, S. Stoll and V. I. Slaveykova, Influence of nanoplastic surface charge on eco-corona formation, aggregation and toxicity to freshwater zooplankton, Environ. Pollut., 2019, 252(Pt A), 715–722 CrossRef CAS PubMed.
- R. Cukalevski, S. A. Ferreira, C. J. Dunning, T. Berggård and T. Cedervall, IgG and fibrinogen driven nanoparticle aggregation, Nano Res., 2015, 8(8), 2733–2743 CrossRef CAS.
- Z. Liu, P. Yu, M. Cai, D. Wu, M. Zhang and Y. Huang,
et al. Polystyrene nanoplastic exposure induces immobilization, reproduction, and stress defense in the freshwater cladoceran Daphnia pulex, Chemosphere, 2019, 215, 74–81 CrossRef CAS PubMed.
- Z. Liu, M. Cai, D. Wu, P. Yu, Y. Jiao and Q. Jiang,
et al. Effects of nanoplastics at predicted environmental concentration on Daphnia pulex after exposure through multiple generations, Environ. Pollut., 2020, 256, 113506 CrossRef CAS PubMed.
Footnote |
† Electronic supplementary information (ESI) available. See DOI: 10.1039/c9en01236b |
|
This journal is © The Royal Society of Chemistry 2020 |