Testing the bioaccumulation of manufactured nanomaterials in the freshwater bivalve Corbicula fluminea using a new test method†
Received
29th September 2019
, Accepted 8th December 2019
First published on 8th January 2020
Abstract
Increasing amounts of manufactured nanomaterials (MNMs) are produced for their industrial use and released to the environment by the usage or disposal of the products. As depending on their annual production rate, substances are subjected to PBT assessment, the availability of reliable methods to evaluate these endpoints for (corresponding) nanoforms/MNMs becomes relevant. The classical method to elucidate the bioaccumulation potential of chemicals has been the flow-through study with fish, which has limitations as regards meeting the requirements of MNMs. Most MNMs tend to sediment in the aquatic environment. Thus, maintenance of stable exposure conditions for bioaccumulation testing with fish is nearly impossible to achieve when using MNMs. Corbicula fluminea, a freshwater filter-feeding bivalve distributed worldwide, has been previously shown to ingest and accumulate MNMs present in the water phase. To investigate the suitability of C. fluminea for bioaccumulation testing we developed a new flow-through system to expose mussels under constant exposure conditions. Two nanoparticles (NPs), the AgNP NM 300K and the TiO2NP NM 105, were applied. In addition, C. fluminea was exposed to AgNO3 as a source of dissolved Ag+ to compare the bioaccumulation of Ag in dissolved and nanoparticulate forms. For each MNM exposure scenario we were able to determine steady-state bioaccumulation factors. BAFss values of 31 and 128 for two NM 300K concentrations (0.624 and 6.177 μg Ag per L) and 6150 and 9022 for TiO2 (0.099 and 0.589 μg TiO2 per L) showed the exposure dependence of the BAFss estimates. The progression of metal uptake and elimination in the soft tissue provided clear indications that the uptake and thus accumulation is mainly driven by the uptake of NPs and less of dissolved ions.
Environmental significance
Filter-feeding organisms such as bivalves represent a major target for the bioaccumulation of nanomaterials in the aquatic environment. Therefore, bivalves should be considered as test organisms for the bioaccumulation assessment of nanomaterials. A new flow-through system to expose the freshwater bivalves under constant exposure conditions was developed. Bioaccumulation studies with the freshwater bivalve C. fluminea on two nanoparticles, the AgNP NM 300K and the TiO2NP NM 105, demonstrated the suitability of the new test system. The results obtained with this test system can be used to generate useful endpoints required for regulatory purposes and could be included in a tiered bioaccumulation testing strategy for manufactured nanomaterials.
|
Introduction
The European Commission estimated the global amount of produced manufactured nanomaterials (MNMs) to be 11.5 million tons per year which corresponds to a market value of €20bn.1 Further industrialization and economic expansion of industrial countries and economies will increase the production and usage of MNMs. MNMs are released to the environment during their production, their use and the disposal of MNM-containing products.2–4 The increasing production of MNMs leads inevitably to a larger environmental burden and a reliable environmental risk assessment of MNMs is thus required.5
Due to the high annual production of corresponding bulk substances, several MNMs are subject to assessment of bioaccumulation such as required by the European Chemicals Registration REACH,6 the Japanese Chemical Substance Control Act “Kashinho” or others, e.g. HPV, TCFCA or KKDIK.7–10
Under REACH, the bioconcentration factor (BCF) represents the most important endpoint for bioaccumulation assessment.11 The BCF is mostly determined by fish flow-through studies in accordance with OECD test guideline 305 which was developed for water-soluble test items.12,13 However, dispersed MNMs in aquatic systems represent meta-stable systems that are only kinetically stabilized and tend to agglomerate and sediment, leading to problems with respect to the maintenance of stable and continuous exposure conditions during classic flow-through studies with MNMs. Thus, the establishment of suitable experimental conditions is difficult and consequently there is a need to develop suitable test methods adapted to the specific need of testing MNMs. The REACH regulation was developed without considering the specific properties of MNMs, and thus the development of more suitable test methods was suggested.14,15
In 2018, Handy et al.16 presented a tiered testing approach to meet the requirements of MNM bioaccumulation testing that also includes bioaccumulation tests with invertebrates which provide indications that may allow a waiver of further studies using fish as the test organism.
This is in accordance with the Guidance on Information Requirements and Chemical Safety Assessment of REACH where it is mentioned that taxonomic groups other than fish are allowed to gain data for the assessment of the B criteria if representing a relevant target organism.17 Within this guidance the mussel bioconcentration test of the ASTM is given as an example.11,18
Marine and freshwater bivalves are widely used and established as bioindicators for pollution in aquatic systems, e.g. due to their ability to accumulate high concentrations of heavy metals in their tissue.19–23 Moreover, bivalves are used for the determination of the bioavailability and effects of xenobiotics and genotoxic compounds.22,24,25 Filter-feeding bivalves may ingest considerable amounts of particulate materials that are concentrated in their feces or pseudo-feces.26,27 As described by Hull et al.,28 these particulate materials could be MNMs that are dispersed in the water. The filter-feeding behavior of bivalves for respiratory and nutritional purposes and their benthic mode of life make bivalves a group of organisms predominantly exposed to MNMs.29–34 Mesocosm studies of Ferry et al.35 showed that bivalves represent an important sink for MNMs, and similar results were presented by Cleveland et al.36 Because the feces and pseudo-feces of bivalves represent an important part of the diet of benthic invertebrates, they may play a key role regarding the transfer of MNMs into the aquatic food chain.37,38 Bivalves may also represent a link between the aquatic and the terrestrial environment being part of the diet of water birds.39,40
The freshwater bivalve Corbicula fluminea is an invasive species that is, due to its ability to tolerate a wide range of environmental conditions, widely spread in Africa, North and South America, Europe and the Pacific Islands.41–44 The high filtration rate of this species makes C. fluminea ideal for use in bioaccumulation test systems.45 Due to the euryoecious characteristics of this species, C. fluminea can be used in test systems that are highly adjustable to meet the requirements of the wide spectrum of different MNMs.
The aim of this study was to develop a flow-through system that allows a continuous and constant exposure of MNMs to determine the bioavailability and bioaccumulation of MNMs in bivalves. Based on the above facts, C. fluminea was used as the test species. Silver nanoparticles (NM 300K) and the titanium dioxide NP NM 105 were selected as test items representing typical MNMs from the European Commission's Joint Research Centre repository.46,47 AgNPs are mainly employed due to their antibacterial properties and belong to the most investigated MNMs.36,48–52 The antibacterial effects are based on released silver ions (Ag+) that can cause disruption of the respiratory chain of the cells, the deactivation of proteins and the disturbance of membrane transport processes.53–55 To compare the fate and potential bioaccumulation of AgNPs and Ag+, we also tested AgNO3 as a source of dissolved Ag+.
In contrast to AgNPs, TiO2NPs are nearly chemically inert, representing one of the most commonly used MNMs56 belonging to the group of non-ion-releasing MNMs under environmental conditions and showing condition-dependent dispersion stability.57 TiO2NPs are of great ecotoxicological interest due to their potential to alter the bioavailability and thus the toxicity of coexisting contaminants like heavy metals or organic compounds in aquatic organisms like fish and bivalves.58–63
For the evaluation of the bioaccumulation potential of the MNMs applied in this study, total metal concentrations were analysed in the whole animals and the distribution of accumulated material in the soft tissue was determined. In addition, particle concentrations and particle sizes were measured in the different tissue compartments of the animals to allow the differentiation between bioaccumulation of dissolved and particulate material. Similarly, dispersions applied in this study were characterized for their concentrations and particle size distribution. A promising technique for analyzing particle sizes and numbers at low concentrations is single-particle ICP-MS (spICP-MS).64,65 The underlying concept is based on the measurement of diluted suspensions, enabling the introduction of individual particles into the ICP-MS plasma. For the analysis of bioaccumulated MNMs by spICP-MS, particles have to be extracted from the biological matrix under mild conditions to avoid dissolution of the particles (e.g. AgNPs) and changes in their state, e.g. in terms of their size distribution. Therefore, mild extraction procedures such as enzymatic digestion are required.66 The development of a workflow for the analysis of MNMs bioaccumulated in mussel tissue using spICP-MS was part of this study and is presented.
Methods
Corbicula fluminea
Freshwater bivalves C. fluminea used in this study were collected from the river Niers near Wachtendonk (47669, Germany) and kept in 1.5 m3 glass microcosms. Up to 2000 animals were placed in 3 stainless steel baskets within a microcosm with regular water change (copper-reduced tap water) every three weeks. Water was aerated with two airstones per microcosm, and slight water circulation was provided by a circulation pump; the water temperature was around 12 ± 4 °C. Animals were fed with 0.5 L of a suspension containing 200 mg of fine-milled stinging nettle leaves67 (BRENNNESSEL TEE N, Aurica®) per liter after each water change. Microcosms were checked for dead animals at least 3 times a week and carcasses were removed from the microcosms. An acclimatization phase of at least 2 weeks after harvesting of the bivalves from the river was required before the usage of the animals in the studies. For the studies, only individuals with a length (anterior–posterior) of 2.5 (±0.5) cm were used. The length of the animals was measured as described below.
Test system
A flow-through system was developed to allow continuous exposure of MNM containing test media at constant concentrations (Fig. 1). The central part of the new test system is a Zuger glass jar test vessel with a volume of 8 L. Within this vessel a V4A stainless steel rack allows placement of up to 170 bivalves (shell length in the range of 2–2.5 cm) on perforated shelves. Adjustable aeration as well as a stirrer (RZR 1, Heidolph) with adjustable spin rates from 35 to 2200 rpm can be used to ensure sufficient aeration and constant mixing of the test media. Stock solution (MNM suspension) and food suspension are added to a mixing vessel using peristaltic pumps (IPC High Precision Multichannel Dispenser, ISMATEC®) and further diluted with copper-reduced tap water supplied by a membrane pump (gamma/X, ProMinent®) to produce the test medium which is supplied into the Zuger glass jar using TYGON® tubes (E-3603, TYGON®). The whole system allows a flow rate from <0.5 L h−1 to >20 L h−1. The test medium leaves the test system at the bottom of the Zuger glass jar by an overflow pipe.
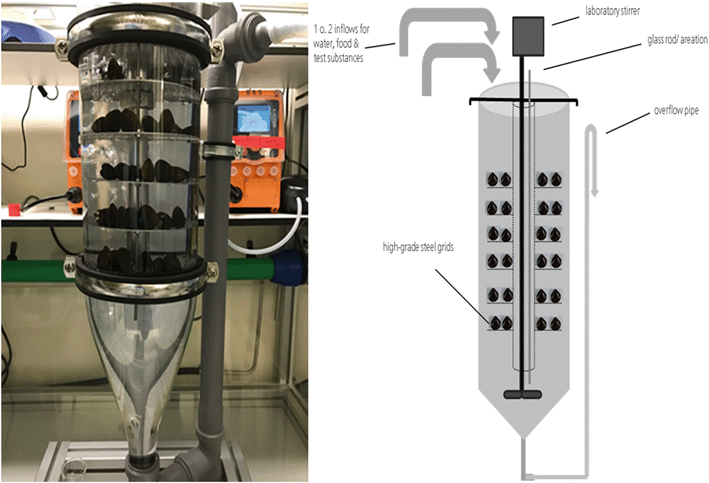 |
| Fig. 1 Bivalvia flow-through system containing C. fluminea. | |
Feed evaluation study
A feeding study lasting 192 h was carried out to identify a suitable experimental diet for bioaccumulation studies with the freshwater bivalve C. fluminea. Therefore, 5 potential diets were tested in the newly developed test system: Spirulina species (SP), ground stinging nettle (SN), ground plant-based fish food tablets (FFT) (https://www.ms-tierbedarf.de/), a combination of ground stinging nettle and fish food tablets (SN + FFT), and a no food treatment (/). The test system ran for 24 h before the test started to allow equilibration of experimental conditions.
For the feeding study, 5 flow-through systems (one unit per treatment) were stocked with 50 animals each, which were pre-conditioned in microcosms for several weeks. Prior to the test the animals were brushed and transferred into a fresh microcosm without any food source in order to promote defecation. After 24 h, the cleaning procedure was repeated and animals were transferred into a second microcosm for 24 h prior to being transferred into the test systems. Before this, the length of the animals was measured using a ruler and their valves were dried using paper towels to allow the accurate measurement of the animals' weight (AUW220D, SHIMADZU). During the dietary test, the test systems were supplied with test medium (copper-reduced tap water) at a flow rate of 4 L h−1 and each liter of test medium contained 16 mL of food suspension (equivalent to 400 mg dry mass per L). The temperature, dissolved oxygen, and the pH value in the test system were measured daily. Measurements of ammonia, nitrite and nitrate were carried out by photometric measurements (NANOCOLOR® 500D, Machery-Nagel) at the start and end of the test. During the dietary test the valve opening filtration activity was monitored by visual judgement. At the end of the dietary test, dead animals were counted. The valves of the live animals were cleaned and dried, and the length and weight of each animal was measured (AUW220D, SHIMADZU). The mortality, average weight and length of the animals were calculated for each treatment.
Bioaccumulation studies
Preparation of stock suspensions.
Bioaccumulation studies were carried out with NM 300K as a test material representing well-dispersed and ion-releasing AgNPs. AgNO3 was used as a non-nano structural and soluble species of Ag. NM 105 (TiO2) was used as a test material representing a non-ion-releasing MNM with a tendency to agglomerate.47 Both NMs are representative test and reference materials from the European Commission's Joint Research Centre and in the scope of the OECD Working Party on Manufactured Nanomaterials (WPMN) Sponsorship Program and were provided by the Fraunhofer Institute for Molecular Biology and Applied Ecology IME. Information on the characterisation and physico-chemical properties of the NPs is summarized in the JRC Reports for NM 300K and the titanium dioxide series.46,47 Electron microscopy was performed on the feedstock materials (Fig. S10 and S11†); the acquired grain size distributions are in accordance with the data from the JRC Reports. The stock suspension of NM 300K contains 10.16% (w/w) AgNPs having an average particle size of 15 nm.46 The NM 300K suspension was stabilized with a dispersing agent (NM-300 DIS) containing 4% (w/v) polyoxyethylene, glycerol, trioleate, and polyoxyethylene (20) sorbitan monolaurate (Tween 20) each.68 For the production of the AgNP working suspension, 300 mg of NM 300K were diluted with 30 ml ultra high quality water (UHQ-water), hand-shaken for 1 min and sonicated for 10 min with a pulsation pause ratio of 0.2/0.8 using an ultrasonic homogenizer (Bandelin Sonopuls HD2200 ultrasonic homogenizer, 200 W, Bandelin Cup Horn BB6) to disperse the NM 300K and to carefully homogenize the suspension for the dilution with UHQ to achieve the final concentration of 834 μg Ag per L. During the sonication process, the suspension (contained in a 50 ml centrifugal tube, PP) was cooled with ice water in the cup horn. AgNO3 was purchased from Carl Roth with a purity of >99.9% and diluted with UHQ-water for the preparation of the stock solution. NM 105 was present in the form of a powder and was also suspended in UHQ-water as described above for NM 300K.
Performance of bioaccumulation studies.
In three bioaccumulation tests NM 300K, AgNO3 and NM 105 were tested in two concentrations each with 170 mussels per test. As described for the dietary test, the shell of all bivalves was cleaned before the animals were transferred into the test vessels. Again, only individuals with a length (anterior–posterior) of 2.5 (±0.5) cm were used. During none of the studies, the stirrer which is part of the test system was applied. The NM 300K working suspension (834 μg Ag per L) was produced by dilution of the NM 300K stock suspension after sonication with UHQ-water. The AgNO3 working solution (834 μg Ag per L) was produced by dissolving a defined amount of AgNO3 in UHQ-water. The NM 105 stock suspension was diluted after sonication using UHQ-water. The diluted suspension (1 mg TiO2 per L) was allowed to settle for 72 h in 2 L glass beakers at room temperature. After decanting, the suspension with the remaining stable dispersed fraction was used as the working suspension. Test media were composed of copper-reduced tap water, a suspension of ground stinging nettle tea (400 mg dry mass per L) and the respective MNM suspension or AgNO3 solution and were applied at a flow rate of 4 L h−1. In the case of NM 300K and AgNO3 the working suspension/solution was pumped into the mixing vessel before being introduced into the test vessel. The NM 105 working suspension was introduced directly into the test vessel to avoid sedimentation processes in the mixing vessel. The working suspension was discharged into the test system at three different surface positions of the water body to ensure an even distribution of the TiO2NPs in the test water which was constantly supplied into the test vessel at a flow rate of 4 L h−1.
The test system was allowed to equilibrate for at least 48 h before the test start until constant flow through conditions and stable media concentrations (variation of concentration ≤20%) were reached for at least three sampling times, separated by at least 3 h. Measurements of ammonia, nitrite and nitrate were carried out at the start and end of the uptake and elimination phase to check the culture conditions in the test system during the study. Animals were exposed for 144 h (AgNO3), 96 h (NM 300K), and 120 h (NM 105). Following the uptake phase, previously exposed animals were placed in a new test vessel and maintained under the same experimental conditions but without the test item for 144 h (AgNO3), 48 h (NM 300K), and 144 h (NM 105). Triplicate samples of three animals were taken from the test system at different sampling dates during the uptake and elimination phase as shown in Fig. 2. The shells of collected animals were cleaned. Disposable scalpels (Cutfix Fig. 10, B. Braun) were used to open the shells by gently pressing the blade between the shells at the position of the siphons. Before levering the shells, the blade was slid to the posterior and anterior end to sever the adductor muscles. The opened bivalves were cleaned by dipping and moving the animal in two separate wash boxes filled with UHQ-water. The soft tissue was then removed from the shells using the disposable scalpels, blotted with lint free laboratory paper and weighed. All tissue samples were immediately frozen using liquid nitrogen and stored at −20 °C until further processing for determination of total Ag or Ti concentrations. Prior to the collection of test animals, duplicate media samples were taken for the measurement of media concentrations. In addition, media samples were taken from different levels of the test system to check whether homogeneous concentrations of test item were applied. In the case of the Ag treatments, the samples (20 mL) were acidified by adding 200 μL of nitric acid (69%, suprapure grade, Roth) and stored at 4 °C. Media samples for single particle measurements were not acidified and measured directly. The appropriate duration of the uptake phase to ensure steady-state conditions was estimated for each test item in a pretest. Depuration phases following the uptake phase were continued for as long as enough bivalves were available. Due to the increased loss of animals exposed to the high concentrations of AgNO3 and NM 300K, only a few samples could be obtained during the depuration periods.
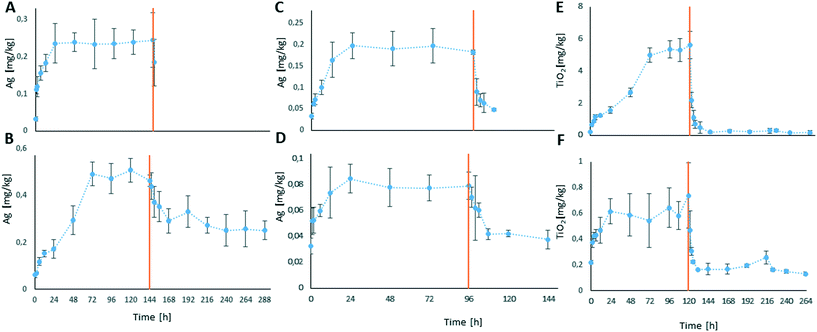 |
| Fig. 2 Total Ag and TiO2 concentrations in the mussel soft tissue during the bioaccumulation studies. Red vertical line shows the end of the uptake phase. (A) AgNO3 high exposure concentration, (B) AgNO3 low exposure concentration n, (C) NM 300K high exposure concentration, (D) NM 300K low exposure concentration, (E) NM 105 high exposure concentration, and (F) NM 105 low exposure concentration. | |
Determination of total metal concentrations
Total silver and titanium concentrations in the aqueous test media were determined by inductively coupled plasma mass spectrometry (ICP-MS, Agilent 7700 ICP-Q-MS, Agilent Technologies, Waldbronn, Germany). The instrument calibration and method verification was carried out as described by Kühr et al.69 using certified element and multi-element standards (Merck) and reference material (TM 25.4; Environment Canada). A rhodium standard solution (Merck KGaA; CertiPUR) was applied as an internal standard for compensation of instrumental fluctuations. For each standard and sample, at least three measurements were recorded and the mean concentration was determined using the ICP-MS software. The test media samples (20 mL) which were acidified after sampling were measured directly by ICP-MS. The tissue samples were digested using a microwave (MLS Ultra Clave) for the determination of total metal concentrations. First, 5 mL nitric acid (69%, suprapure grade) were added to the tissue samples followed by vortex stirring (VORTEX GENIE 2, Si™ Scientific Industries). Samples were transferred to the microwave and digested (max. temperature: 220 °C, max. pressure: 95 bar, energy: 1 kW h). The digested samples were diluted up to 15 mL with nitric acid (10%) and measured by ICP-MS.
Calculation of bioaccumulation factors
The data analysis software OriginPro 2017 (OriginLab Corporation) was used to subject all measured concentration data to an analysis of variance (ANOVA). Time-weighted average concentrations (TWA) of Ag and TiO2 in test media of the different studies were calculated for the uptake phases.70 Total Ag and TiO2 concentrations in the test animals' soft tissue, sampled under steady-state conditions were divided by the TWA concentrations of the media to gain bioaccumulation factors (BAFss) for the MNM treatments and steady-state bioconcentration factors (BCFss) for the AgNO3 treatment.
Investigations on tissue distribution
Performance of bioaccumulation studies.
Additional bioaccumulation studies were carried out with C. fluminea to further elucidate the uptake of the MNMs and AgNO3 in the soft tissue. The distribution of the total metal and MNM content in the different compartments of the animals was investigated to evaluate whether the determined elemental concentrations in the soft tissue derived from nanoparticles or dissolved metal. The tests were carried out with AgNO3, NM 300K and NM 105 under the same conditions as the main studies. The highest test concentrations tested in the bioaccumulation studies were applied to make the results comparable. The duration of the uptake phase was the same as in the main study to ensure that steady-state conditions were reached. The uptake phase was followed by a shortened elimination period. Animals for the analysis of compartment-specific differences were sampled at the end of the uptake period. To investigate the total metal concentrations as well as particle distribution in the different compartments, clean disposable scalpels (Cutfix Fig. 10, B. Braun) were used to separate the adductor muscle tissue (AM), the foot (F), the mantle including the siphons (M) and the remaining visceral mass (VM) (Fig. S8†). Five replicates consisting of five animals each were sampled to gain enough sample mass. In addition, triplicate samples, each consisting of 3 animals, were sampled for spICP-MS analysis during the uptake and elimination phase. Collected tissue samples were frozen immediately using liquid nitrogen and stored at −20 °C.
Calculation of distribution factors
The total metal concentrations were determined for the single compartments as described above and their contribution to the whole body burden was calculated.
Distribution factors (DFs) were calculated for each test item to clarify the differences between the single compartments of the animals using the following formula:
The mean concentration in the whole soft tissue was calculated by calculating the mean concentration of each single replicate based on the measured metal contents of the compartments of the respective replicate. All data were subjected to an outlier test (SQS 2013 Version 1.00 by J. Klein and G. Wachter). Replicates identified as outliers were excluded from further data analysis.
Determination of particle number concentrations and size distribution in test media and soft tissue
In addition to the determination of total metal concentrations in test media and in the mussel tissues, particle size distributions and particle number concentrations in the test media as well as in whole soft body and specific tissues were determined by spICP-MS. Samples of the test media were dispersed by ultrasonic treatment prior to appropriate dilution followed by analysis with spICP-MS. For the analysis of particles in mussel tissue a low perturbing sample preparation method was required that does not dissolve the particles and affects the particle properties as little as possible. Thus, tissue samples were digested using the enzyme proteinase K (Sigma Aldrich) according to the method described by Loeschner et al.66 and Schmidt et al.71 The defrosted tissues were incubated with 10 mL of the digestion solution per 400 mg (fresh weight) for 3 h at 50 °C and shaken at 100 rpm using an orbital shaker. The digestion solution was prepared by dissolving 45 mg proteinase K in 1 L buffer solution (0.5% SDS + 50 mM NH4HCO3, pH adjusted to 8.0–8.2). After complete dissolution of the tissue, which was confirmed by visual inspection, the solution was filtered using 0.45 μm syringe filters (Minisart® NML, 0.45 μm) and then measured using an ICP-QQQ-MS instrument (Agilent 8900, Agilent Technologies, Waldbronn, Germany). The possible influence of the digestion procedure on the particle size distributions was evaluated qualitatively. For this purpose, mussel tissue was spiked with an aliquot of the respective particle stock dispersion prior to digestion and the measured distribution was compared with that of the stock dispersion. Additionally, hydrolysates of mussel tissue not spiked/not exposed to the respective particles prior to digestion were spiked with an aliquot in order to assess the impact of the obtained solutions on the size distributions.
The dwell time in the single particle measurement mode of the ICP-MS was set to 100 μs and time resolved signals were recorded on the selected isotope for 60 s. Peak detection and integration was conducted automatically by the Agilent MassHunter software and converted into particle sizes. Dispersions of 60 nm gold nanoparticles (AuNPs 60 nm, BBI solutions, UK) were used for the determination of the nebulization efficiency and prepared freshly on the day of measurement. The samples were diluted in ultrapure water by a factor of 102–105 for measurement to reach a particle concentration of 200–2000 particle events per minute. According to Sannac, Tadjiki and Moldenhauer72 and Mitrano et al.73 this correlates to an element concentration in the range of ng L−1. Ag was measured as the isotope 107Ag. Titanium was measured as 48Ti in the NH3-reaction mode to minimize interferences with calcium. The threshold between background and particle signals was defined based on visual inspection of the measured signal distributions. The resulting histograms are shown in the form of histograms normalized to the most frequent particle size.
Results
Feed evaluation study
Animals used in the dietary test had an average weight of 68 (±0.79) g and average lengths of 26.84 (±1.23) mm (anterior–posterior) and 17.07 (±0.77) mm (ventral–dorsal). Temperature in the single treatment units ranged from 17.7 to 19.0 °C over all test vessels, and pH values ranged from 7.5 to 7.9. Oxygen saturation ranged from 93% in the SP and FFT treatments to 97% in the “no food” treatment, which also showed the lowest water chemical parameters (0.2 mg NH4+ per L, 0.11 mg NO2− per L, 7 mg NO3− per L), whereas the SP treatment showed the highest water load (0.9 mg NH4+ per L, 0.37 mg NO2− per L, 8 mg NO3− per L). Mortality rates after 192 h ranged from 4% in the SN treatment to 10% in the SP treatment. In all treatments, filtration activity of the test animals was observed, whereas the animals in the no food treatment showed very low filtration activity. The strongest pollution of the test vessels caused by (pseudo) feces or biofilms appeared in the SP treatment, whereas nearly no pollution was detectable in the no food treatment. Ground stinging nettle was identified as a suitable diet. Compared to the other tested diets, the suspension of ground stinging nettle was leading to less pollution and a low buildup of biofilm and accumulation of organic matter.
Bioaccumulation studies
AgNO3.
Time-weighted average concentrations (TWAs) were calculated for media samples collected at different sampling points. A TWA of 0.684 μg Ag per L was calculated for the treatment with the lower Ag concentration. The TWA of the assay with the higher AgNO3 concentration was calculated to be 7.791 μg Ag per L. Homogenous concentrations were present in the test vessels during the uptake phase (Fig. S1 and S2†).
Animals exposed to the higher Ag concentration showed a soft tissue concentration of 0.24 mg Ag per kg after 24 h of the uptake phase (initial 0.03 mg Ag per kg). The concentration remained stable until the end of the uptake phase (144 h) (Fig. 2A), resulting in a calculated BCFss of 30.5. The soft tissue concentration following exposure to the lower concentration increased from initially 0.03 mg Ag per kg to around 0.5 mg Ag per kg after 72 h of exposure and stayed at this level until the end of the uptake phase after 144 h (Fig. 2B). The body burden decreased to a soft tissue concentration of around 0.25 mg Ag per kg within 72 h of depuration (216 h after study start) and stayed at this level until the end of the depuration phase (144 h/288 h after study start). A BCFss of 710.7 was calculated for the lower concentration. In both treatments, a strong reduction of the bivalve's filtration activity was observed during the uptake phase.
NM 300K.
The total Ag concentrations without differentiation of the particulate or dissolved Ag in the exposure media and soft tissue samples were measured as equivalent to NM 300K. The TWA of the total Ag concentrations in the test media of both treatments were 0.624 and 6.177 μg Ag per L. In both systems homogenous concentrations of Ag were measured in the course of the study (Fig. S3 and S4†).
All bivalves that were exposed to NM 300K during the 96 h uptake phase showed detectable Ag concentrations in their soft tissue. In both treatments a clear increase of the Ag concentration was observed over the duration of the uptake phase, as well as a clear decrease of the body burden during the depuration phases (Fig. 2C and D).
In animals exposed to the lower test concentration treatment the total Ag concentration in the soft tissue increased from initially 0.03 mg Ag per kg (0 h) to 0.8 mg Ag per kg at 24 h and stayed at this level until the end of the uptake phase (96 h, Fig. 2D). During the depuration phase of the lower concentration NM 300K treatment the total Ag concentration in the soft tissue decreased to 0.04 mg Ag per kg within 12 h of depuration and was stable until the end of the depuration phase (Fig. 2D). The calculated BAFss was 128.
During exposure to the higher test concentration the total Ag concentration in the soft tissue increased from initially 0.03 mg Ag per kg (0 h) to around 0.20 mg Ag per kg at 24 h and was stable until end of the uptake phase (96 h, Fig. 2C). Within 12 h of depuration the total Ag concentration decreased to 0.05 mg Ag per kg. The calculated BAFss was 31.
The filtration activities of the bivalves were reduced in both NM 300K treatments during the uptake phase, whereby the filtration activity at the lower test concentration assay was slightly higher than at the higher test concentration.
NM 105.
No differences in the bivalves' filtration activity was observed during the whole bioaccumulation study with NM 105. The presented TiO2 concentrations were calculated from the measured Ti concentrations. The TWA of the total TiO2 concentrations in the exposure media of the two treatments containing NM 105 were 0.099 and 0.589 μg TiO2 per L. During the uptake phase, homogeneous concentrations were given in the test systems (Fig. S5 and S6†). The initial total TiO2 concentration in the bivalves' soft tissue increased during the uptake phases from 0.22 mg TiO2 per kg to a plateau level of around 0.6 mg TiO2 per kg after 24 h of the uptake phase and to around 5.4 mg TiO2 per kg after 96 h of the uptake at the lower and higher concentration treatments, respectively (Fig. 2E and F). Plateau concentrations remained stable until the end of the uptake phase (120 h), resulting in BAFSS values of 6150 (lower concentration) and 9022 (higher concentration). During the following depuration phase the TiO2 body burden of the bivalves decreased rapidly. In the test with the lower concentration, a stable total TiO2 concentration of 0.22 mg TiO2 per kg was reached after 16 h of the depuration phase. A stable TiO2 concentration of 0.16 to 0.17 mg TiO2 per kg was reached after 24 h in the depuration phase of the test with the higher concentration.
During all bioaccumulation tests no notable mortality was observed.
Investigations on tissue distribution
Distribution of total metal concentrations in the soft tissues.
Additional bioaccumulation studies with AgNO3, NM 300K and NM 105 were performed. At the end of the uptake phase, mussels were collected to analyse the distribution of total metal concentrations in the soft tissue. Following exposure to NM 300K and AgNO3, the mantle showed the highest distribution factor (DF) with 1.20 and 1.36, respectively, followed by the viscera (1.09 and 1.04). The muscle tissue showed the lowest distribution factor for total Ag content 0.33 and 0.15 for NM 300K and AgNO3, respectively. In contrast, the highest distribution factor for total TiO2 content (1.45) was estimated for the visceral tissue. The DF values estimated for the other compartments were all lower but in a similar range from 0.40 in the foot tissue and 0.48 in the mantle to 0.50 in the muscle tissue (Fig. 3).
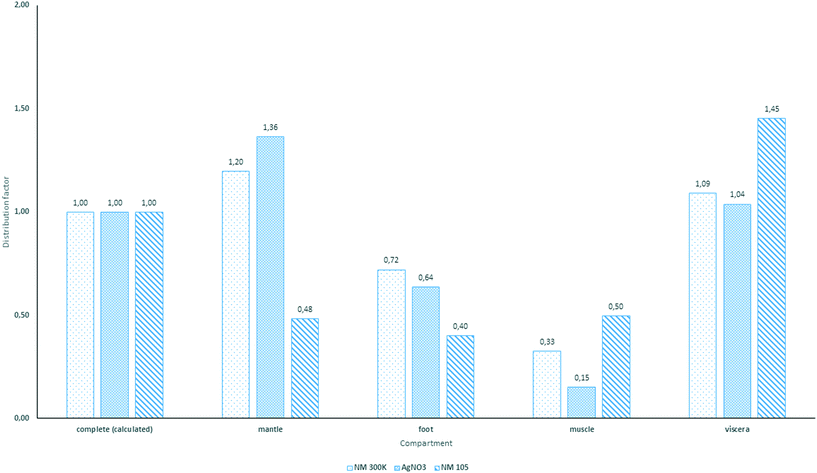 |
| Fig. 3 Distribution factors for total concentration of Ag/TiO2 under steady-state conditions, in relation to the calculated total concentration of Ag and TiO2 in the whole soft body; n = 5. | |
Particle size distribution in the test media.
In order to estimate the particle size distribution in the test media and to check for possible changes/modifications of the applied MNMs, aliquots of the test media were sampled from the test systems prior to the collection of mussels for tissue analysis. Media samples were analysed by spICP-MS. As shown in Fig. 4A–D, no visible changes with regard to the size distribution of the applied MNMs could be observed in the test medium, indicating a high degree of dispersion stability for the time frame of the test and under test conditions. In addition to the MNM dispersions, the stock solution and media of the AgNO3 test were analysed in the spICP-MS mode in order to check for possible precipitates. The results are shown in Fig. 4E and F. It is clearly visible that the “size distribution” obtained from the stock solution differs significantly from the histogram derived from analysis of test media (Fig. 4F). While the stock solution shows results that could be expected for a dissolved analyte, apparently silver nanoparticles are formed in the test medium. That these particles are indeed formed in the test medium can be supported by comparison of the respective transient signals (raw data, see Fig. S7A and B†), where a constant signal as expected for dissolved species was observed for the stock solution and a high number of particle-related spikes could be observed for the medium.
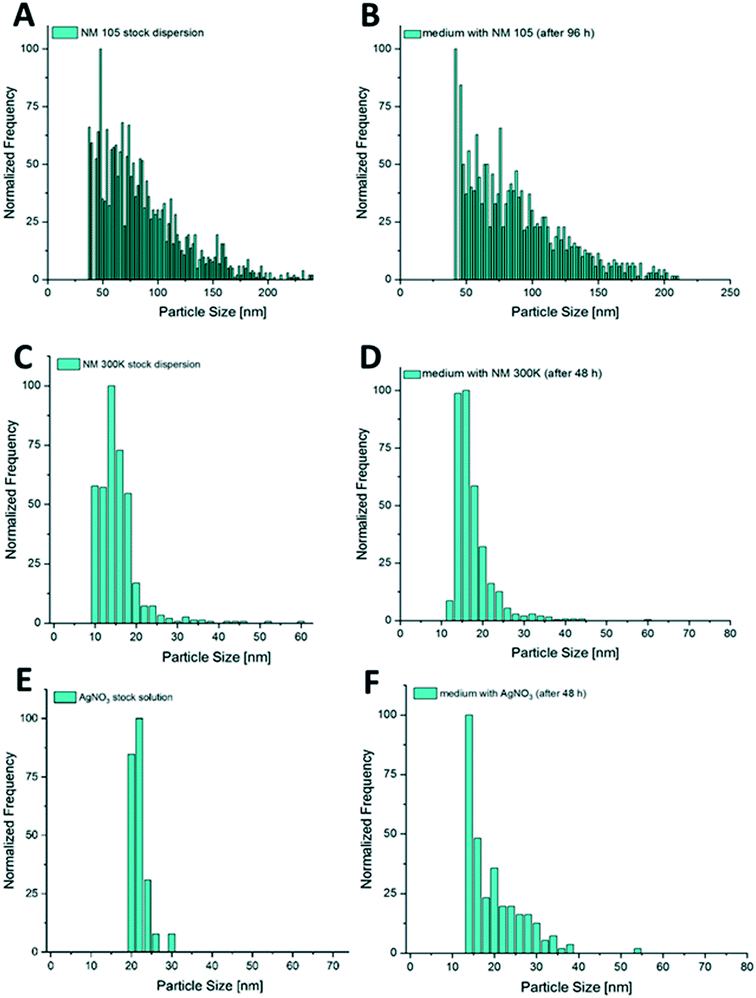 |
| Fig. 4 Comparison of size distribution in media against stock dispersion. (A) NM 105 stock dispersion, (B) NM 105 in test medium at 96 h, (C) NM 300K stock dispersion, (D) NM 300K in test medium at 48 h, (E) AgNO3 stock solution, and (F) AgNO3 in test medium at 48 h. | |
However, a few spikes are also still visible in the raw data for the stock solution and are interpreted as particles which together with the background signal form the size distribution shown in Fig. 4E. At this point, it is important to emphasize that the histograms shown use normalized scales and cannot be directly compared.
Validation of tissue digestion procedure.
Mussel tissue had to be processed prior to analysis by spICP-MS. The impact of the digestion procedure on the size distribution of the nanoparticles was evaluated qualitatively. Mussel tissue was spiked with NM 300K and NM 105 prior to digestion and the measured size distribution was compared with the aqueous stock dispersion. Additionally, hydrolysates of mussels without prior exposure to the MNM were spiked after digestion with NM 300K in order to evaluate the impact of the resulting solution on the size distribution. No visible impact of the tissue hydrolysate (Fig. 5C), as well as the digestion procedure (Fig. 5B) on the size distribution was observed. Similar observations were made for the size distributions of particles extracted from the soft tissue of mussels which were exposed to NM 300K over an extended period of 48 h and 120 h (Fig. 7).
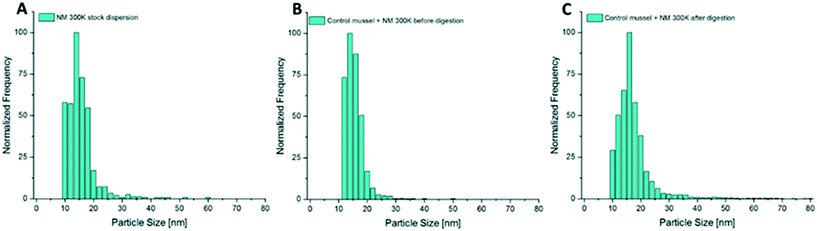 |
| Fig. 5 Particle size distributions of NM 300K, AgNPs determined by spICP-MS analysis. (A): Analysis of stock dispersion in UHQ-water, (B) analysis of digested mussel tissue with NM 300K spiked prior to digestion, and (C) analysis of digested mussel tissue with NM 300K spiked after digestion of the tissue. | |
The results of the respective experiment with NM 105 are shown in Fig. 6. For both hydrolysates spiked prior and after digestion a shift towards smaller sizes was observed. The effect was more pronounced for the mussel already spiked prior to the digestion.
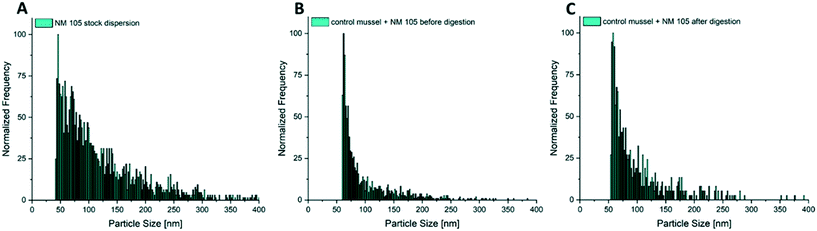 |
| Fig. 6 Particle size distributions of NM 105, TiO2NPs determined by spICP-MS analysis. (A) Analysis of stock dispersion in UHQ-water, (B) analysis of digested mussel tissue with NM 105 spiked prior to digestion, and (C) analysis of digested mussel tissue with NM 105 spiked after digestion of the tissue. | |
Fig. 8 shows the size distributions of NM 105 in mussel tissue extracts after different exposure times compared to the size distribution of NM 105 in the surrounding test medium. In contrast to the observations made for NM 300K (Fig. 5A and 7A), the distribution of NM 105 in mussel tissue extracts showed a loss of bigger particles compared to the size distribution of the stock dispersion and the test medium (Fig. 8A and B). Median particle size of the TiO2NPs measured in the soft tissue was in the range of 42 to 49 nm, whereas the median particle size in the exposure media was measured to be in the range of 65 to 83 nm. The shift is more pronounced than observed for the digestion validation samples (Fig. 6). No difference was observed regarding the size distributions of NM 105 in extracts of mussel tissue collected after different exposure times.
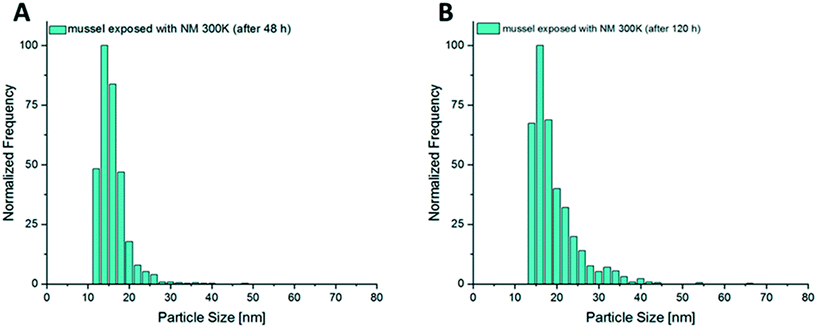 |
| Fig. 7 (A) Size distribution of AgNPs from digested mussel tissue after 48 h of exposure and (B) size distribution of NM 300K in mussel tissue after 120 h of exposure time. | |
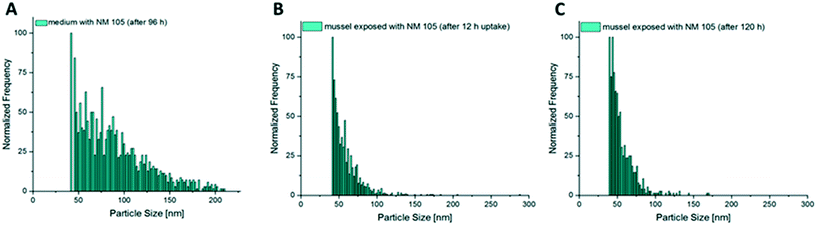 |
| Fig. 8 Size distributions of NM 105 in (A) test medium, (B) tissue after 12 h, and (C) tissue after 120 h. | |
Particle concentrations and distribution in the soft tissue
During the additional bioaccumulation study with AgNO3, no significant increase of the measured Ag particles was observed during the 48 h uptake phase (Fig. 9A). The measured concentration of the presumed Ag particles in the different compartments was 5 times higher in the viscera when compared to the other compartments.
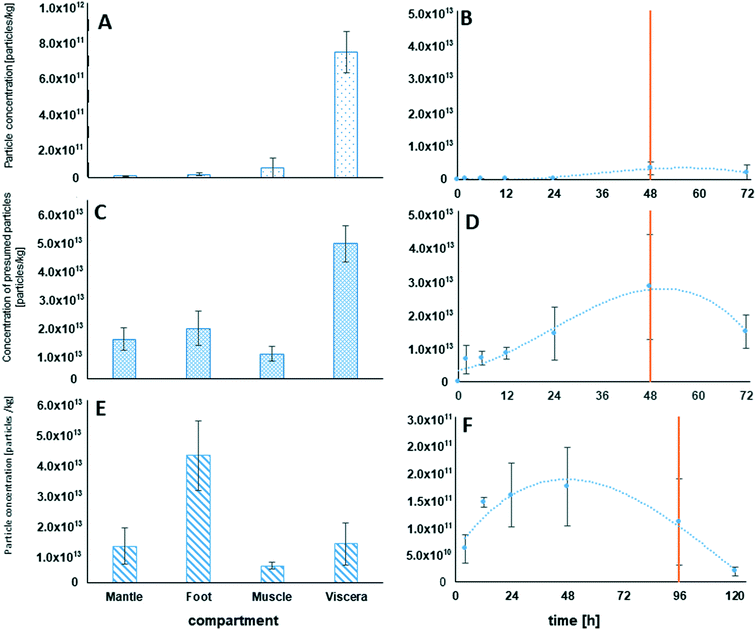 |
| Fig. 9 Particle concentrations and distributions in the soft tissue of C. fluminea. (A) Concentration of presumed particles in different compartments at steady-state conditions (48 h) and in the whole soft body during the uptake phase and in the depuration time of the AgNO3 treatment (B), (C) NM 300K particle concentration in different compartments at steady state conditions (48 h) and in the whole soft body during the uptake phase and in the depuration time (D), (E) NM 105 particle concentration in different compartments at steady state conditions (96 h) and in the whole soft body during the uptake phase and in the depuration time (F). | |
For NM 300K exposed animals, a clear increase of particle concentrations could be measured in the whole soft tissue of the test animals during the 48 h uptake phase. A trend of decreasing particle concentration in the soft tissue during the following depuration period lasting 24 h was observed (Fig. 9D). The determined median particle size of the AgNPs in the tissue was 14 nm, whereas the determined median particle size in the exposure media was 15 nm.
The tissue of the viscera showed the highest single particle concentration of all measured compartments, sampled after 48 h of exposure. Particle concentrations in the other compartments were significantly lower and not significantly different (Fig. 9C).
Also, in the additional bioaccumulation study with NM 105, an increase in the particle concentration was measured in the soft tissue of the test animals during the uptake period (Fig. 9F). A stable particle concentration was reached after 12 h of exposure and remained stable until the end of the uptake phase (96 h). Concentrations decreased significantly during the following 24 h of depuration (Fig. 9F). Median particle size of TiO2 MNMs measured in the soft tissue was in the range of 42 to 49 nm, whereas the median particle size in the exposure media was measured to be in a range of 65 to 83 nm. The highest measured particle concentration of the compartment samples after 96 h of exposure was determined in foot tissue, whereas the particle concentrations in the mantle, muscle and viscera were up to four times lower and showed no significant differences (Fig. 9E).
Discussion
Bioaccumulation studies with MNMs are difficult to carry out due to their tendency to agglomerate and deposit in sediments. The bioaccumulation studies with the freshwater bivalve C. fluminea demonstrated the suitability of the new test system which meets the need to investigate bioaccumulation in filtering/sediment living organisms as organisms of special relevance for environmental risk assessment of MNMs. During all flow-through studies a continuous exposure of the bivalves with stable MNM concentrations could be achieved. Water quality parameters measured within the test system (dissolved oxygen, pH, ammonia, nitrate/nitrite) were constantly maintained in an acceptable range and provided optimal experimental conditions for the performance of the bioaccumulation studies. An experimental diet was required which ensures a sufficient nutrient supply during the extended experimental periods. Ground stinging nettle was identified as a suitable diet being rich in elements and minerals (e.g. ferric oxide, potassium, calcium, and silicium)74 which are essential for the shell formation of bivalves. It is hypothesized that the small particles of the ground material were triggering the filtration activity of the mussels. However, the amount of food supplied to the test system should be generally reduced as far as possible to avoid sorption processes of the MNMs to the food while being high enough to allow sufficient nutrient uptake during the bioaccumulation studies.
Two MNMs were chosen based on notable differences in their major characteristics and tested for their bioaccumulation potential. The silver nanoparticle NM 300K was tested as representative of MNMs that disperse well and release ions. In contrast the TiO2 nanomaterial NM 105 is nearly chemically inert and is one of the most commonly used MNMs56 representative of non-ion-releasing and condition-dependent sedimenting MNMs. AgNO3 was used as an additional test item representing the same element as NM 300K but in a non-nanoparticulate form, allowing the possibility of comparing the bioavailability, accumulation and fate of dissolved and particulate Ag.
During AgNO3 exposure the bioaccumulation of Ag resulted from the uptake of dissolved Ag+ from the exposure media. Thus, the estimated endpoint was the BCFss and not BAFss. But the measurements using spICP-MS showed the presence of presumed particles that could be resulting from precipitation processes of Ag+, e.g. Ag2S, AgCl, or Ag2CO3. Due to the non-differentiable uptake of Ag in the case of the AgNO3 exposure, the BAF should be used for bioaccumulation assessment and not the BCF. With a BAFss of 30.5, a significantly lower bioaccumulation of silver was observed following exposure to the higher test concentration compared to a BAFss of 710.7 for the lower concentration AgNO3 treatment. This might be explained by reduced filtration activities of the mussels in response to increased metal concentrations which has been previously observed in bivalves and which were considered as a protection mechanism to avoid adverse effects by metal accumulation: for instance, a slight decrease of the filtration rate was observed in response to increased metal exposure, e.g. for the green mussel Perna viridis,75 the zebra mussel Dreissena polymorpha76 and C. fluminea.77,78 A complete bivalve closing behavior in response to higher heavy metal concentrations in the water was described as a strategy to avoid toxic conditions.79–84 Reduced filtration activities were also observed in this study during exposure to AgNO3, however in varying intensities. Due to the visibly lower reduction of the filtration rate in the lower concentration AgNO3 treatment, the continuous uptake of small amounts of Ag+ may have led to the higher BAFss value compared to that in the higher test concentration. Deviation in filtration activities between both treatments may also explain the differences in the time required to reach steady-state Ag concentrations in the soft tissue of C. fluminea. Therefore, the protective behaviour of bivalves needs to be considered in bioaccumulation studies of ionic or ion-releasing and other acute toxic substances as the reduction in filtration activity may lead to totally different results depending on the level of the concentrations used.
Pretests should be carried out to elucidate the fate of the test item in the test system and to estimate a suitable exposure concentration to avoid protective behavior as described for AgNO3. At least two test concentrations should be applied to identify potential concentration-dependent effects.
During bioaccumulation studies with NM 300K, a visibly lower inhibition of the filtration activity was observed compared to the study with AgNO3 at similar total Ag concentration levels. Due to this observation it is safe to assume that the protection behavior is mainly triggered by free Ag+. The amount of dissolved Ag in the NM 300K test media was estimated in pretests to be between 1.6% to 21.5% within 24 h after production of the test media under static conditions. Considering the flow-through conditions applied in the bioaccumulation studies with NM 300K, the percentage of dissolved Ag+ may have been even on a lower level but still sufficient to induce a weak valve closing behavior. Consequently, the potentially higher release of Ag+ in the higher concentration NM 300K treatment compared to the lower concentration treatment has obviously led to a reduced filtration activity leading to different BAFss values of 31 and 128, respectively. During the depuration phase, a rapid and nearly complete elimination of Ag was observed which indicates that total Ag concentrations measured in the bivalve samples were supposed to be mainly represented by the particulate fraction. The ingested AgNPs obviously simply moved through the digestive tract. This was confirmed by the additional investigations using spICP-MS. Particle concentrations measured during the uptake and elimination phase in the soft tissue of the animals followed the total Ag concentration measured in the soft tissue during the bioaccumulation study. The majority of the particles were found in the viscera, including the gills and the digestive tract. All other compartments showed very low particle concentrations. This clearly indicates that there was no significant transport of AgNPs through the different compartment tissues or hemolymph and is thus pointing to the negligible bioavailability of AgNPs to C. fluminea. It can be only speculated as to which extent dissolved Ag+ from the test material (NM 300K) was bio concentrated by the test organisms and contributed to the total Ag concentrations measured throughout the study. However, the distribution factors for accumulated Ag in the different compartments underpin the presumption that only Ag+, even present at low concentrations, was really incorporated in the mussel tissue. Both exposure scenarios, AgNO3 and NM 300K, led to comparable distribution factors for total silver (Fig. 3). Further investigations are required to elucidate the incorporation of AgNPs into the different compartments.
However, the results also show the limits of the analytical methods. For NM 300K there is a significant overlap with the background signals due to the small size of the material in the range of the instrumental size detection limit (∼10 nm for Ag, given by the Agilent MassHunter Software). In addition the known presence of even smaller particles in the range of 5 nm and thus the resulting size distributions have to be treated carefully with respect to the lower sizes.85 This significant overlap can be highlighted if the size distributions are compared with the distribution derived from the complete signal distribution, without application of a particle detection threshold (for the size distributions shown in Fig. S8B†). From Fig. S8B† it is clear that for NM 300K there is no clear gap between the particle-related signals and the background due to the small size of the material.
This observations are also well in line with reported particle detection limits (e.g. 16–20 nm in Lee et al.86).
The comparison of histograms derived from measurements of dissolved Ag (e.g.Fig. 4E) show similar distributions highlighting the significant overlap with the background. However, for the normalized histograms established for AgNO3 stock solution it has to be noted that there were in fact nearly no particles in the sample and the derived “size distribution” was mainly based on much lower numbers of signal spikes than for the NM 300K-containing samples. Thus, if this silver nanomaterial has to be tested in the future it is recommended to either establish a procedure for removal of the dissolved background (e.g. by ion exchange resins, ultrafiltration or centrifugation) or measuring at higher time resolution (e.g. with shorter dwell times).87
Particle specific analysis of test media for AgNO3 tests revealed the presence of nanosized silver containing particles that were not observed for the stock solution and are thus formed in the medium by precipitation.
The increase in the total Ti tissue concentration during the bioaccumulation studies can be explained by the uptake and potential accumulation of MNMs from the media. This was confirmed by single particle measurements in the mussel tissue showing a progression during the uptake and depuration phase that mirrored the course of the total Ti concentrations in the soft tissue. However, the measured concentrations were mainly caused by particles localized in the digestive tract and in the viscera as shown by the distribution factors for TiO2 with the viscera showing the highest distribution factor.
The analysis of particle size distributions in test media and mussel tissue by spICP-MS following enzymatic digestion was shown to be suitable for NM 105 TiO2 particles. The particles have shown a broad size distribution clearly above the background signal. Even though there was a shift towards smaller particle sizes observed for the digestion procedure by analysis of spiked mussels, the size distributions measured for animals exposed in the test system, where the uptake was due to filtration, showed a more pronounced loss of bigger particles, indicating exclusion of these particles by the filtration mechanism. This finding also highlights the necessity of particle specific analysis methods, which could provide more insights into the relevant processes compared to standard total concentration analysis.
As for NM 300K, it is debatable whether the particles were only ingested or really incorporated into the tissue. However, the very effective and fast elimination that was observed during the depuration phase of both tested concentrations with TiO2 points to the assumption that the MNMs were only ingested. Our observations are in accordance with the results of the work of Doyle et al. (2015),88 where an elimination of more than 90% of TiO2NPs previously ingested from Mytilus edulis within 12 h was observed.
The increased BAFss values (6150 and 9022) estimated for TiO2NPs compared to NM 300K may be explained by two factors: first, by a potentially high filtration activity during the uptake phase, triggered by particulate matter. This effect should be stronger for NM 105 that were measured to be in the range of 63 to 83 nm in the exposure media, while particles of NM 300K were clearly smaller (15 to 17 nm). In addition, NM 105 shows a high tendency to agglomerate which may lead to a more effective uptake. It was shown that filter-feeding mussels take up bigger and agglomerated NMNs much more effectively than smaller und free particles.28,89 Second, the higher BAFss values for the TiO2NPs may be explained by the lack of dissolved toxic ions in the test media that could trigger a protective mechanism as assumed for the Ag tests.
The bioaccumulation studies with the freshwater bivalve C. fluminea demonstrated the feasibility of the new test system for testing MNMs in filtering organisms. During all studies, a continuous exposure with stable MNM concentrations was achieved. The elucidation of bioavailability, uptake and elimination as well as accumulation of the test items was possible on the level of total and particle concentrations for the whole soft body as well as the single tissue compartments. By this, the fate of MNMs within the body or different tissues could be further clarified. However, methods like transmission electron microscopy are required for the additional complementary evidence that MNMs are really incorporated into the tissue or penetrated into cells. Nevertheless, the results obtained with the described test system can be used to generate useful endpoints required for regulatory processes and could be included in a tiered bioaccumulation testing strategy for MNMs.16 Even if some MNMs are not really bioaccumulated, the BAFss obtained provides a valuable indication of the ingestion of MNMs by bivalves, if combined with information on the elimination rate estimated following the ingestion of MNMs. A fast elimination (time back to start concentration ≤24 h) points to the ingestion but no incorporation of MNMs in the animal tissues. A slow elimination (time back to start concentration >24 h) provides clear indications of the incorporation of the MNMs. However, it cannot be excluded that the bioaccumulation occurred by incorporation of dissolved/ionic fractions or particulate matter which would require further elucidations using microscopy methods. Suitable criteria for the regulatory assessment of bioaccumulation based on BAF estimates need to be derived and verified.
Comparison of bioaccumulation of the nano forms with freely dissolved ions/forms of some elements or compounds is not possible if the ionic form tends to precipitate in the presence of ubiquitous elements or compounds like in the case of Ag and S. The same applies for elements with strong binding affinities to organic matter like proteins or humic acids.
Because bivalves and other filtering benthic organisms represent the main part of the biomass in freshwater systems, the benthic food chain is supposed to play a central role regarding the ecological impact of MNMs.26,37,38,90 The steady-state concentration represents the maximum loading capacity of MNMs taken up from the surrounding medium by bivalves. Due to the high filtration rate of the bivalves the loading capacity for MNMs compared to the surrounding medium concentration may lead to an increased risk of secondary poisoning of predatory species even if no real bioaccumulation occurs. This was shown in the studies on TiO2 where high body burden but no incorporation of the test material was observed. The results of the Bivalvia bioaccumulation test may thus provide important information regarding the transfer of MNMs into the aquatic food chain via predators or benthic invertebrates that feed on bivalve feces and/or pseudo-feces.26,27,37–40 The high filtration rate of bivalves may cause feces/pseudo-feces with high concentrations of MNMs. Further investigations are required to elucidate the uptake of highly contaminated feces or pseudo-feces by benthic invertebrates that feed on fecal matter.
The suitability of the new test system for bioaccumulation studies with freshwater bivalves has been demonstrated in this study. The use of marine bivalves for bioaccumulation studies is described in two guidance documents of the American Society for Testing and Materials91 and US EPA.92 However, the systems described are assumed to be unsuitable for a constant exposure of MNMs. The new test system may thus also represent a potential alternative for testing MNMs in marine bivalves, however, specific media adjustments would be required. In addition to the bioaccumulation assessment of MNMs, the test system may also be suitable to investigate the uptake/bioaccumulation of microplastic in bivalves.93–95
Conclusions
The bioaccumulation studies with the freshwater bivalve C. fluminea demonstrated the suitability of the new test system. During all studies a continuous exposure with stable MNM concentrations was achieved. The elucidation of bioavailability, uptake and elimination as well as accumulation of the test items was possible on the level of total and particle concentrations for the whole soft body as well as the single tissue compartments. In this way, the fate of MNMs within the body or different tissues could be further elucidated. However, methods like transmission electron microscopy are required as proof that MNMs are really incorporated into the tissue or penetrated into cells. The results obtained with this test system can be used to generate useful endpoints required for regulatory purposes and could be included in a tiered bioaccumulation testing strategy for MNMs.
List of abbreviations
Ag+ | Silver(I) ion |
AgNPs | Silver nanoparticles |
ANOVA | Analysis of variance |
BAFss | Bioaccumulation factor estimated at steady state |
BCFss | Bioconcentration factor estimated at steady state |
DF | Distribution factor |
FFT | Fish food tablet treatment in the dietary test |
ICP-MS | Inductively coupled plasma mass spectrometry |
MNM | Manufactured nanomaterial |
SN | Stinging nettle treatment in the dietary test |
SP |
Spirulina species treatment in the dietary test |
spICP | Single-particle inductively coupled plasma mass spectrometry |
TiO2NP | Titanium dioxide nanoparticle |
TWA | Time-weighted average (concentration) |
UHQ-water | Ultra high quality water |
Conflicts of interest
The authors declare no conflicts of interest.
Acknowledgements
This work was financially supported by the German Federal Ministry for the Environment, Nature Conservation and Nuclear Safety within the ReFoPlan Project FKZ 3716 66 410 0 which was supervised by the German Environment Agency and the Fraunhofer Institute for Molecular Biology and Applied Ecology IME. The opinions and views expressed in the present study do not necessarily reflect those of the German Federal Environment Agency. Special thanks to Markus Pojda, Andreas Rickert and Martin Baumann for the installation of the test system and to Laura Föckeler, Kevin Ladage and Virginia Schraps for the support during the sample processing and measurements of total concentrations as well as to Dana Esser and Anna Schauerte for their technical support in conducting the bioaccumulation studies. We thank Dr. Ralf Kägi for performing EDX measurements of the applied nanomaterials and for providing TEM/SEM images.
References
-
European Commission, Communication from the commission to the European Parliament, the Council and the European Economic and Social Committee, 2012.
- T. M. Benn and P. Westerhoff, Nanoparticle Silver Released into Water from Commercially Available Sock Fabrics, Environ. Sci. Technol., 2008, 42, 4133–4139 CrossRef CAS PubMed.
- N. C. Mueller and B. Nowack, Exposure Modeling of Engineered Nanoparticles in the Environment, Environ. Sci. Technol., 2008, 42, 4447–4453 CrossRef CAS PubMed.
- F. Gottschalk and B. Nowack, The release of engineered nanomaterials to the environment, J. Environ. Monit., 2011, 13, 1145 RSC.
- C. Levard, E. M. Hotze, G. V. Lowry and G. E. Brown, Environmental Transformations of Silver Nanoparticles: Impact on Stability and Toxicity, Environ. Sci. Technol., 2012, 46, 6900–6914 CrossRef CAS PubMed.
-
European Parliament Council, Regulation (EC) No 1907/2006 of the European Parliament and of the Council of 18 December 2006 concerning the Registration, Evaluation, Authorisation and Restriction of Chemicals (REACH), establishing a European Chemicals Agency, amending Directive 1999/45/EC and repealing Council Regulation (EEC) No 793/93 and Commission Regulation (EC) No 1488/94 as well as Council Directive 76/769/EEC and Commission Directives 91/155/EEC, 93/67/EEC, 93/105/EC and 2000/21/EC, Off. J Eu.
- Y. Naiki, Assessing Policy Reach: Japan's Chemical Policy Reform in Response to the EU's REACH Regulation, J. Environ. Law, 2010, 22, 171–195 CrossRef.
-
USEPA, High Production Volume Challenge Program High Production Volume Challenge Program, 2004.
-
Korea Ministry of Government Legislation, Korean Laws in English - Toxic Chemicals Control Act, http://www.moleg.go.kr/english/korLawEng?pstSeq=47535, (accessed 4 January 2018).
-
Ministry of Environment and Urbanization(MoEU) of Turkey, Draft by-law on registration, evaluation, authorization and restriction of chemicals.
-
ECHA, Guidance on Information Requirements and Chemical Safety Assessment Chapter R.11: PBT/vPvB assessment, Eur. Chem. Agency, 2017, 494.
- Organisation for Economic Co-operation and Develpement (OECD), Test No. 305: Bioaccumulation in fish: aqueous and dietary exposure, OECD Guidel. Test. Chem. Paris.
- W. de Wolf, M. Comber, P. Douben, S. Gimeno, M. Holt, M. Léonard, A. Lillicrap, D. Sijm, R. van Egmond, A. Weisbrod and G. Whale, Animal use replacement, reduction, and refinement: development of an integrated testing strategy for bioconcentration of chemicals in fish, Integr. Environ. Assess. Manage., 2007, 3, 3–17 CrossRef CAS.
- K. Aschberger, C. Micheletti, B. Sokull-Klüttgen and F. M. Christensen, Analysis of currently available data for characterising the risk of engineered nanomaterials to the environment and human health — Lessons learned from four case studies, Environ. Int., 2011, 37, 1143–1156 CrossRef CAS PubMed.
-
S. M. Hankin, S. A. K. Peters, C. A. Poland, S. Foss Hansen, J. Holmqvist, B. L. Ross, J. Varet and R. J. Aitken, FINAL Specific Advice on Fulfilling Information Requirements for Nanomaterials under REACH (RIP-oN 2) - Final Project Report, 2011.
- R. D. Handy, J. Ahtiainen, J. M. Navas, G. Goss, E. A. J. Bleeker and F. von der Kammer, Proposal for a tiered dietary bioaccumulation testing strategy for engineered nanomaterials using fish, Environ. Sci.: Nano, 2018, 5, 2030–2046 RSC.
-
ECHA, Guidance on Information Requirements and Chemical Safety Assessment Chapter R.7a: Endpoint specific guidance, Eur. Chem. Agency, DOI:10.2823/337352.
-
American Society for Testing and Materials, ASTM E1022 - 94 Standard Guide for Conducting Bioconcentration Tests with Fishes and Saltwater Bivalve Mollusks, 2013 Search PubMed.
- F. Regoli and E. Orlando, Accumulation and subcellular distribution of metals (Cu, Fe, Mn, Pb and Zn) in the Mediterranean mussel Mytilus galloprovincialis during a field transplant experiment, Mar. Pollut. Bull., 1994, 28, 592–600 CrossRef CAS.
- C. Cossu, A. Doyotte, M. C. Jacquin, M. Babut, A. Exinger and P. Vasseur, Glutathione Reductase, Selenium-Dependent Glutathione Peroxidase, Glutathione Levels, and Lipid Peroxidation in Freshwater Bivalves, Unio tumidus, as Biomarkers of Aquatic Contamination in Field Studies, Ecotoxicol. Environ. Saf., 1997, 38, 122–131 CrossRef CAS PubMed.
- A. Doyotte, C. Cossu, M.-C. Jacquin, M. Babut and P. Vasseur, Antioxidant enzymes, glutathione and lipid peroxidation as relevant biomarkers of experimental or field exposure in the gills and the digestive gland of the freshwater bivalve Unio tumidus, Aquat. Toxicol., 1997, 39, 93–110 CrossRef CAS.
- M.-L. Vidal, A. Bassères and J.-F. Narbonne, Potential biomarkers of trichloroethylene and toluene exposure in Corbicula fluminea, Environ. Toxicol. Pharmacol., 2001, 9, 87–97 CrossRef CAS PubMed.
- F. Geret, A. Serafim and M. J. Bebianno, Antioxidant Enzyme Activities, Metallothioneins and Lipid Peroxidation as Biomarkers in Ruditapes decussatus?, Ecotoxicology, 2003, 12, 417–426 CrossRef CAS PubMed.
- J. F. Narbonne, J. E. Djomo, D. Ribera, V. Ferrier and P. Garrigues, Accumulation Kinetics of Polycyclic Aromatic Hydrocarbons Adsorbed to Sediment by the MolluskCorbicula fluminea, Ecotoxicol. Environ. Saf., 1999, 42, 1–8 CrossRef CAS PubMed.
- A. Legeay, M. Achard-Joris, M. Baudrimont, J.-C. Massabuau and J.-P. Bourdineaud, Impact of cadmium contamination and oxygenation levels on biochemical responses in the Asiatic clam Corbicula fluminea, Aquat. Toxicol., 2005, 74, 242–253 CrossRef CAS PubMed.
- R. Gergs, K. Rinke and K.-O. Rothhaupt, Zebra mussels mediate benthic–pelagic coupling by biodeposition and changing detrital stoichiometry, Freshwater Biol., 2009, 54, 1379–1391 CrossRef.
- T. Basen, R. Gergs, K.-O. Rothhaupt and D. Martin-Creuzburg, Phytoplankton food quality effects on gammarids: benthic–pelagic coupling mediated by an invasive freshwater clam, Can. J. Fish. Aquat. Sci., 2012, 70, 198–207 CrossRef.
- M. S. Hull, P. Chaurand, J. Rose, M. Auffan, J.-Y. Bottero, J. C. Jones, I. R. Schultz and P. J. Vikesland, Filter-Feeding Bivalves Store and Biodeposit Colloidally Stable Gold Nanoparticles, Environ. Sci. Technol., 2011, 45, 6592–6599 CrossRef CAS PubMed.
- M. N. Moore, Do nanoparticles present ecotoxicological risks for the health of the aquatic environment?, Environ. Int., 2006, 32, 967–976 CrossRef CAS PubMed.
- A. Baun, N. B. Hartmann, K. Grieger and K. O. Kusk, Ecotoxicity of engineered nanoparticles to aquatic invertebrates: a brief review and recommendations for future toxicity testing, Ecotoxicology, 2008, 17, 387–395 CrossRef CAS PubMed.
- R. J. Griffitt, J. Luo, J. Ao, J. C. Bonzongo and D. S. Barber, Effects of particle composition and species on toxicity of metallic nanomaterials in aquatic organisms, Environ. Toxicol. Chem., 2008, 27, 1972–1978 CrossRef CAS PubMed.
- S. Tedesco, H. Doyle, J. Blasco, G. Redmond and D. Sheehan, Exposure of the blue mussel, Mytilus edulis, to gold nanoparticles and the pro-oxidant menadione, Comp. Biochem. Physiol., Part C: Toxicol. Pharmacol., 2010, 151, 167–174 Search PubMed.
- T. J. Baker, C. R. Tyler and T. S. Galloway, Impacts of metal and metal oxide nanoparticles on marine organisms, Environ. Pollut., 2014, 186, 257–271 CrossRef CAS PubMed.
- D. Kühnel and C. Nickel, The OECD expert meeting on ecotoxicology and environmental fate — Towards the development of improved OECD guidelines for the testing of nanomaterials, Sci. Total Environ., 2014, 472, 347–353 CrossRef PubMed.
- J. L. Ferry, P. Craig, C. Hexel, P. Sisco, R. Frey, P. L. Pennington, M. H. Fulton, I. G. Scott, A. W. Decho, S. Kashiwada, C. J. Murphy and T. J. Shaw, Transfer of gold nanoparticles from the water column to the estuarine food web, Nat. Nanotechnol., 2009, 4, 441–444 CrossRef CAS PubMed.
- D. Cleveland, S. E. Long, P. L. Pennington, E. Cooper, M. H. Fulton, G. I. Scott, T. Brewer, J. Davis, E. J. Petersen and L. Wood, Pilot estuarine mesocosm study on the environmental fate of Silver nanomaterials leached from consumer products, Sci. Total Environ., 2012, 421–422, 267–272 CrossRef CAS PubMed.
- A. Y. Karatayev, L. E. Burlakova and D. K. Padilla, The effects of Dreissena polymorpha (Pallas) invasion on aquatic communities in eastern Europe, J. Shellfish Res., 1997, 16, 187–203 Search PubMed.
- H. A. Roditi, D. L. Strayer and S. E. G. Findlay, Characteristics of zebra mussel
(Dreissena polymorpha) biodeposits in a tidal freshwater estuary, Arch. Hydrobiol., 1997, 140, 207–219 CrossRef CAS.
- C. M. Custer and T. W. Custer, J. Ornithol., 1996, 67, 86–99 Search PubMed.
- E. H. van Nes, R. Noordhuis, E. H. H. R. Lammens, R. Portielje, B. Reeze and E. T. H. M. Peeters, Modelling the effects of diving ducks on zebra mussels Dreissena polymorpha in lakes, Ecol. Modell., 2008, 211, 481–490 CrossRef.
- H. L. Phelps, The Asiatic Clam (Corbicula fluminea) Invasion and System-Level Ecological Change in the Potomac River Estuary near Washington, D. C, Estuaries, 1994, 17, 614 CrossRef.
- S. Rajagopal, G. van der Gelde and A. bij de Vaate, Reproductive biology of the Asiatic clams Corbicula fluminalis and Corbicula fluminea in the river Rhine, Fundam. Appl. Limnol., 2000, 149, 403–420 CrossRef.
- G. Darrigran, Potential Impact of Filter-feeding Invaders on Temperate Inland Freshwater Environments, Biol. Invasions, 2002, 4, 145–156 CrossRef.
-
A. Y. Karatayev, R. G. Howells, L. E. Burlakova and B. D. Sewell, History of spread and current distribution of Corbicula fluminea (müller) in texas, DOI:10.2983/0730-8000(2005)24[553:hosacd]2.0.co;2.
- R. Sousa, L. Guilhermino and C. Antunes, Molluscan fauna in the freshwater tidal area of the River Minho estuary, NW of Iberian Peninsula, Ann. Limnol. - Int. J. Lim., 2005, 41, 141–147 CrossRef.
-
C. L. Klein, B. Stahlmecke, J. Romazanov, T. A. J. Kuhlbusch, E. Van Doren, P.-J. De Temmerman, J. Mast, P. Wick, H. Krug, G. Locoro, K. Hund-Rinke, W. Kördel, S. Friedrichs, G. Maier, J. Werner, T. Linsinger, B. M. Gawlik, S. Comero, Institute for Health and Consumer Protection, European Commission, Joint Research Centre, Institute for Environment and Sustainability and Institute for Reference Materials and Measurements, NM-Series of representative manufactured nanomaterials: NM-300 Silver Characterisation, Stability, Homogeneity, Publications Office, 2011.
-
K. Rasmussen, J. Mast, P.-J. De Tammermann, E. Verleysen, N. Waegneers, F. Van Steen, J. Pizzolon and Institute for Health and Consumer Protection, Titanium dioxide, NM-100, NM-101, NM-102, NM-103, NM-104, NM-105 characterisation and physico-chemical properties, Publications Office of the European Union, 2014.
- J. Fabrega, S. N. Luoma, C. R. Tyler, T. S. Galloway and J. R. Lead, Silver nanoparticles: Behaviour and effects in the aquatic environment, Environ. Int., 2011, 37, 517–531 CrossRef CAS PubMed.
- G. Vale, K. Mehennaoui, S. Cambier, G. Libralato, S. Jomini and R. F. Domingos, Manufactured nanoparticles in the aquatic environment-biochemical responses on freshwater organisms: A critical overview, Aquat. Toxicol., 2016, 170, 162–174 CrossRef CAS PubMed.
- A. J. Bone, B. P. Colman, A. P. Gondikas, K. M. Newton, K. H. Harrold, R. M. Cory, J. M. Unrine, S. J. Klaine, C. W. Matson and R. T. Di Giulio, Biotic and Abiotic Interactions in Aquatic Microcosms Determine Fate and Toxicity of Ag Nanoparticles: Part 2–Toxicity and Ag Speciation, Environ. Sci. Technol., 2012, 46, 6925–6933 CrossRef CAS PubMed.
- E. McGillicuddy, I. Murray, S. Kavanagh, L. Morrison, A. Fogarty, M. Cormican, P. Dockery, M. Prendergast, N. Rowan and D. Morris, Silver nanoparticles in the environment: Sources, detection and ecotoxicology, Sci. Total Environ., 2017, 575, 231–246 CrossRef CAS PubMed.
- C. Zhang, Z. Hu and B. Deng, Silver nanoparticles in aquatic environments: Physiochemical behavior and antimicrobial mechanisms, Water Res., 2016, 88, 403–427 CrossRef CAS.
- P. D. Bragg and D. J. Rainnie, The effect of silver ions on the respiratory chain of Escherichia coli, Can. J. Microbiol., 1974, 20, 883–889 CrossRef CAS.
- Q. L. Feng, J. Wu, G. Q. Chen, F. Z. Cui, T. N. Kim and J. O. Kim, A mechanistic study of the antibacterial effect of silver ions on Escherichia coli and Staphylococcus aureus, J. Biomed. Mater. Res., 2000, 52, 662–668 CrossRef CAS PubMed.
- W. J. Schreurs and H. Rosenberg, Effect of silver ions on transport and retention of phosphate by Escherichia coli, J. Bacteriol., 1982, 152, 7–13 CAS.
- F. Piccinno, F. Gottschalk, S. Seeger and B. Nowack, Industrial production quantities and uses of ten engineered nanomaterials in Europe and the world, J. Nanopart. Res., 2012, 14, 1109 CrossRef.
- F. Abdolahpur Monikh, A. Praetorius, A. Schmid, P. Kozin, B. Meisterjahn, E. Makarova, T. Hofmann and F. von der Kammer, Scientific rationale for the development of an OECD test guideline on engineered nanomaterial stability, NanoImpact, 2018, 11, 42–50 CrossRef.
- G. Vale, C. Franco, M. S. Diniz, M. M. C. dos Santos and R. F. Domingos, Bioavailability of cadmium and biochemical responses on the freshwater bivalve Corbicula fluminea – the role of TiO2 nanoparticles, Ecotoxicol. Environ. Saf., 2014, 109, 161–168 CrossRef CAS.
- T. Balbi, A. Smerilli, R. Fabbri, C. Ciacci, M. Montagna, E. Grasselli, A. Brunelli, G. Pojana, A. Marcomini, G. Gallo and L. Canesi, Co-exposure to n-TiO2 and Cd2 + results in interactive effects on biomarker responses but not in increased toxicity in the marine bivalve M. galloprovincialis, Sci. Total Environ., 2014, 493, 355–364 CrossRef CAS PubMed.
- J. Farkas, S. Bergum, E. W. Nilsen, A. J. Olsen, I. Salaberria, T. M. Ciesielski, T. Bączek, L. Konieczna, W. Salvenmoser and B. M. Jenssen, The impact of TiO2 nanoparticles on uptake and toxicity of benzo(a)pyrene in the blue mussel (Mytilus edulis), Sci. Total Environ., 2015, 511, 469–476 CrossRef CAS PubMed.
- X. Fan, P. Wang, C. Wang, B. Hu and X. Wang, Lead accumulation (adsorption and absorption) by the freshwater bivalve Corbicula fluminea in sediments contaminated by TiO2 nanoparticles, Environ. Pollut., 2017, 231, 712–721 CrossRef CAS PubMed.
- X. Zhu, J. Zhou and Z. Cai, TiO2 Nanoparticles in the Marine Environment: Impact on the Toxicity of Tributyltin to Abalone (Haliotis diversicolor supertexta) Embryos, Environ. Sci. Technol., 2011, 45, 3753–3758 CrossRef CAS PubMed.
- X. Zhang, H. Sun, Z. Zhang, Q. Niu, Y. Chen and J. C. Crittenden, Enhanced bioaccumulation of cadmium in carp in the presence of titanium dioxide nanoparticles, Chemosphere, 2007, 67, 160–166 CrossRef CAS.
- H. E. Pace, N. J. Rogers, C. Jarolimek, V. A. Coleman, E. P. Gray, C. P. Higgins and J. F. Ranville, Single Particle Inductively Coupled Plasma-Mass Spectrometry: A Performance Evaluation and Method Comparison in the Determination of Nanoparticle Size, Environ. Sci. Technol., 2012, 46, 12272–12280 CrossRef CAS PubMed.
- F. Laborda, J. Jiménez-Lamana, E. Bolea and J. R. Castillo, Critical considerations for the determination of nanoparticle number concentrations, size and number size distributions by single particle ICP-MS, J. Anal. At. Spectrom., 2013, 28, 1220 RSC.
- K. Loeschner, J. Navratilova, C. Købler, K. Mølhave, S. Wagner, F. von der Kammer and E. H. Larsen, Detection and characterization of silver nanoparticles in chicken meat by asymmetric flow field flow fractionation with detection by conventional or single particle ICP-MS, Anal. Bioanal. Chem., 2013, 405, 8185–8195 CrossRef CAS PubMed.
- C. Ortmann and M. K. Grieshaber, Energy metabolism and valve closure behaviour in the Asian clam Corbicula fluminea, J. Exp. Biol., 2003, 206, 4167–4178 CrossRef CAS PubMed.
- E. Muth-Köhne, L. Sonnack, K. Schlich, F. Hischen, W. Baumgartner, K. Hund-Rinke, C. Schäfers and M. Fenske, The toxicity of silver nanoparticles to zebrafish embryos increases through sewage treatment processes, Ecotoxicology, 2013, 22, 1264–1277 CrossRef PubMed.
- S. Kühr, S. Schneider, B. Meisterjahn, K. Schlich, K. Hund-Rinke and C. Schlechtriem, Silver nanoparticles in sewage treatment plant effluents: chronic effects and accumulation of silver in the freshwater amphipod Hyalella azteca, Environ. Sci. Eur., 2018, 30, 7 CrossRef PubMed.
-
OECD, Test No. 211: Daphnia magna Reproduction Test, OECD, 2012.
- B. Schmidt, K. Loeschner, N. Hadrup, A. Mortensen, J. J. Sloth, C. Bender Koch and E. H. Larsen, Quantitative Characterization of Gold Nanoparticles by Field-Flow Fractionation Coupled Online with Light Scattering Detection and Inductively Coupled Plasma Mass Spectrometry, Anal. Chem., 2011, 83, 2461–2468 CrossRef CAS PubMed.
-
S. Sannac, S. Tadjiki and E. Moldenhauer, Single particle analysis using the Agilent 7700x ICP-MS, Agil. Technol.
- D. M. Mitrano, J. F. Ranville, A. Bednar, K. Kazor, A. S. Hering and C. P. Higgins, Tracking dissolution of silver nanoparticles at environmentally relevant concentrations in laboratory, natural, and processed waters using single particle ICP-MS (spICP-MS), Environ. Sci.: Nano, 2014, 1, 248–259 RSC.
- S. Đurović, B. Pavlić, S. Šorgić, S. Popov, S. Savić, M. Pertonijević, M. Radojković, A. Cvetanović and Z. Zeković, Chemical composition of stinging nettle leaves obtained by different analytical approaches, J. Funct. Foods, 2017, 32, 18–26 CrossRef.
- K. Vijayavel, S. Gopalakrishnan and M. P. Balasubramanian, Sublethal effect of silver and chromium in the green mussel Perna viridis with reference to alterations in oxygen uptake, filtration rate and membrane bound ATPase system as biomarkers, Chemosphere, 2007, 69, 979–986 CrossRef CAS.
- P. J. Wildridge, R. G. Werner, F. G. Doherty and E. F. Neuhauser, Acute Effects of Potassium on Filtration Rates of Adult Zebra Mussels, Dreissena polymorpha, J. Great Lakes Res., 1998, 24, 629–636 CrossRef CAS.
-
J. Rodgers, D. Cherry, R. Graney, K. Dickson and J. Cairns, in Aquatic Toxicology, ed. L. J. Weber, ASTM International, West Conshohocken, 1980, p. 266 Search PubMed.
- F. G. Doherty and D. S. Cherry, Tolerance of the Asiatic clam Corbicula spp. to lethal level of toxic stressors—A review, Environ. Pollut., 1988, 51, 269–313 CrossRef CAS PubMed.
- F. G. Doherty, D. S. Cherry and J. Cairns, Valve closure responses of the Asiatic clam Corbicula fluminea exposed to cadmium and zinc, Hydrobiologia, 1987, 153, 159–167 CrossRef CAS.
- E. Kádár, J. Salánki, R. Jugdaohsingh, J. J. Powell, C. R. McCrohan and K. N. White, Avoidance responses to aluminium in the freshwater bivalve Anodonta cygnea, Aquat. Toxicol., 2001, 55, 137–148 CrossRef.
- D. Tran, P. Ciret, G. Durrieu and J.-C. Massabuau, Estimation of potential and limits of bivalve closure response to detect contaminants: application to cadmium, Environ. Toxicol. Chem., 2003, 22, 914–920 CrossRef CAS PubMed.
- E. Fournier, D. Tran, F. Denison and J.-C. Massabuau, Valve closure response to uranium exposure for a freshwater bivalve (corbicula fluminea): quantification of the influence of pH, Environ. Toxicol. Chem., 2004, 23, 1108–1114 CrossRef CAS.
- C.-M. Liao, L.-J. Jou and B.-C. Chen, Risk-based approach to appraise valve closure in the clam Corbicula fluminea in response to waterborne metals, Environ. Pollut., 2005, 135, 41–52 CrossRef CAS PubMed.
- D. Tran, E. Fournier and G. Durrieu, Inorganic mercury detection by valve closure response in the freshwater clam corbicula fluminea: integration of time and water metal concentration changes, Environ. Toxicol. Chem., 2007, 26, 1545–1551 CrossRef CAS PubMed.
-
C. L. Klein, S. Comero, B. Stahlmecke, J. Romazanov, T. Kuhlbusch, E. Van Doren, P. J. De Temmerman, J. Mast, P. Wick and H. Krug, NM-Series of representative manufactured nanomaterials: NM-300 Silver characterisation, stability, homogeneity, EUR 24693 EN-2011.
- S. Lee, X. Bi, R. B. Reed, J. F. Ranville, P. Herckes and P. Westerhoff, Nanoparticle Size Detection Limits by Single Particle ICP-MS for 40 Elements, Environ. Sci. Technol., 2014, 48, 10291–10300 CrossRef CAS.
- M. D. Montaño, H. R. Badiei, S. Bazargan and J. F. Ranville, Improvements in the detection and characterization of engineered nanoparticles using spICP-MS with microsecond dwell times, Environ. Sci.: Nano, 2014, 1, 338–346 RSC.
- J. J. Doyle, J. E. Ward and R. Mason, An examination of the ingestion, bioaccumulation, and depuration of titanium dioxide nanoparticles by the blue mussel (Mytilus edulis) and the eastern oyster (Crassostrea virginica), Mar. Environ. Res., 2015, 110, 45–52 CrossRef CAS PubMed.
- C. A. García-Negrete, J. Blasco, M. Volland, T. C. Rojas, M. Hampel, A. Lapresta-Fernández, M. C. Jiménez de Haro, M. Soto and A. Fernández, Behaviour of Au-citrate nanoparticles in seawater and accumulation in bivalves at environmentally relevant concentrations, Environ. Pollut., 2013, 174, 134–141 CrossRef PubMed.
-
R. F. McMahon, Ecology of an invasion pest bivalve, Corbicula, The mollusca, 1983, vol. 6, pp. 505–561 Search PubMed.
-
ASTM International, Standard Guide for Conducting Bioconcentration Tests with Fishes and Saltwater Bivalve Mollusks (E1022 - 94), West Conshohocken, PA, United States, 2013 Search PubMed.
-
US-EPA, Ecological Effects Test Guidelines OPPTS 850.1710 Oyster BCF, 1996.
- L. Su, Y. Xue, L. Li, D. Yang, P. Kolandhasamy, D. Li and H. Shi, Microplastics in Taihu Lake, China, Environ. Pollut., 2016, 216, 711–719 CrossRef CAS PubMed.
- P. Oliveira, L. G. A. Barboza, V. Branco, N. Figueiredo, C. Carvalho and L. Guilhermino, Effects of microplastics and mercury in the freshwater bivalve Corbicula fluminea (Müller, 1774): Filtration rate, biochemical biomarkers and mercury bioconcentration, Ecotoxicol. Environ. Saf., 2018, 164, 155–163 CrossRef CAS PubMed.
- L. Guilhermino, L. R. Vieira, D. Ribeiro, A. S. Tavares, V. Cardoso, A. Alves and J. M. Almeida, Uptake and effects of the antimicrobial florfenicol, microplastics and their mixtures on freshwater exotic invasive bivalve Corbicula fluminea, Sci. Total Environ., 2018, 622–623, 1131–1142 CrossRef CAS PubMed.
Footnote |
† Electronic supplementary information (ESI) available. See DOI: 10.1039/c9en01112a |
|
This journal is © The Royal Society of Chemistry 2020 |
Click here to see how this site uses Cookies. View our privacy policy here.