Smectite clay pillared with copper complexed polyhedral oligosilsesquioxane for adsorption of chloridazon and its metabolites†
Received
28th August 2019
, Accepted 21st November 2019
First published on 21st November 2019
Abstract
Chloridazon has been a widely used herbicide during the past decades, especially in sugar-beet cultivation. UV-induced degradation of chloridazon leads to the formation of desphenyl counterparts, i.e. desphenyl-chloridazon and methyl-desphenyl-chloridazon. Even if accumulation of these residues in natural waters is far from alarming, a low-cost effective and environmentally friendly adsorbent, capable of binding chloridazon and its degradation products is desirable to reduce their concentration in water even further below legal limits. Here we show that pillared smectite clay, prepared by cation exchange of sodium with copper complexed, cage-shaped polyhedral oligomeric silsesquioxane (Cu2+@POSS) could be a promising candidate for this purpose. X-ray diffraction and high resolution transmission electron microscopy evidenced a homogeneous layered structure where the interlayer spacing is enlarged by 7.1 ± 0.2 Å (the diameter of Cu2+@POSS) with respect to the pristine clay. Exposure of this pillared smectite clay to chloridazon and its metabolites in water showed that Cu2+@POSS intercalation significantly improved its adsorption capacity. In addition, after several thermal regeneration cycles, Cu2+@POSS_SWy-2 still exhibited excellent adsorption properties. These findings demonstrate that smectite clay pillared with copper complexed polyhedral oligosilsesquioxane is a promising environmentally friendly and relatively low cost material for herbicide waste remediation.
Environmental significance
Chloridazon, a herbicide largely employed in European agriculture, and its metabolites have accumulated in the environment to a level where their elimination from drinking water is recommended to safeguard human health but a recyclable and relatively inexpensive remediation technology for such herbicides has not been developed yet. This work proposes smectite clay pillared with copper-complexed polyhedral oligosilsesquioxane as an adsorbent, and demonstrates the efficacy of this material in extracting chloridazon and its metabolites from water. The design of this adsorbent can be adapted to other contaminants by choosing appropriate ligands for the polyhedral oligosilsesquioxane and metal ions for the complexation as to optimize the pore size and affinity for trapping the chosen molecule.
|
Introduction
Addressing the lack of adequate and safe water is among the most important challenges in the last few decades.1 Groundwater, an important source of drinking water in most countries, has been threatened by herbicide/pesticide contamination due to runoff from the soil surface and plants to rivers and lakes.2 Studies on groundwater contamination have reported increasing amounts of herbicides/pesticides and their metabolites.3,4 Chloridazon, a selective systemic herbicide used for the control of annual broadleaf weeds, has been widely employed especially in sugar-beet cultivation since it was considered to be a relatively non-harmful herbicide.5 Its degradation leads to the formation of desphenyl-chloridazon and methyl-desphenyl-chloridazon;6 the former, produced by aerobic degradation in soil, is predominant. According to European regulations, the maximum residue limit for herbicides is 0.1 μg L−1 for single compounds and 0.5 μg L−1 for the sum of all herbicides.7 As reported by Schuhmann et al.,8 in some parts of Europe, chloridazon was detected at concentrations up to 3.5 μg L−1, whereas the metabolites desphenyl-chloridazon and methyl-desphenyl-chloridazon were present in concentrations of 24.0 μg L−1 and 6.1 μg L−1, respectively.
In order to limit exposure to chloridazon and its metabolites, their elimination from natural water by the use of an innovative adsorbent with high selectivity and sorption capacity, but available at a relatively low cost, would be an important step forward.9 Since the beginning of civilization, natural clay minerals have been employed by mankind and because of their low cost, abundance in most continents, high sorption properties and potential for ion exchange, clay materials are also a good option for the present purpose.10,11 Due to their layered structure, clay materials can host adsorbates and counter ions in the space between the platelets. They exhibit a strong affinity for both cationic and anionic moieties, whereas their sorption capacity for basic molecules is much higher than that for acidic ones because of the negative charge inherent in the clay structure.12 However, not solely the chemical affinity of the interlayer space of clay can limit the adsorption capacity for chloridazon, but also the size of the interlayer space plays an important role and it is therefore crucial to modify the structure of the clay in order to improve its adsorption capacity.
Pillaring is a well-known technique for engineering the interlayer space in clay materials by increasing the specific surface area and providing accessible, active sites for adsorption.13,14 Molecules,15 ions,16 or complexes17 can be used as pillaring agents. Among them, organic–inorganic cage-like polyhedral oligomeric silsesquioxanes (POSS) are particularly flexible18,19 because different organic ligands can tune the functionalization to achieve desired properties. Moreover, the chemical affinity of the nanopores created by pillaring with POSS can be tailored through complexation of metal ions.20,21
In this work, we report the intercalation of Cu2+-complexed POSS into smectite clay towards the synthesis of a novel type of pillared structure suitable for environmental remediation. Copper was chosen as a complex metal because of its affinity for nitrogen-containing groups which shows potential for enhancing the adsorption capacity for chloridazon and its metabolites. POSS was selected because it presents three important advantages: i) the eight nitrogen containing organic ligands (–NH2 and –NH) on each POSS allow complexation of 4 Cu ions; ii) POSS′ cubic shape separates the complexed copper ions from each other and avoids aggregation during the synthesis process, which would reduce the activity of copper ions, and iii) provides the appropriate interlayer space between the smectite layers and the right porosity for chloridazon to interact.
Characterization of the nanocomposite included X-ray diffraction (XRD), high-resolution transmission electron microscopy (HRTEM), scanning electron microscopy (SEM), Fourier transform infrared spectroscopy (FTIR), nitrogen adsorption–desorption measurements, thermogravimetric and differential thermal analysis (TGA/DTA) and X-ray photoelectron spectroscopy (XPS). The pillared clay exhibited a high specific surface area; compared to pristine smectite clay, it showed significant enhancement of adsorption capacity for chloridazon and its metabolites.
Experimental
Materials
Wyoming montmorillonite (SWy-2, (Na, Ca)0.3(Al, Mg)2Si4O10(OH)2·n(H2O)), with a cation exchange capacity (CEC) of 78 meq/100 g and flake size of ∼200 μm, was obtained from the Source Clay Minerals Repository, Columbia. 3-(2-Aminoethylamino)-propyltrimethoxysilane (EDAPTMOS) was acquired from Fluka Chemicals, chloridazon (C10H8ClN3O, AR) from Sigma-Aldrich, desphenyl-chloridazon (C4H4ClN3O, AR) and methyl-desphenyl-chloridazon (C5H6ClN3O, AR) from Enamine Ltd. All the chemicals were used as received.
Synthesis of the Cu2+@POSS_SWy-2 nanocomposite
Milli-Q water (resistivity 18 MΩ cm, 25 °C) was used in the synthesis. SWy-2 was purified with the standard method in clay science, as described in our previous paper.22 Sodium exchanged montmorillonite was prepared by immersing the pristine clay in 1 N sodium chloride solution, washing it with distilled deionized water and transferring it to dialysis tubes in order to obtain chloride-free clay.22 The synthesis of cage-shaped POSS was based on a hydrolytic polycondensation reaction; EDAPTMOS was diluted in ethanol/water (v/v = 14/1) to give a 0.45 M POSS solution, which was shaken for a few minutes before bottling for further use.23,24 20 mL of 0.15 M CuCl2 aqueous solution (3 mmol) was complexed with 14 mL POSS solution (6 mmol) through sonication and stirred for 30 min. The complexation of the copper cations with the amino and imino groups on the R branches of POSS was evident from the colour change of the complex solution, which turned from light blue to dark blue. The Cu2+@POSSS_SWy-2 nanocomposite was prepared by cation exchange of sodium with copper-complexed POSS (Cu2+@POSS) as indicated in Scheme 1. A suspension of 0.5 wt% SWy-2 in water was mixed with Cu2+@POSS in a weight ratio [Cu2+@POSS]/[SWy-2] = 3, which ensures that there are sufficient siloxane complexes to exchange for the sodium ions in the clay interlayer space. After stirring for 24 h, the Cu2+@POSS_SWy-2 nanocomposite was separated by centrifugation at 5000 rpm and washed three times with ethanol/water (v/v = 1
:
1), before being spread on glass plates for air drying.
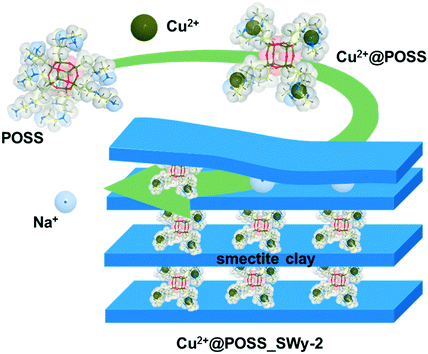 |
| Scheme 1 Schematic representation of the complexation of POSS with Cu2+, and of the intercalation of the POSS complex into the inter-lamellar space of the smectite clay. | |
In order to study the role of Cu2+, the POSS-pillared clay without the complex metal was also prepared by the same synthetic route; this nanocomposite will be identified as POSS_SWy-2 in the following.
Characterization of the pillared nanocomposite Cu2+@POSS_SWy-2
FTIR spectra were recorded on a SHIMADZU 8400 infrared spectrometer in the range of 4000–500 cm−1; each spectrum was the average of 200 scans, collected with a resolution of 2 cm−1. The samples were KBr pellets that contained 2 wt% Cu2+@POSS_SWy-2. XRD spectra were acquired using a D8 Advance Bruker diffractometer with Cu Kα radiation (λ = 1.5418 Å) employing a 0.25° divergent slit and a 0.125° anti-scattering slit; the patterns were recorded in the 2θ range from 2° to 80°, in steps of 0.02° and a counting time of 2 s per step. The nitrogen adsorption–desorption isotherms were measured at −196 °C on a Micromeritics ASAP 2420 V2.05 (V2.05J) porosimeter. Prior to analysis, all three samples were degassed overnight at 120 °C under vacuum. The specific surface area was evaluated with the Brunauer–Emmett–Teller (BET) model by fitting the N2 adsorption isotherms, the pore volume was determined at P/P0 = 0.995 and the non-local density functional theory (NLDFT) method25 was applied to the N2 adsorption data to obtain the pore size distribution. TGA/DTA was performed using a Perkin Elmer Pyris Diamond TG/DTA. Samples of approximately 5 mg were heated in N2 from 25 °C to 700 °C, at a rate of 5 °C min−1. TEM images were obtained by using an FEI Tecnai T20, operating at 200 keV. Images were recorded under low-dose conditions with a slow-scan CCD camera. SEM images were recorded with an FEI-Philips FEG-XL30s microscope, and energy dispersive X-ray spectroscopy (EDS) was also performed with the help of an EDAX Octane silicon drift detector with an accelerating voltage of 20 kV. XPS was performed with an SSX-100 (Surface Science Instruments) spectrometer equipped with a monochromatic AlKα X-ray source (hν = 1486.6 eV). The measurement chamber pressure was maintained at 1 × 10−9 mbar during data acquisition; the photoelectron take-off angle was 37° with respect to the surface normal. The samples were dispersed in ethanol/water (v/v = 30/70) by sonication and stirring for 30 min, and a small drop of the suspension was left to dry in air on a homemade26 150 nm thick gold film supported on mica. Spectral analysis included Shirley background subtraction and fitting with peak profiles taken as a convolution of Gaussian and Lorentzian functions, with the help of the least squares curve-fitting program WinSpec (LISE, University of Namur, Belgium). Binding energies (BEs) were referenced to the Si2p peak27 at 103.3 eV and are accurate to ±0.1 eV when deduced from the fitting procedure. All measurements were carried out on freshly prepared samples; three different spots were measured on each sample to check for homogeneity.
Batch adsorption experiments
10 mg of Cu2+@POSS_SWy-2, POSS_SWy-2 or SWy-2 was swelled in 10 mL ethanol/water (30/70, v/v) for 24 h under stirring and sonication in a glass vial. Stock solutions of 50 ppm chloridazon, desphenyl-chloridazon and methyl-desphenyl-chloridazon were prepared in ethanol/water (30/70, v/v). Batch adsorption experiments were conducted at ambient temperature. Chloridazon, desphenyl-chloridazon or methyl-desphenyl-chloridazon was added, at concentrations adjusted from 5 ppm to 25 ppm, to 10 mg of swelled suspensions of Cu2+@POSS_SWy-2, POSS_SWy-2 or SWy-2; the suspensions were stirred for 24 h. After the adsorption equilibrium was reached, the suspensions were centrifuged and filtered with 0.45 μm nylon filters. The concentrations of the herbicide and its metabolites were measured with high performance liquid chromatography (Prominence HPLC, Shimadzu) using a diode array detector and data station and analysed at 283 nm, the wavelength of maximum absorption of these compounds. All of the experimental data reported were the average of triplicate determinations. The adsorption capacities of the organic solute by Cu2+@POSS_SWy-2, POSS_SWy-2 and SWy-2 were calculated with the equation: | Qe = [(C0 − Ce)/m] × V | (1) |
where C0 and Ce are the initial and the adsorption equilibrium concentrations, respectively; m is the mass of the adsorbent and V represents the volume of the suspension.
The adsorption isotherms were analysed with the Freundlich isotherm model, commonly used for aqueous phase adsorption:
| 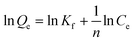 | (2) |
where
Kf represents the Freundlich constant,
n is the Freundlich linearity index and
Ce represents the adsorption equilibrium concentration.
Thermal regeneration experiments
250 mg of Cu2+@POSS_SWy-2 was added to 250 mL ethanol/water (30/70, v/v) in a glass vial and stirred for 24 h. Stock solutions of 50 ppm chloridazon were prepared in ethanol/water (30/70, v/v). 250 mL of the stock solution of chloridazon was added to the Cu2+@POSS_SWy-2 suspension and the mixture was stirred for 24 h. The adsorbent was separated by centrifugation and dried in an oven (Thermol Scientific Heraeus) overnight at 40 °C, followed by heating in a different oven (Nabertherm box furnace) at 290 °C for 4 h. After that, the cooled sample was carefully weighed and used for the second cycle. Adsorption experiments were carried out with an adsorbent concentration of 0.5 mg mL−1 and a chloridazon concentration of 25 mg L−1; four cycles were performed.
Results and discussion
Material characterization
The samples were analysed by FTIR to confirm the successful incorporation of the POSS derivative in the layered structure. The spectra of pristine SWy-2, POSS_SWy-2 and Cu2+@ POSS_SWy-2 are shown in Fig. 1(a). One recognizes the characteristic peaks of the smectite clay at 1060 cm−1 (Si–O stretching), 695 cm−1 and 642 cm−1 (Si–O–Si bending vibrations).28 In addition, in the spectrum of pristine SWy-2, the –OH stretching vibration around 3630 cm−1 is ascribed to the presence of –OH on the aluminosilicate skeleton, while the stretching modes at 3400–3600 cm−1 and the bending vibration at 1645 cm−1 can be attributed to physisorbed water. A confirmation of the presence of POSS in the hybrid material comes from the bands centred at 2863 cm−1 and 2921 cm−1, which correspond to C–H stretching vibrations, as well as from the peak at 1520 cm−1, due to the N–H stretching mode.29
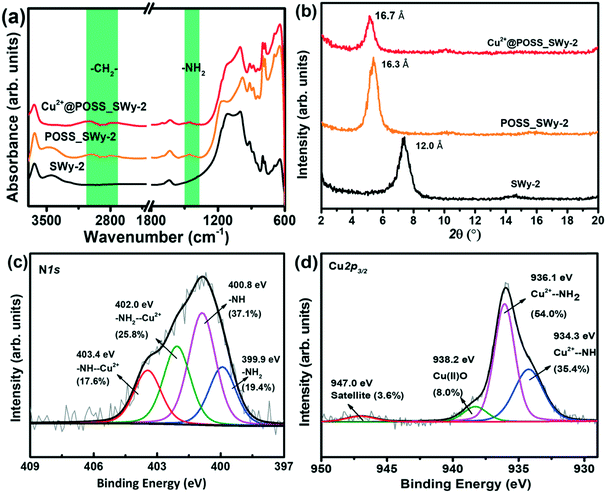 |
| Fig. 1 (a) FTIR spectra and (b) XRD spectra of SWy-2, POSS_SWy-2 and Cu2+@POSS_SWy-2; X-ray photoelectron spectra of Cu2+@POSS_SWy-2 (c) N1s and (d) Cu2p3/2 core level regions. | |
The successful intercalation of POSS derivatives in SWy-2 can be proved by XRD, which allows estimation of the interlayer distance between the clay platelets. The XRD spectra of SWy-2, POSS_SWy-2 and Cu2+@POSS_SWy-2 are shown in Fig. 1(b). By applying the Bragg equation, one can derive the basal d001-spacing, which in the pristine smectite clay amounts to 12.0 ± 0.3 Å, but becomes 16.3 ± 0.3 Å after intercalation with POSS. The latter basal plane spacing corresponds to an interlayer separation Δ = (16.3 ± 0.3) Å − 9.6 Å = 6.7 ± 0.3 Å, where 9.6 Å represents the thickness of a single clay layer.30 This value is in accordance with the size of POSS.30 For Cu2+@POSS_SWy-2, the basal plane spacing is even larger, namely 16.7 ± 0.4 Å, and the corresponding interlayer separation of (16.7 ± 0.2) Å − 9.6 Å = 7.1 ± 0.2 Å points to successful Cu2+ complexation with POSS and intercalation of Cu2+@POSS between the smectite clay layers.31
To verify the presence and integrity of Cu2+@POSS within the layered nanostructure, as well as to analyse the chemical environment of Cu2+ and POSS, we employed XPS. The overview spectrum attests to the presence of all the expected elements (see ESI,† Fig. S1). The XPS spectrum of the N1s core level region of Cu2+@POSS_SWy-2, shown in Fig. 1(c), shows four contributions in the fit: the spectral signatures of –NH2 and –NH bonds of the POSS are centred at BEs of 399.9 eV and 400.8 eV, respectively, and make up 19.4 ± 4% and 37.1 ± 3% of the total N1s intensity. The contributions at BEs of 403.4 eV and 402.0 eV are respectively due to NH–Cu2+ and NH2–Cu2+ and represent 17.6 ± 4% and 25.8 ± 3% of the total N1s intensity. Hence the spectral intensities of the total amounts of –NH2 (–NH2 and –NH2–Cu2+) and –NH– (–NH– and –NH–Cu2+) are almost equal (45.2 ± 2% and 54.7 ± 2%, respectively), which dovetails with the ratio of the two kinds of amino groups in EDAPTMOS. The fact that the spectral intensity of –NH2–Cu2+ is higher than that of –NH–Cu2+ implies that –NH2 exhibits a stronger affinity for Cu2+.
The XPS spectrum of the Cu2p3/2 core level region of Cu2+@POSS_SWy-2 is shown in Fig. 1(d), where the contributions at BEs of 934.3 eV, 936.1 eV and 938.2 eV correspond respectively to the electron-rich NH group Cu2+–NH, the relatively electron-poor amino Cu2+–NH2 and Cu(II)O; the small peak at 947.0 eV is the shake-up satellite.32,33 The ratio between Cu2+–NH and Cu2+–NH2 in the Cu2p3/2 spectrum agrees with the N1s spectrum. Taken together, the XPS spectra confirm that Cu2+@POSS was successfully intercalated into SWy-2.
Undeniable proof for the successful intercalation of Cu2+@POSS into the interlayer space of SWy-2 is provided by the high-resolution transmission electron microscopy (HRTEM) images shown in Fig. 2, where the layered structure can be clearly observed in panel (a). The interlayer spacing can be retrieved by performing a fast Fourier transform (FFT) of the image, where the diffraction corresponding to the (001) planes of the SWy-2 structure is observed as shown in Fig. 2(b). Performing an inverse FFT on the marked area, the basal plane spacing d002 is measured to be ∼1.79 nm in agreement with the XRD result discussed above.
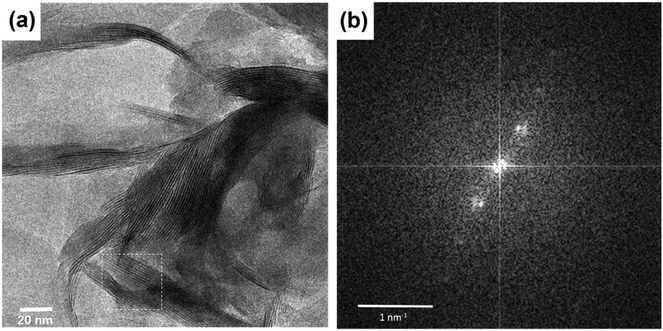 |
| Fig. 2 (a) TEM image of Cu2+@POSS_SWy-2. (b) Fast Fourier transform (FFT) of the square marked in white. An interlayer distance of 17.9 Å is deduced from this graph. | |
In order to gain further insight into the morphology of the composite material, SEM was performed. A well-defined porous structure, which resembles the crumpled sheet, can be observed on the image in Fig. 3(a). In an enlarged view of the same spot, presented in Fig. 3(b), the exfoliated layers (white arrows) and the enlarged interlayer space (yellow arrows) of the smectite clay can be clearly seen.
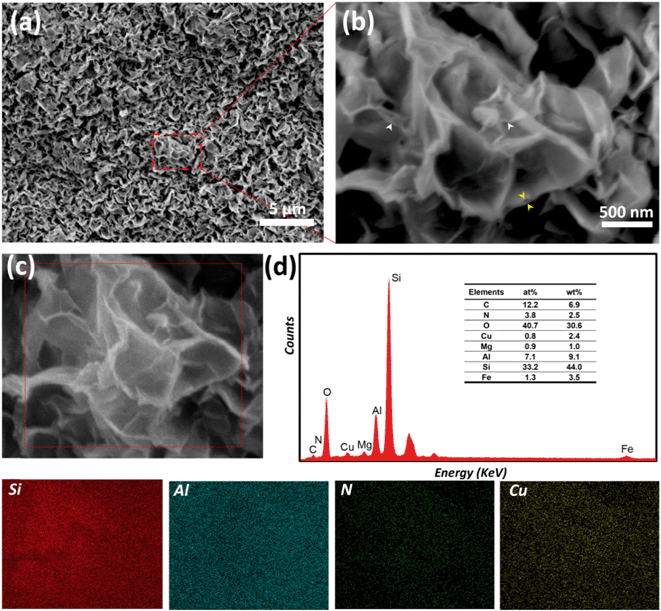 |
| Fig. 3 (a) and (b) SEM images of Cu2+@POSS_SWy-2. (c) SEM image with the area selected for energy dispersive X-ray spectroscopy (EDS) spectra and EDS elemental mapping measurement marked in red. (d) SEM-EDS spectra of Cu2+@POSS_SWy-2. Inset: The elemental composition results. The bottom panels show the EDS elemental mapping results for Si, Al, N and Cu in Cu2+@POSS_SWy-2 based on the red square area in (c). | |
Furthermore, EDS elemental mapping was performed to verify whether the pillaring structures were uniformly distributed and to determine the relative ratio of Cu2+ in Cu2+@POSS_SWy-2. As shown in Fig. 3, we collected the EDS spectra in the area marked in dark red in the SEM image (Fig. 3(c)) and found identical spectra, of which one is shown in Fig. 3(d). We then imaged the distribution of Si, Al, N and Cu in the area enclosed in the red square area (Fig. 3(c)) and found a homogeneous distribution for all four elements, which indicates that the insertion of Cu2+ into the smectite clay is uniform throughout the sample. In addition, Al and Si are the main components of the smectite layers, which are composed of an octahedral alumina layer and sandwiched between two tetrahedral silica layers. From the elemental composition deduced from the EDS spectra, we can infer that the amount of Cu2+ in the nanocomposite is approximately 2.4 wt%.
To estimate the amount of POSS and Cu2+@POSS introduced into SWy-2, TGA-DTA was performed on POSS_SWy-2 and Cu2+@POSS_SWy-2, as well as on the pristine clay; the results are shown in Fig. 4(a–c). For the pristine smectite clay, the broad peak trace observed at 667 °C on the relevant DTA marks the dihydroxylation of structural –OH groups in SWy-2, which make up 3.0% of the total mass.34 For POSS_SWy-2 and Cu2+@POSS_SWy-2, the region from 200 °C to 500 °C refers to the decomposition of POSS: between 200 °C and 300 °C, amino groups detach from POSS,35 followed by the combustion of the alkane on the R group of POSS between 300 °C and 500 °C.21 The Si–O bond decomposition of the POSS-cage and the dehydroxylation of the structural –OH group in SWy-2 occur between 500 °C and 700 °C.36 From these data, we can estimate that for POSS_SWy-2, the amount of POSS intercalated into SWy-2 equals 2.0 wt% of the total mass, while for Cu2+@POSS_SWy-2, it reaches 8.0 wt% of the total mass. In other words, Cu2+ favours the insertion of POSS between SWy-2 layers. If we calculate the amount of nitrogen based on the POSS structure, we find that nitrogen should make up about 2.5 wt% of the total weight, in good agreement with the EDS result (2.6%).
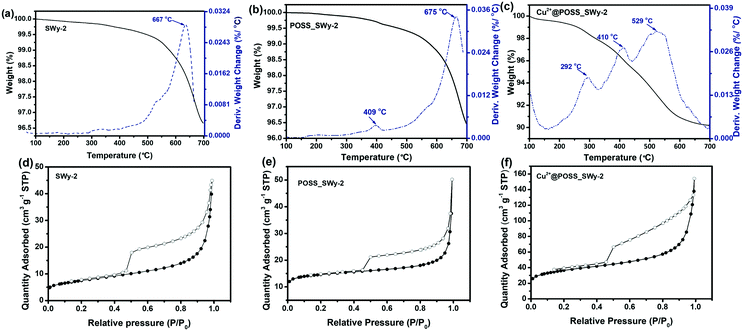 |
| Fig. 4 Thermogravimetric analysis (TGA) and differential thermal analysis (DTA) data for (a) SWy-2, (b) POSS_SWy-2 and (c) Cu2+@POSS_SWy-2. N2 adsorption–desorption isotherms of SWy-2 (d), POSS_SWy-2 (e) and Cu2+@POSS_SWy-2 (f). | |
Nitrogen adsorption–desorption measurements were performed on SWy-2, POSS_SWy-2 and Cu2+@POSS_SWy-2 to monitor the increase of surface area with intercalation and to retrieve information on the pore structure; the isotherms are presented in Fig. 4(d–f). As for the pristine smectite, a sudden release of N2 at P/P0 ≈ 0.5 gives rise to a type H3 hysteresis loop, commonly ascribed to slit-shaped pores in layered materials, and the adsorption–desorption isotherm corresponds to a type II isotherm, attributed to the general microporous structure.37 After POSS and Cu2+@POSS intercalation, the hysteresis loop widens, implying an increase in pore volume from 0.093 cm3 g−1 in the pristine material to 0.230 cm3 g−1 in Cu2+@POSS_SWy-2 (Table 1).38 The specific surface area (SSA) and the pore volume calculated from the BET data are reported in Table 1. The SSA for SWy-2 was found to amount to 26 m2 g−1, but increased to 127 m2 g−1 after intercalation with Cu2+@POSS. This indicates that N2 can only be adsorbed on the external surface and the interlayer space remains inaccessible in the pristine material, while the opening of the interlayer space in the nanocomposite allows for N2 penetration. However, compared to POSS_SWy-2, a significant increase of SSA can be observed for Cu2+@POSS_SWy-2; this indicates that the Cu2+ enhanced POSS intercalation leads to the formation of more mesoporous structures, which can result in a dramatically increased pore surface area.39
Table 1 Specific surface area, total pore volume and micropore volume of SWy-2, POSS_SWy-2 and Cu2+@POSS_SWy-2
Samples |
Specific surface area (SBET) [m2 g−1] |
Total pore volume [cm3 g−1] |
Micropore volume [cm3 g−1] |
SWy-2 |
26 |
0.093 |
0.004 |
POSS_SWy-2 |
54 |
0.143 |
0.026 |
Cu2+@POSS_SWy-2 |
127 |
0.230 |
0.045 |
To gain better insight into the structural characteristics of our materials, we determined the pore size distribution (PSD). For this, the NLDFT method was employed since both micropores and mesopores are present in the structure of pillared smectite clay materials.40 The PSD results obtained from the NLDFT model are shown in Fig. S2(a),† where one notes that the relative amount of micropores increased with the intercalation of POSS and Cu2+@POSS. The intercalation of POSS in SWy-2 has a limited effect on the internal and external pore distributions.41 However, when Cu2+@POSS was used as a pillaring agent, the microporosity as well as mesoporosity increased significantly (Fig. S2(b)†). In the micropore range, the peaks in the pore diameter centred at 0.5 nm and 1.4 nm for Cu2+@POSS_SWy-2 differ considerably from the broad peak at 0.7 nm observed when pillaring with POSS.41 The complexation of Cu2+ with the nitrogen-containing ligands results in an increased rigidity of the POSS molecule, which might result in micropores,42 whose size is centred at 0.5 nm. Since Cu2+@POSS is positively charged, the narrowly distributed pore sizes around 1.4 nm might be the result of electrostatic repulsion.13 Such electrostatic repulsion could also be responsible for the larger amount of randomly distributed mesopores, giving rise to the hierarchical micro-/mesoporous structure seen in the TEM image, which results in the increase in the total pore volume reported in Table 1.
Adsorption isotherms and adsorption capacity for chloridazon and its metabolites
The adsorption isotherms of chloridazon on SWy-2, POSS_SWy-2 and Cu2+@POSS_SWy-2 are shown in Fig. 5(a). In the Giles classification, these isotherms can be classified as L-type, which indicates that pristine and intercalated smectite clay materials have a moderate affinity for chloridazon and there is no strong competition from the solvent.43 However, the slope of the initial part of the adsorption isotherms on Cu2+@POSS_SWy-2 is much higher than on SWy-2, which illustrates that Cu2+@POSS_SWy-2 and POSS_SWy-2 have a higher affinity for chloridazon than SWy-2. For an initial concentration of 25 mg L−1, the amount of chloridazon adsorbed on Cu2+@POSS_SWy-2 (Qe) is nearly 3 times that on SWy-2 and 1.5 times that on POSS_SWy-2. To determine the adsorption capacity of the nanocomposites for chloridazon, the adsorption data were fitted with the Freundlich model and the result is shown in Fig. 5(b). The fit and the statistical analysis were performed with Origin software and the estimated parameters are given in Table 2. Kf is an empirical constant related to the adsorption capacity (mg(1−n) kg−1 Ln), n is the Freundlich exponent or a site energy heterogeneity factor, often used as a measure of isotherm nonlinearity,44 and the value of the correlation coefficient R2 relates to the quality of the isotherm fit: the closer the value to 1, the better the fit. As can be seen from Table 2, R2 values amounted to 0.992, 0.998 and 0.996 for SWy-2, POSS_SWy-2 and Cu2+@POSS_SWy-2, confirming a good fit in all cases. The empirical constant Kf increased 8 times for the adsorption of chloridazon on Cu2+@POSS_SWy-2 compared to that for adsorption on the pristine clay. The higher adsorption capacity of Cu2+@POSS_SWy-2 can be ascribed to two reasons: on the one hand, the significantly higher specific surface area of Cu2+@POSS_SWy-2 provides more physisorption sites for chloridazon. On the other hand, the presence of Cu2+, which has a strong affinity for Cl- and N-containing organic molecules, enhances the chemisorption of chloridazon.
 |
| Fig. 5 Adsorption isotherms of (a) chloridazon (inset: structural model of chloridazon; grey = carbon, blue = nitrogen, red = oxygen and green = chlorine), (c) desphenyl-chloridazon (inset: structural model of desphenyl-chloridazon, colour codes same as those in (a)), and (e) methyl-desphenyl-chloridazon (inset: structural model of methyl-desphenyl-chloridazon, colour codes of the balls same as those in (a)). Freundlich model fitting for the adsorption of (b) chloridazon on Cu2+@POSS_SWy-2, POSS_SWy-2 and SWy-2, and Freundlich model fitting for the adsorption of (d) desphenyl-chloridazon and (f) methyl-desphenyl-chloridazon on Cu2+@POSS_SWy-2 and SWy-2. X-ray photoelectron spectra of chloridazon + Cu2+@POSS_SWy-2: (g) Cu2p3/2 and (h) Cl2p core level regions. | |
Table 2 Parameters of Freundlich isotherm model Qe = Kf × Ce1/n for the adsorption of chloridazon, desphenyl-chloridazon and methyl-desphenyl-chloridazon on SWy-2, POSS_SWy-2 and Cu2+@POSS_SWy-2
Samples |
K
f
|
n
|
R
2
|
Chloridazon |
SWy-2 |
58.22 |
0.900 |
0.992 |
POSS_SWy-2 |
137.01 |
1.008 |
0.998 |
Cu2+@POSS_SWy-2 |
471.07 |
1.305 |
0.996 |
Desphenyl-chloridazon |
SWy-2 |
17.15 |
0.892 |
0.986 |
Cu2+@POSS_SWy-2 |
81.29 |
1.262 |
0.984 |
Methyl-desphenyl-chloridazon |
SWy-2 |
10.55 |
0.861 |
0.991 |
Cu2+@POSS_SWy-2 |
25.68 |
0.934 |
0.984 |
In order to check the adsorption mechanism of chloridazon on Cu2+@POSS_SWy-2, XPS was performed. As shown in Fig. 5(g), the Cu2p3/2 core-level region requires 5 components in the fit. The three components also present in the starting material, i.e. the first one due to Cu2+–NH at a BE of 934.2 eV, the second one stemming from Cu2+–NH2 at 936.0 eV and the third originating from Cu(II)O at 938.3 eV,33,45 contribute here differently to the Cu2p3/2 spectral intensity. The increased relative intensity of the Cu2+–NH2 component (now 61.0%) and the decreased relative intensity of the Cu2+–NH one (now 4.4%) confirm that the –NH2 group of chloridazon is very active towards Cu2+ and replaces the weakly bonded –NH group. Additionally, the two new components at BEs of 936.9 eV and 940.3 eV can be ascribed to the adsorption of Cl to Cu2+ and Cu(II)O, respectively. In fact, since the –Cl group of chloridazon is more electronegative than –NH2, Cu2+ to which it is attached becomes more electron-poor, and hence appears at a higher BE.45 Likewise, –Cl attached to Cu(II)O further decreases the electron density around the Cu2+, resulting in an additional component at a higher binding energy.33 Correspondingly, the Cl2p core-level region (Fig. 5(h)) can be fitted with three components at BEs of 198.1 eV, 199.4 eV and 200.8 eV and originating respectively from Cl–Cu(II)O,46 Cl–Cu2+,45 and Cl-cyclic of chloridazon.47 The N1s core level region, reported in the ESI,† agrees with this interpretation.
The uptake of desphenyl-chloridazon on Cu2+@POSS_SWy-2 was also found to be significantly higher than that on SWy-2, as seen from the adsorption isotherms shown in Fig. 5(c). At an initial concentration of 25 mg L−1, the Qe for desphenyl-chloridazon adsorbed on Cu2+@POSS_SWy-2 is nearly 2 times that on SWy-2. The Freundlich model fitting results are shown in Fig. 5(d) and the data are listed in Table 2. Similar to chloridazon, also for desphenyl-chloridazon, the empirical constant Kf significantly increased for adsorption on Cu2+@POSS_SWy-2 as compared to pristine SWy-2, and the Freundlich exponent n changed from 0.892 to 1.262, testifying to an improved adsorption capacity.
For the uptake of the other metabolite, methyl-desphenyl-chloridazon, the isotherms are shown in Fig. 5(e) and their fit with the Freundlich model is shown in Fig. 5(f). Since methyl-desphenyl-chloridazon and desphenyl-chloridazon have a similar chemical structure, their adsorption behaviour is analogous. At an initial concentration of 25 mg L−1, the Qe for methyl-desphenyl-chloridazon on Cu2+@POSS_SWy-2 was determined to amount to 891 mg kg−1, which is twice that on SWy-2. The observed difference between the uptake of chloridazon, desphenyl-chloridazon and methyl-desphenyl-chloridazon is attributed to the different substituent group at the imino position. The significantly higher uptake capacity for chloridazon than those for its two metabolites is presumably related to the phenyl group. On the one hand, the higher adsorption capacity can be ascribed to the hydrophobic effects because the siloxanes of the basal planes are considered to be hydrophobic, and therefore have a higher affinity for organic molecules containing aromatic rings.48 This type of bonding is not present for desphenyl-chloridazon and methyl-desphenyl-chloridazon, where the phenyl group is replaced by hydrogen or by a methyl group. On the other hand, the phenyl group is electron richer than –CH3 or –H, which means it can donate more electronic charges to –NH2 and –Cl to make them more electronegative than in desphenyl-chloridazon and methyl-desphenyl-chloridazon. This results in Cu2+ having a relatively higher affinity for chloridazon.
Thermal regeneration analysis
An important issue for assessing the potential of Cu2+@POSS_SWy-2 as a chloridazon adsorbent is to test its reusability; hence we regenerated the material thermally and followed the sorption behaviour over four adsorption/regeneration cycles. The TGA/DTA data for chloridazon, presented in Fig. 6(a), show that decomposition occurs around 290 °C. However, as discussed above (Fig. 6(b)), below 300 °C, the amino groups detach from POSS, while Cu2+ remains in the interlayer space of SWy-2. This means that after decomposition of the adsorbed chloridazon at 290 °C, the structure of Cu2+@POSS_SWy-2 is slightly altered, but the pillaring Si–O cubes still remain intact. We therefore checked if the material still adsorbs chloridazon after this regeneration treatment. The adsorption capacity of Cu2+@POSS_SWy-2 for chloridazon as a function of number of regeneration cycles is plotted in Fig. 6(c). While the pristine Cu2+@POSS_SWy-2 adsorbs 4920 ± 36 mg kg−1, after four thermal regeneration cycles, the adsorption capacity for chloridazon was 3260 ± 61 mg kg−1, i.e. reduced by 32.3%. To check whether this reduction is due to the oxidation of Cu2+ to Cu(II)O, which weakens the affinity for chloridazon, we checked the XPS spectrum of the Cu2p3/2 core level region of thermally regenerated Cu2+@POSS_SWy-2, as shown in Fig. 6(d). The spectrum clearly testifies to an important increase of Cu(II)O after the heat treatment.32,33 Despite this decrease in adsorption capacity after recycling, Cu2+@POSS_SWy-2 can be considered as a potential adsorbent for chloridazon and its metabolites.
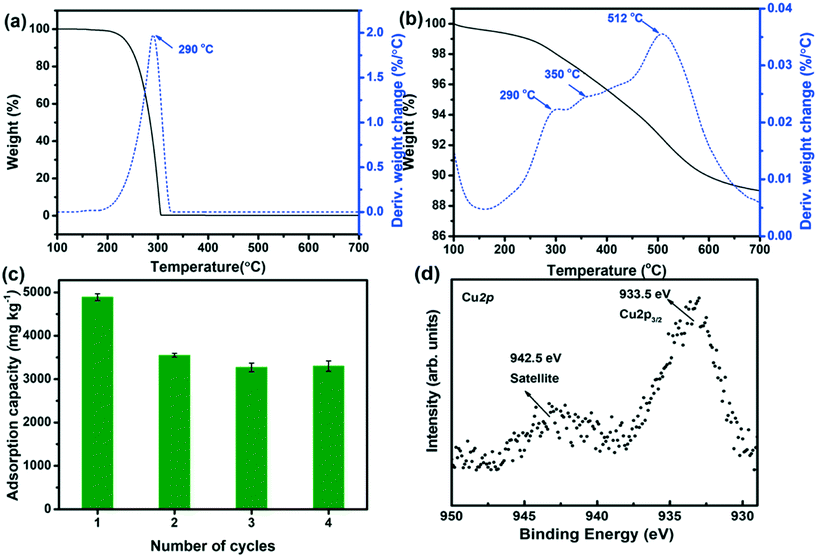 |
| Fig. 6 Thermogravimetric analysis (TGA) and differential thermal analysis (DTA) data for (a) chloridazon and (b) Cu2+@POSS_SWy-2 + chloridazon, and (c) adsorption capacity for chloridazon of Cu2+@POSS_SWy-2 after an increasing number of thermal regeneration cycles. (d) X-ray photoelectron spectrum of the Cu2p3/2 core level region of thermally regenerated Cu2+@POSS_SWy-2. | |
Conclusions
We established a controllable and reproducible synthesis method for a new adsorbent of chloridazon and its metabolites, namely smectite clay pillared with copper complexed polyhedral oligosilsesquioxane. The successful intercalation of Cu2+ complexed POSS into the interlayer space of the clay was confirmed by FTIR, XRD and high-resolution TEM. X-ray photoelectron spectroscopy as well as thermogravimetric and differential thermal analysis illustrated the type of interaction between the host materials and the intercalated molecules, and also shed light on the intercalation yield. Porosimetry measurements revealed that pillared structures are created and gave the specific surface area of the hybrid nanostructures. The hybrid nanostructure obtained by intercalation of Cu2+@POSS into the smectite clay showed a four times higher adsorption capacity for chloridazon, and a two times higher adsorption capacity for its metabolites, desphenyl-chloridazon and methyl-desphenyl-chloridazon, than the pristine SWy-2. This enhanced performance is attributed to the high specific surface area and the affinity of copper ions for nitrogen-containing herbicides. Cu2+@POSS_SWy-2 maintains an excellent adsorption capacity even after several thermal regeneration cycles. These findings show the potential of Cu2+@POSS_SWy-2 as a relatively low cost material for herbicide waste remediation.
Author contributions
D. Gournis and P. Rudolf designed the experiments and finalized the manuscript. F. Yan conducted all the experiments and wrote the draft of the manuscript. K. Spyrou and E. Thomou participated in the synthesis, S. Kumar participated in the XPS experiments and in the analysis of the XPS data, H. Cao and Y. Pei participated in the SEM experiments, and M. C. A. Stuart conducted the TEM experiments and participated in the analysis of the data. All authors discussed the results and commented on the manuscript.
Conflicts of interest
There are no conflicts to declare.
Acknowledgements
F. Y. thanks the China Scholarship Council (CSC No. 201704910930) and the University of Groningen for support for his PhD studies. E. T. was supported by the Hellenic Foundation for Research and Innovation (HFRI) and the General Secretariat for Research and Technology (GSRT), under the HFRI PhD Fellowship grant (GA. no. 1829). This work was supported by the Advanced Materials research program of the Zernike National Research Centre under the Bonus Incentive Scheme of the Dutch Ministry for Education, Culture and Science.
Notes and references
- H. Yang, R. Bain, J. Bartram, S. Gundry, S. Pedley and J. Wright, Water safety and inequality in access to drinking-water between rich and poor households, Environ. Sci. Technol., 2013, 47, 1222–1230 CrossRef CAS PubMed.
- J. Wu and Z. Sun, Evaluation of Shallow Groundwater Contamination and Associated Human Health Risk in an Alluvial Plain Impacted by Agricultural and Industrial Activities, Mid-west China, Exposure Health, 2016, 8, 311–329 CrossRef CAS.
- D. Lapworth, N. Baran, M. Stuart and R. Ward, Emerging organic contaminants in groundwater: a review of sources, fate and occurrence, Environ. Pollut., 2012, 163, 287–303 CrossRef CAS PubMed.
- T. Reemtsma, L. Alder and U. Banasiak, Emerging pesticide metabolites in groundwater and surface water as determined by the application of a multimethod for 150 pesticide metabolites, Water Res., 2013, 47, 5535–5545 CrossRef CAS PubMed.
- E. Jakiene, V. Spruogis, A. Dautarte, K. Romaneckas and D. Avizienyte, The bio-organic nano fertilizer improves sugar beet photosynthesis process and productivity, Zemdirbyste, 2015, 102, 141–146 CrossRef.
- G. Buttiglieri, M. Peschka, T. Frömel, J. Müller, F. Malpei, P. Seel and T. P. Knepper, Environmental occurrence and degradation of the herbicide n-chloridazon, Water Res., 2009, 43, 2865–2873 CrossRef CAS.
- GWD, EU. Directive 2006/118/EC of the European Parliament and of the Council of 12 December 2006 on protection of groundwater against pollution and deterioration. O. J. L, 2006, vol. 372, pp. 19–31.
- A. Schuhmann, O. Gans, S. Weiss, J. Fank, G. Klammler, G. Haberhauer and M. H. Gerzabek, A long-term lysimeter experiment to investigate the environmental dispersion of the herbicide chloridazon and its metabolites-comparison of lysimeter types, J. Soils Sediments, 2016, 16, 1032–1045 CrossRef.
- A. Alsbaiee, B. J. Smith, L. Xiao, Y. Ling, D. E. Helbling and W. R. Dichtel, Rapid removal of organic micropollutants from water by a porous β-cyclodextrin polymer, Nature, 2016, 529, 190 CrossRef CAS PubMed.
-
F. Bergaya and G. Lagaly. General introduction: clays, clay minerals, and clay science, in Handbook of clay science: Developments in clay science, 2006, vol. 1, pp. 1–18 Search PubMed.
- D. Carroll, Ion exchange in clays and other minerals, Geol. Soc. Am. Bull., 1959, 70, 749–779 CrossRef CAS.
- M. Auta and B. Hameed, Modified mesoporous clay adsorbent for adsorption isotherm and kinetics of methylene blue, Chem. Eng. J., 2012, 198, 219–227 CrossRef.
- T. Pinnavaia, Intercalated clay catalysts, Science, 1983, 220, 365–371 CrossRef CAS PubMed.
- C. W. Chiu, T. K. Huang, Y. C. Wang, B. G. Alamani and J. J. Lin, Intercalation strategies in clay/polymer hybrids, Prog. Polym. Sci., 2014, 39, 443–485 CrossRef CAS.
- B. Krishna, D. Murty and B. J. Prakash, Surfactant-modified clay as adsorbent for chromate, Appl. Clay Sci., 2001, 20, 65–71 CrossRef CAS.
- J. Luo, W. Zhang, H. Yuan, C. Jin, L. Zhang, H. Huang, C. Liang, Y. Xia, J. Zhang and Y. Gan, Pillared structure design of MXene with ultralarge interlayer spacing for high-performance lithium-ion capacitors, ACS Nano, 2017, 11, 2459–2469 CrossRef CAS.
- D. M. Fox, P. H. Maupin, R. H. Harris, J. W. Gilman, D. V. Eldred, D. Katsoulis, P. C. Trulove and H. C. De Long, Use of a polyhedral oligomeric silsesquioxane (POSS)-imidazolium cation as an organic modifier for montmorillonite, Langmuir, 2007, 23, 7707–7714 CrossRef CAS PubMed.
- F. Carniato, C. Bisio, G. Gatti, E. Boccaleri, L. Bertinetti, S. Coluccia, O. Monticelli and L. Marchese, Thermal stability
of polystyrene nanocomposites from improved thermally stable organoclays, Angew. Chem., Int. Ed., 2009, 48, 6059–6061 CrossRef CAS PubMed.
- G. Potsi, A. Rossos, A. Kouloumpis, M. Antoniou, K. Spyrou, M. A. Karakassides, D. Gournis and P. Rudolf, Carbon nanostructures containing polyhedral oligomeric silsesquioxanes (POSS), Curr. Org. Chem., 2016, 20, 662–673 CrossRef CAS.
- H. He, B. Li, J. Dong, Y. Lei, T. Wang, Q. Yu, Y. Feng and Y. Sun, Mesostructured nanomagnetic polyhedral oligomeric silsesquioxanes (POSS) incorporated with dithiol organic anchors for multiple pollutants capturing in wastewater, ACS Appl. Mater. Interfaces, 2013, 5, 8058–8066 CrossRef CAS PubMed.
- G. Potsi, A. K. Ladavos, D. Petrakis, A. P. Douvalis, Y. Sanakis, M. S. Katsiotis, G. Papavassiliou, S. Alhassan, D. Gournis and P. Rudolf, Iron-substituted cubic silsesquioxane pillared clays: Synthesis, characterization and acid catalytic activity, J. Colloid Interface Sci., 2018, 510, 395–406 CrossRef CAS PubMed.
- D. Gournis, A. Lappas, M. Karakassides, D. Többens and A. Moukarika, A neutron diffraction study of alkali cation migration in montmorillonites, Phys. Chem. Miner., 2007, 35, 49–58 CrossRef.
- D. Petridis, D. Gournis and M. Karakassides, The Chemistry of Organofunctionalized Silicon Cubanes in Swelling Smectites, Mol. Cryst. Liq. Cryst., 2006, 311, 345–350 CrossRef.
- P. Olivera-Pastor, P. Mairles-Torres, E. Rodriguez-Castellon and A. Jimenez-Lopez, Nanostructured inorganically pillared layered metal (IV) phosphates, Chem. Mater., 1996, 8, 1758 CrossRef CAS.
- J. Landers, G. Gennady and N. Alexander, Density functional theory methods for characterization of porous materials, Colloids Surf., A, 2013, 437, 3–32 CrossRef CAS.
- O. Ivashenko, H. Logtenberg, J. Areephong, A. Coleman, P. Wesenhagen, E. Geertsema, N. Heureux, B. L. Feringa, P. Rudolf and W. Browne, Remarkable stability of high energy conformers in self-assembled monolayers of a bistable electro-and photoswitchable overcrowded alkene, J. Phys. Chem. C, 2011, 115, 22965–22975 CrossRef CAS.
- T. L. Barr, An XPS study of Si as it occurs in adsorbents, catalysts, and thin films, Appl. Surf. Sci., 1983, 15, 1–35 CrossRef CAS.
- M. A. Karakassides, D. Gournis and D. Petridis, An infrared reflectance study of Si–O vibrations in thermally treated alkali-saturated montmorillonites, Clay Miner., 1999, 34, 429–438 CrossRef CAS.
- K. Spyrou, G. Potsi, E. K. Diamanti, X. Ke, E. Serestatidou, I. I. Verginadis, A. P. Velalopoulou, A. M. Evangelou, Y. Deligiannakis, G. Van Tendeloo, D. Gournis and P. Rudolf, Towards novel multifunctional pillared nanostructures: Effective intercalation of adamantylamine in graphene oxide and smectite clays, Adv. Funct. Mater., 2014, 24, 5841–5850 CrossRef CAS.
-
K. G. Theng, The Chemistry of Clay-Organic Reactions, Adam Hilger, London, 1974, p. 133 Search PubMed.
- G. Balomenou, P. Stathi, A. Enotiadis, D. Gournis and Y. Deligiannakis, Physicochemical study of amino-functionalized organosilicon cubes intercalated in montmorillonite clay: H-binding and metal uptake, J. Colloid Interface Sci., 2008, 325, 74–83 CrossRef CAS.
- J. Huo, X. Liu, X. Li, L. Qin and S. Z. Kang, An efficient photocatalytic system containing Eosin Y, 3D mesoporous graphene assembly and CuO for visible-light-driven H2 evolution from water, Int. J. Hydrogen Energy, 2017, 42, 15540–15550 CrossRef CAS.
- S. Poulston, P. M. Parlett, P. Stone and M. Bowker, Surface Oxidation and Reduction of CuO and Cu2O Studied Using XPS and XAES, Surf. Interface Anal., 1996, 24, 811–820 CrossRef CAS.
- R. Mansa, L. Dzene, A. Quintela, F. Rocha and C. Detellier, Preparation and characterization of novel clay/scleroglucan nanocomposites, Appl. Clay Sci., 2016, 126, 235–244 CrossRef CAS.
- I. Weiss, C. Muth, R. Drumm and H. O. K. Kirchner, Thermal decomposition of the amino acids glycine, cysteine, aspartic acid, asparagine, glutamic acid, glutamine, arginine and histidine, BMC Biophys., 2018, 11, 1–2 CrossRef PubMed.
- F. Zhao, C. Wan, X. Bao and B. Kandasubramanian, Modification of montmorillonite with aminopropylisooctyl polyhedral oligomeric silsequioxane, J. Colloid Interface Sci., 2009, 333, 164–170 CrossRef CAS PubMed.
- O. Cubuk, C. Bulent, T. Cihan, C. Fatih, S. Gokhan, T. Ahmet and S. Erdal, Structural characterization of hexadecyl-trimethylammonium-smectite composites and their potentiometric electrode applications, Appl. Surf. Sci., 2015, 338, 99–112 CrossRef CAS.
- K. Kaneko, Determination of pore size and pore size distribution: 1. Adsorbents and catalysts, J. Membr. Sci., 1994, 96, 59–89 CrossRef CAS.
- D. Chen, G. Du, Q. Zhu and F. Zhou, Synthesis and characterization of TiO2 pillared montmorillonites: application for methylene blue degradation, J. Colloid Interface Sci., 2013, 409, 151–157 CrossRef CAS PubMed.
- P. Behrens, Mesoporous inorganic solids, Adv. Mater., 1993, 5, 127–132 CrossRef CAS.
- M. E. R. Jalil, R. Vieira, D. Azevedo, M. Baschini and K. Sapag, Improvement in the adsorption of thiabendazole by using aluminum pillared clays, Appl. Clay Sci., 2013, 71, 55–63 CrossRef.
- M. Opanasenko, W. Parker Jr, M. Shamzhy, E. Montanari, M. Bellettato, M. Mazur, R. Millini and J. Čejka, Hierarchical hybrid organic–inorganic materials with tunable textural properties obtained using Zeolitic-layered precursor, J. Am. Chem. Soc., 2014, 136, 2511–2519 CrossRef CAS PubMed.
- C. Giles, D. Smith and A. Huitson, A general treatment and classification of the solute adsorption isotherm. I. Theoretical, J. Colloid Interface Sci., 1974, 47, 755–765 CrossRef CAS.
- N. Daneshvar, S. Aber, A. Khani and M. Rasoulifard, Investigation of adsorption kinetics and isotherms of imidacloprid as a pollutant from aqueous solution by adsorption onto industrial granular activated carbon, J. Food, Agric. Environ., 2007, 5, 425 CAS.
- D. Drolet, D. Manuta, A. Lees, A. Katnani and G. Coyle, FT-IR and XPS study of copper (II) complexes of imidazole and benzimidazole, Inorg. Chim. Acta, 1988, 146, 173–180 CrossRef CAS.
- J. Ci, W. Tu, W. Uen, S. Lan, J. Zeng, T. Yang, C. Shen and J. Jhao, Chlorine-doped n-type cuprous oxide films fabricated by chemical bath deposition, J. Electrochem. Soc., 2014, 161, D321–D326 CrossRef CAS.
- T. Mondal, A. Bhowmick and R. Krishnamoorti, Chlorophenyl pendant decorated graphene sheet as a potential antimicrobial agent: synthesis and characterization, J. Mater. Chem., 2012, 22, 22481–22487 RSC.
- J. Lambert, Organic pollutant adsorption on clay minerals, Dev. Clay Sci., 2018, 9, 195–253 Search PubMed.
Footnote |
† Electronic supplementary information (ESI) available: XPS survey spectrum of Cu2+@POSS_SWy-2 and pore size distribution of SWy-2, POSS_SWy-2 and Cu2+@POSS_SWy-2 determined by the non-local density functional theory; N1s XPS spectrum of Cu2+@POSS_SWy-2 after adsorption of chloridazon. See DOI: 10.1039/c9en00974d |
|
This journal is © The Royal Society of Chemistry 2020 |