Biodegradation kinetics testing of two hydrophobic UVCBs – potential for substrate toxicity supports testing at low concentrations†
Received
30th June 2020
, Accepted 3rd September 2020
First published on 4th September 2020
Abstract
The biodegradation kinetics of UVCB substances (unknown or variable composition, complex reaction products or biological materials) should be determined below the solubility limit to avoid experimental artefacts by the non-dissolved mixture. Recently, we reported delayed biodegradation kinetics of single petroleum hydrocarbons even at concentrations just below the solubility limit and attributed this to toxicity. The present study aimed to determine the concentration effect on biodegradation kinetics for constituents in two UVCBs, using surface water from a rural stream as the inoculum. Parallel biodegradation tests of diesel and lavender oil were conducted at concentrations just below the solubility limit and two orders of magnitude lower. The biodegradation kinetics of diesel oil constituents were generally similar at the two concentrations, which coincided with the stimulation of bacterial productivity (growth) at both concentrations, determined by [3H]leucine incorporation. By contrast, the biodegradation of lavender oil constituents was significantly delayed or even halted at the high test concentration. This was consistent with lavender oil stimulating bacterial growth at low concentration but inhibiting it at high concentration. The delayed biodegradation kinetics of lavender oil constituents at high concentration was best explained by mixture toxicity near the solubility limit. Consequently, biodegradation testing of hydrophobic UVCBs should be conducted at low, environmentally relevant concentrations ensuring that mixture toxicity does not affect the biodegradation kinetics.
Environmental significance statement
Biodegradation kinetics is a fundamental component of environmental risk and hazard assessment. However, biodegradation testing of complex mixtures is often limited by analytical and technical challenges. In this study, we apply a recently developed biodegradation platform to determine the biodegradation kinetics for constituents in two hydrophobic complex mixtures at two concentrations (just below the solubility limit and two orders of magnitude lower). The results demonstrate why it is important to test at low, non-toxic and fully dissolved concentrations, and show how this can be done when using advanced analytical methods.
|
Introduction
Biodegradation testing of substances of unknown or variable composition, complex reaction products or of biological materials (UVCB)1 is necessary, but poses major analytical and technical challenges.2 For risk assessment purposes such testing should be conducted below the solubility limit in order to avoid non-dissolved test compounds.3 However, for hydrophobic UVCB substances such as petroleum products and many essential oils, it can be challenging to establish a well-defined and reproducible initial mixture concentration and composition with all constituents fully dissolved.4 Furthermore, we recently reported delayed biodegradation kinetics of petroleum hydrocarbons tested individually at concentrations just below the solubility limit and attributed this to toxicity.5 While the toxicity of mixtures is widely studied, there are some crucial data and knowledge gaps related to the effect of test concentration and mixture toxicity on the biodegradation kinetics of chemicals in mixtures.
The chemical test concentration is important in biodegradation studies because at a low concentration a substance may serve as a substrate and stimulate microbial respiration and growth, and thus biodegradation, while at a higher concentration that same substance may be toxic and inhibit biodegradation. The link between the chemical test concentration and biodegradation kinetics is relatively well-studied for single chemicals.5–10 At very low concentrations of a single chemical (e.g. low ng L−1 range), biodegradation may be limited by substrate availability in the absence of other carbon sources.7,8 An increase in chemical concentration will stimulate the microbial metabolic activity and growth, but only up to a certain point.6,10 Increasing the concentration above this point will not increase the degradation activity, and eventually the substrate might reach a level where it becomes toxic.6,10 When conducting biodegradation tests with a complex mixture, however, many different interactions will take place. For instance, while biodegradation of a single chemical may be limited at very low concentrations,7,8 bacteria have been observed to subsist on a multitude of carbon substrates that were each present at minuscule concentrations.11 At the higher concentration end, hydrophobic organic chemicals (HOCs) typically exert baseline toxicity at concentrations above 1% of solubility, i.e. at chemical activity above 0.01.12 For mixtures of chemicals, antagonistic or synergistic interactions can result in the mixture toxicity being lower or higher than what would be expected based on concentration addition. The link between the chemical test concentration and biodegradation kinetics thus needs to be studied specifically for complex mixtures.
A fair amount of research has been conducted on aquatic biodegradation of petroleum products such as crude oil,13,14 gasoline,15–18 diesel oil18 and biodiesel.19 Many of these studies targeted oil spill situations and most were conducted at concentrations above the water solubility limit of the oil constituents. Prince et al.14 reviewed twenty-two papers published between 1995 and 2016 that studied biodegradation of dispersed oil in seawater and reported half-lives ranging from 1 to 276 days (some results were extrapolated beyond the study duration). They inferred that the main driver for this variability was the large difference in the initial oil concentration (between 2 and 10
000 mg L−1) and further derived that many of these studies must have contained hydrocarbon constituents in a free phase. When Prince et al.14 studied the degradation of dispersed crude oil in seawater at different concentrations (between approximately 2.5 and 2500 mg L−1) they observed that increasing the oil concentration significantly slowed the biodegradation. However, in all these tests some constituents were also present as oil droplets. When reviewing the literature, no studies were found on the effect of test concentration on the biodegradation kinetics of hydrophobic complex mixtures at concentrations below the solubility limit.
Primary biodegradation kinetics can be determined for individual chemicals in mixtures by specific chemical analysis (e.g. gas chromatography),20–22 and recent advancements in analytical chemistry have enabled biodegradation kinetics studies without labelled substances even in the sub μg L−1 concentration range.23,24 The present study applied a recently developed experimental and analytical platform for biodegradation kinetics testing of hydrophobic chemicals in defined mixtures.20,23 Primary biodegradation kinetics were determined for individual constituents in two complex mixtures, diesel oil and lavender oil, at two different concentrations. Passive dosing was used to set initial concentrations in a surface water inoculum, thus avoiding undissolved compounds or co-solvents.4 Automated headspace solid phase microextraction was applied directly on the test systems, which had the advantage of reduced test substance losses and an enhanced sensitivity for the most hydrophobic mixture constituents whereby the number of detectable mixture constituents was increased.
The mixture effect on bacterial productivity (growth) was determined by measuring the rates of [3H]leucine incorporation during the first nine days of the biodegradation experiment. Leucine incorporation is a widely used method25 to evaluate bacterial productivity and has, for example, been used to assess the toxicity of gasoline vapors to soil bacterial communities,26 and of antibiotics27,28 and surfactants29 to limnic bacterial communities. Most freshwater bacterial groups can take up leucine and incorporate it in proteins,25 and leucine incorporation is considered a sensitive assay for determining the toxic effects of chemicals in complex microbial communities.28,29
The aim of this study was to determine the concentration effect of diesel and lavender oil on the biodegradation kinetics of their constituents, and then to link this to the mixture effect on bacterial productivity.
Biodegradation tests were conducted at two test concentrations, (i) just below the solubility limit, i.e. within the range where baseline toxicity has been observed in microorganisms, and (ii) two orders of magnitude below the solubility limit. The working hypothesis was that the biodegradation of mixture constituents can be inhibited at high test concentrations due to mixture toxicity, resulting in a longer lag phase and half-life compared to the lower test concentrations.
Methods
Materials
Two UVCB substances were included in this study: Diesel Multi-Parameter Certified Reference Material (Paragon Scientific Ltd, UK) and Lavender Oil Barreme Type (CAS 84776-65-8, Givaudan, UK). The diesel oil was a distillate petroleum product consisting primarily of hydrocarbons with boiling points in the range of 172–360 °C. The lavender oil had nine constituents at a concentration above 1% in the mixture according to the manufacturer, namely linalyl acetate, linalool, beta-caryophyllene, cis-beta-ocimene, trans-beta-ocimene, terpin-1-en-4-ol, lavandulyl acetate, 1,8-cineole (eucalyptol) and 3-octanone, with linalyl acetate and linalool as the two main constituents, together making up 71% of the mixture. For the passive dosing setup, a translucent silicone rod (OD 3 mm, polydimethylsiloxane (PDMS)) without a whitening agent was ordered custom-made (Altec, UK). The rods were cleaned with ethyl acetate (purity ≥99.7%, Sigma-Aldrich, Denmark), ethanol (absolute, VWR International, Denmark) and ultrapure water as described in Hammershøj et al.4 Ultrapure water was produced with an Elga Purelab flex water system (Holm & Halby, Denmark). Silicone oil (CAS 63148-62-9, Sigma-Aldrich, Denmark) was used to make reference standards. Test systems for the biodegradation experiment were made with 20 mL amber glass autosampler vials closed with PTFE/silicone septa and gas-tight stainless-steel screw caps (Sigma-Aldrich, Denmark).
Preparation of passive dosing systems
Passive dosing from a loaded silicone rod was used to establish the initial mixture concentrations and compositions in the biodegradation experiment. The employed passive dosing method is described in detail in Hammershøj et al.4
In brief, a pre-cleaned silicone rod was loaded with the liquid chemical test mixture in one of two ways. At low loading levels (<5 g mixture per g silicone), a defined volume of the mixture was added to a silicone rod in a glass jar using a gastight Hamilton syringe. The glass jar was then closed and rolled horizontally for 48 h, which allowed the rod to absorb the added test mixture. At high loading levels, an excess amount of the mixture was added to a silicone rod in a glass jar using a gastight Hamilton syringe. The glass jar was then closed and rolled horizontally until a loading level was reached that corresponded to approximately 70% of saturation.4 The time that was required to reach this loading level was predetermined from a loading-kinetics curve made for each test mixture (ESI 1†). After loading, all rods were rinsed with ultrapure water and stored individually in 240 mL gas-tight amber glass bottles for 2 weeks until use.
Seven identical passive dosing systems (loaded rod in a 240 mL gas-tight amber glass bottle) were made for each of the four biodegradation tests (Table S1†). The dimensions of the passive dosing systems were chosen to ensure fast kinetics (high donor surface area to water volume ratio) and avoid donor depletion for all mixture constituents (high donor volume to water volume ratio, i.e. Vdonor/Vwater > 0.1 L/L for constituents with silicone to water partition coefficients, Ksilicone/water, > 100 L/L).4 Since the lavender oil included constituents with Ksilicone/water < 100 L/L (Table S7†), larger silicone donors were used in the passive dosing systems with lavender oil than with diesel oil. The diesel oil passive dosing systems were loaded at 0.010 g oil per g silicone (low loading) and 0.42 g oil per g silicone (high loading) and the lavender oil passive dosing systems were loaded at 0.010 g oil per g silicone (low loading) and 0.44 g oil per g silicone (high loading). Further details are given in ESI 1.†
Surface water inoculum
A surface water grab sample was collected on August 27, 2018 from a rural stream (Fønstrup stream, Northern Zealand, Denmark) with no direct agricultural, industrial or domestic inputs.30 The surface water sample was used as the inoculum in the biodegradation experiment within 15 h of sampling and without pretreatment. The water sample was stored in glass bottles, in the dark and at room temperature until use. The in situ water temperature, pH, oxygen content, content of nonvolatile organic carbon and heterotrophic plate count are listed in ESI 2.†
Experimental setup
The four biodegradation systems were prepared in the same way. The UVCBs were dosed into the test systems by adding 150 mL of surface water inoculum to each of the seven passive dosing systems and equilibrating by horizontal rolling for at least 1 h. Biotic test systems were made by transferring 15 mL of the dosed surface water inoculum from a passive dosing system (taken with a gastight Hamilton syringe through the pierced septum) to an empty 20 mL autosampler vial. Abiotic test systems were made by transferring 14.5 mL dosed surface water inoculum to a 20 mL autosampler vial containing 0.5 mL sodium azide (final NaN3 concentration 0.05 wt%). The vials were closed immediately. To enable analysis at seven time points, three pairs of biotic/abiotic test systems were prepared from each of the seven passive dosing systems. These three pairs constituted a set of test systems, which was later analyzed at the same sampling time and used as the replicate input for data analysis. The test systems (42 for each biodegradation test and 168 in total) were incubated horizontally on benchtop tube rollers (Ratek, Australia) at ∼30 rpm (no tilt), at a temperature of 20 ± 1 °C. Samples were collected for chemical analysis after 1, 4, 7, 10, 14, 21 and 28 days.
Three additional biotic test systems (15 mL dosed surface water inoculum in a 20 mL autosampler vial) from each passive dosing bottle and fifteen surface water controls (15 mL non-dosed surface water inoculum in a 20 mL autosampler vial) were also prepared. These test systems were used to measure the rates of [3H]leucine incorporation in growing bacteria during the first nine days of the biodegradation experiment relative to the non-dosed surface water inoculum. The test systems and surface water controls were incubated with the other test systems and sampled after 0 (only surface water controls), 1, 3 and 9 days.
All test systems had a 5 mL headspace to ensure aerobic conditions throughout the test, and for confirmation, the oxygen level was measured in separate test systems after 10, 21 and 29 days (n = 1, Table S3†).
Chemical analysis
Fully automated head-space solid phase microextraction (HS-SPME) was performed directly on the biotic and abiotic test systems using a PAL RSI 85 autosampler (CTC Analytics AG, Switzerland) mounted on a 5977A MSD GC coupled to a 7890B MS (Agilent technologies, USA). A 7 μm PDMS fiber (Supelco, USA) was used for the HS-SPME sampling, which was conducted at 35 °C for 60 min at an agitation speed of 250 rpm. Analytes were thermally desorbed from the fiber in the injection port for 10 min at 250 °C. The inlet was operated in a splitless mode (diesel oil at low concentration and lavender oil at low concentration), with a 1
:
1 split (diesel oil near saturation) or with a 25
:
1 split (lavender oil near saturation), and with a septum purge flow of 3 mL min−1. Post-conditioning of the fiber was performed for 10 min at 320 °C. Separation was obtained on a 122-5562UI DB-5 ms Ultra Inert column (Agilent) of 60 m length, 250 μm inner diameter and 0.25 μm film thickness. Helium was used as the carrier gas at a flow rate of 1.2 mL min−1. The GC oven temperature was 40 °C for the 10 min desorption followed by a ramp of 10 °C min−1 to 100 °C, 1.5 °C min−1 to 200 °C and 20 °C min−1 to 320 °C. The total GC cycle time was 89 min. All samples were analyzed by full scan MS from 50 to 500 amu with a gain factor of 3. 20 mL autosampler vials containing 15 mL ultrapure water or 15 mL poisoned surface water inoculum (0.05 wt% NaN3) were included in each run as blanks. Furthermore, duplicate 2 mL samples of 0.01 g g−1 (diesel) or 0.01 g g−1 (lavender) oil in PDMS silicone oil in 20 mL autosampler vials served as reference standards that were used to check for differences in instrument sensitivity and retention time drifts between runs.
Biodegradation data processing and quality assurance
Full scan data files obtained with GC/MSD ChemStation (Agilent Technologies) were exported to MassHunter Quantitative Data Analysis for GCMS and LCMS (Version B.09.00/Build 9.0.647.0, Agilent Technologies). Mixture constituents were found by deconvolution, and the extracted base ion peak area of each analyte was used in further data analysis. All integrations were manually checked in MassHunter. Additionally, the retention time (Rt) and mass-charge (m/z) ratio of the base ion were manually checked for each mixture constituent using MSD ChemStation Enhanced Data Analysis (Agilent Technologies). Peaks with retention times shorter than 16 min (100 °C) were considered background noise and omitted from the analysis.
The detection limit and quantification limit were selected as ten times and twenty times the root-mean-square signal-to-noise ratio, calculated using MSD ChemStation Enhanced Data Analysis for the extracted ion chromatogram. Constituents below the detection limits were omitted from further analysis. If a constituent was below the quantification limit at the low test concentration but not at the high test concentration, its biodegradation kinetics were only determined at the high test concentration. One diesel oil constituent was omitted due to an elevated blank response. One abiotic vial (lavender at high concentration on day 2) had leaked, and the corresponding biotic/abiotic pair was thus excluded.
The background concentrations of mixture constituents in the surface water inoculum were below 1% of the lowest test concentration for all diesel oil and lavender oil constituents. This was determined by comparing levels in poisoned surface water with levels in dosed abiotic test vials analyzed in the same GC-MS run.
After deconvolution, tentative identifications were done by library spectral search in NIST 17 using MassHunter Unknown Analysis (Version B.09.00/Build 9.0.647.0, Agilent Technologies). The spectral matches were manually checked and the suggestion with the highest match factor was generally selected. In a few cases the second or third suggestion was selected due to better spectral match based on the visual comparison of spectra. Only tentative identifications with a match factor >80% are reported. A list of the selected mixture constituents is given for the two test mixtures in ESI S3.†
Biodegradation data analysis
The extracted ion peak area obtained by GC-MS analysis for a mixture constituent in a biotic test system (Abiotic) was divided by the peak area from an abiotic test system (Aabiotic). The ratio between peak areas in these two systems was termed Crelative (eqn (1)). |  | (1) |
Three values of Crelative were calculated for each time point and used as replicate input data for further analysis. A first-order degradation model with a lag phase (eqn (2)) was fitted to these data to obtain estimates on the lag phase (tlag) and (pseudo) first-order degradation rate constant in the test system (ksystem) for each mixture constituent. This was done by least-squares in GraphPad Prism v. 8.2.1(411) with the constraints tlag ≥ 0, ksystem ≥ 0 and Crelative(0) = 1, and data were not weighted. As biodegradation proceeded, the level in the biotic test systems dropped below the quantification limit. This is important information but should not be given too much weight in the fitting. Thus, a maximum of two data points with an average Crelative below 0.01 (1% of initial concentration) were included for each constituent. | 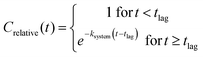 | (2) |
For the purpose of this study, the single first-order model was deemed adequate by the evaluation of the visual fit, but we acknowledge that other models may give a better fit to the data, and that the underlying process may not be truly first-order at all test concentrations.
The test system half-life (T½) for a constituent was calculated as ln(2)/ksystem. The test system biodegradation half-time (DegT50) was calculated as the sum of the lag phase and half-life. Biodegradation kinetics parameters (tlag, ksystem, T½ and DegT50) were reported when at least two measurements were made during the visual degradation phase (10% < Crelative < 90%) and the goodness of fit was R2 > 0.8. Otherwise only DegT50 was reported.
Mass balance calculations
Based on the tentative identifications of mixture constituents, mass balance calculations were performed to illustrate how mixture constituents were distributed between silicone, water and air in the passive dosing systems, and between water and air in the biodegradation test systems. The governing partition coefficients were calculated using the UFZ-LSER database31 and applied in simple mass balance calculations for the respective systems. These calculations confirmed a negligible depletion of mixture constituents from the silicone rod in the passive dosing systems. Details and results are shown in ESI 4.†
[3H]leucine incorporation (bacterial productivity)
Bacterial productivity (growth) was determined in biotic test systems and in parallel surface water controls (non-dosed surface water) after 0 (only controls), 1, 3, and 9 days.29,32 For each measurement point, 1.55 mL was transferred from triplicate biotic test systems and triplicate surface water controls (five on day 0) into 2 mL centrifuge tubes with a gastight Hamilton syringe. A mixture of [3H]leucine (2.59 TBq mmol−1, 37 MBq mL−1, Amersham, Hillerød, Denmark) and unlabeled L-leucine was added to each tube to reach 6 kBq per microtube and a total leucine concentration of 100 nM. The tubes were then vortexed and incubated for 45 minutes at room temperature. Incubations were terminated by adding 160 μL ice-cold 50% trichloroacetic acid (TCA, final concentration 5%), vortexed thoroughly and preserved at 4 °C for up to 10 days. Killed controls were made by adding TCA to non-dosed surface water prior to the addition of the [3H]leucine working solution. Incorporated [3H]leucine was separated from the unincorporated fraction through a number of centrifugation and washing steps and quantified by scintillation counting as described in Bååth et al.33 The average scintillation count of the killed controls was used for blank subtraction. The scintillation counts for day 1, 3 and 9 samples were normalized relative to the average scintillation count for the day-0 surface water control samples (n = 5) and plotted against time.
Results and discussion
[3H]leucine incorporation (bacterial productivity)
Bacterial growth dynamics were recorded during the first nine days of the biodegradation test (Fig. 1). Overall, the bacterial productivity increased during the first 24 h after which the productivity slowly decreased over time. This is a characteristic pattern when incubating naturally occurring microorganisms without continuous substrate supply. [3H]leucine incorporation was stimulated by the diesel oil at both test concentrations relative to the surface water controls (Fig. 1A), with the exception that the [3H]leucine incorporation rates were similar at the low diesel oil concentration and in the surface water controls on day nine. For lavender oil, the [3H]leucine incorporation was stimulated at the low test concentration, whereas a strong inhibition was observed at the high test concentration (>99% inhibition after 24 h and >95% after 9 days) (Fig. 1B).
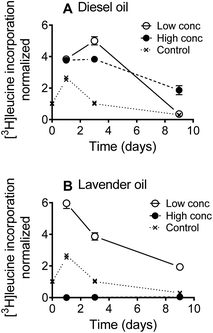 |
| Fig. 1 [3H]leucine incorporation dynamics during the first nine days of the biodegradation experiment for diesel and lavender oil, respectively. All [3H]leucine incorporation rates were normalized relative to the average rate measured on day 0 for the control (n = 5). ‘Low conc’ = low concentration biodegradation test, ‘High conc’ = high concentration biodegradation test, and ‘Control’ = surface water controls without diesel and lavender oil. Error bars represent the standard error of the mean, n = 3. | |
Biodegradation kinetics for diesel oil constituents
Biodegradation kinetic curves were fitted to experimental data for 104 diesel oil constituents, and for 62 constituents data were obtained at both test concentrations (Fig. S3†). Biodegradation curves are shown in Fig. 2 for the four diesel oil constituents that had the best fit (R2) at the low concentration. Kinetic parameters (lag phase, first-order rate constant, half-life and half-time) obtained by model fits are listed in Table S8.†
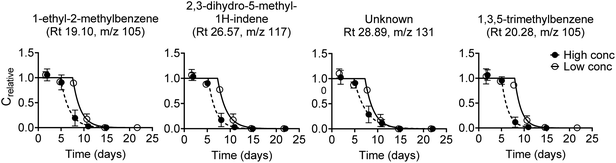 |
| Fig. 2 First-order degradation model fitted to experimental biodegradation data for four diesel oil constituents at the low and the high test concentration. The four constituents were selected based on the best fit (R2, Fig. S6†) at the low test concentration. Note that compound identifications are tentative. Error bars represent the standard error of the mean, n = 3. | |
All but four diesel oil constituents were fully degraded within the 28 day test duration. These four constituents (Rt 45.55, m/z 132; Rt 45.85, m/z 159; Rt 45.98, m/z 159; and Rt 47.53, m/z 159) were 70–90% degraded at the end of the test at the high concentration while they were all below the quantification limit at the low concentration. Degradation kinetics were rather similar for most of the 100 diesel oil constituents that were fully degraded within 28 days. Lag phases were between 1 and 9 days (low test concentration) or 1 and 7 days (high test concentration), followed by degradation with half-lives of 0.5 to 12 days (ESI 5†). The observed degradation pattern for the diesel oil constituents in Fig. 2 was thus generally consistent with the degradation of the other diesel oil constituents.
Biodegradation kinetics for lavender oil constituents
Experimental data were collected for 32 lavender oil constituents. For 22 of these 32 constituents, there were distinct abiotic transformations (dissipation or formation) at one or both test concentrations. This is important information, but the test method applied in this study was not aimed at determining abiotic transformation and consequently the related biodegradation data were excluded. Experimental biodegradation data are thus only shown for 10 of the 32 constituents (Fig. S5†), and data were obtained at both test concentrations for nine of these constituents. Biodegradation curves are shown in Fig. 3 for the four lavender oil constituents that had the best fit (R2) at the low concentration. Kinetic parameters obtained by model fits are listed in Table S9.†
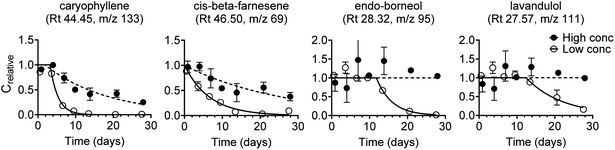 |
| Fig. 3 First-order degradation model fitted to experimental biodegradation data for four lavender oil constituents at the low and the high test concentration. The four constituents were selected based on the best fit (based on R2, Fig. S6†) at the low test concentration. Compound identifications are tentative. Error bars represent the standard error of the mean, n = 3. | |
Biodegradation kinetics (i.e. lag phase and degradation half-life or half-time) varied notably between the lavender oil constituents (Fig. 3 and S5†). Two constituents (tentatively identified as caryophyllene and cis-beta-farnesene) were fully degraded at the low concentration and partially degraded at the high concentration after 28 days. Another three constituents were fully degraded (tentative id. endo-borneol and 3-octanone) or partly degraded (tentative id. lavandulol, ∼84%) at the low concentration while there were no visible signs of degradation at the high concentration after 28 days. For the remaining five constituents, there were no visible signs of biodegradation at either test concentration within the 28 day test duration.
The two main constituents in the lavender oil were linalyl acetate and linalool. In this study, the lavender oil constituent that was tentatively identified as linalyl acetate rapidly dissipated from both abiotic and biotic test systems at both the high and the low test concentration (data not shown), which can be explained by its ability to undergo hydrolysis within a day.34 The constituent that was tentatively identified as linalool was not visibly degraded within the 28 day test duration at any of the two test concentrations (Fig. S5†) even though linalool is listed as readily biodegradable under REACH.35 A similar discrepancy was observed for the constituent tentatively identified as eucalyptol, which was not visibly degraded in this study but is listed as readily biodegradable in its REACH dossier.36 No conclusions could be drawn on the reason for this difference, but potential explanations include a limited number of competent degraders in the used surface water, or mixture effects such as a preferential substrate consumption leading to sequential degradation, or competitive inhibition.
The variations in biodegradation kinetics between the lavender oil constituents could be due to the larger diversity in the chemical structure compared to the diesel oil constituents, since some chemical structures are inherently more recalcitrant to biodegradation than others. It could also be hypothesized, that the different chemical structures are degraded by different types of microorganisms, some of which were present whereas others were not.
Concentration effects on constituent specific degradation
To facilitate the comparison between the biodegradation tests with different initial concentrations, the biodegradation half-time (DegT50) for a given diesel oil or lavender oil constituent in the low concentration test was plotted against DegT50 for the same constituent in the high concentration test (Fig. 4). The figure only includes constituents for which degradation kinetics were determined at both high and low test concentrations, and where the first-order degradation model fit had R2 > 0.8. The four diesel oil constituents that were only partly degraded within the 28 day test duration are not included in the plot as these constituents were below the quantification limit at the low test concentration.
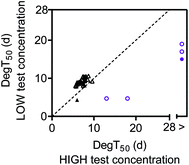 |
| Fig. 4 Biodegradation half-times (DegT50 = lag phase + half-life) for constituents in diesel oil (▲) or lavender oil ( ) at low test concentration vs. at high test concentration. Open symbols indicate that one of the DegT50's was based on a fit with less than 2 measured data points during the visual degradation phase. ‘>’ = Degradation not initiated within the test duration. A dotted 1 : 1 line is included as a visual reference. | |
For the diesel oil constituents, the DegT50's were overall similar at the two test concentrations though slightly longer at the low concentration (Fig. 4). This was attributable to longer lag phases at the low test concentration (Fig. S4† and 2). Once the degradation was initiated, the first-order biodegradation half-lives were similar at low and high test concentrations (Fig. S4†). The similar degradation kinetics are consistent with the initial stimulation of [3H]leucine incorporation observed at both test concentrations (Fig. 1A).
For lavender oil, the DegT50's varied notably between the lavender oil constituents and between the two test concentrations (Fig. 4). The high lavender oil test concentration delayed the biodegradation kinetics for all constituents that were degraded at the lower test concentration. This increase in the degradation half-time at the higher test concentration could be due to growth-linked kinetics as described by the Monod equation.37 A lag phase represents processes such as adaptation and growth of degrading microorganisms, and a longer apparent lag phase can occur when a higher bacterial density is needed to degrade a higher substrate concentration. However, the [3H]leucine incorporation rates showed a near-complete inhibition of bacterial productivity (growth) at the high lavender oil concentration (Fig. 1B). Thus, the delayed biodegradation kinetics near the solubility limit were more likely due to toxicity. In a recent study, Trac et al.38 observed that the toxicity of both the diesel and lavender oil towards Daphnia magna was within the baseline toxicity range but with a higher toxicity of lavender oil compared to diesel oil, and in general, many types of lavender oil are known to have antimicrobial properties.39 This is consistent with the strong inhibition of microbial growth at the high test concentration of lavender oil observed in this biodegradation study. Some biodegradation still occurred at the high test concentration for the constituents tentatively identified as caryophyllene and cis-beta-farnesene. This pattern, with a decoupling of growth and biodegradation, has also been observed for microorganisms in soil, where an exposure to gasoline vapors at toxic levels led to a decoupling between microbial growth and respiration.26
The near absence of a concentration effect for the diesel oil constituents might be explained by the surface water inoculum containing degrading microorganisms that were not very sensitive to the diesel oil,40 which is not unlikely given that hydrocarbons are ubiquitous in the aquatic environment due to their many diffuse sources.41
Implications for biodegradation testing of complex mixtures
This study shows that biodegradation tests with UVCB substances should ideally be conducted at low concentrations to avoid potential substrate toxicity. There can, however, be a trade-off between testing at low and non-toxic concentrations, and having high enough concentrations in the test systems to detect a large number of mixture constituents, especially for complex mixtures with a large number of minor constituents. The exact composition of the two tested UVCBs was unknown, and biodegradation kinetics could not be determined for all mixture constituents, but biodegradation data were generally obtained for a higher number of constituents at the high than at the low test concentration. The lowest test concentration used in this study was still higher than what can be expected in many aquatic environments due to natural distribution processes. Given sufficient analytical sensitivity, future studies could address the impact on biodegradation kinetics when testing at even lower chemical concentrations.
Analytical challenges and technical issues are some of the main limitations to biodegradation testing of UVCB substances.2,42 In this study, the automated HS-SPME-GC/MS method used directly on the test systems ensured minimal losses and low detection limits, which made it possible to obtain primary biodegradation kinetics for many individual, and potentially diverse, mixture constituents even at the low test concentration. The method is thus highly useful for determining primary biodegradation kinetics of individual mixture constituents, whereas it cannot directly be used to determine how much of the original mixture has been degraded in total. This is because the enrichment into the SPME fiber coating and the sensitivity of the MS detector are constituent specific, which results in chromatograms that are not directly representative of the mixture composition in the aqueous phase. Furthermore, the SPME fiber is only sensitive to hydrophobic constituents, and more polar degradation products may therefore not be detected. The total and ultimate biodegradation (mineralization) of a complex mixture can be determined by measuring a non-specific analytical parameter such as CO2 production.42 However, determining the overall mineralization of a mixture will not provide information on potentially persistent constituents, even if a pass criterion43 like 60% of the theoretical CO2 production is reached. Future biodegradation testing strategies for UVCB substances may thus require a combination of specific and non-specific approaches.
A focal feature of the partitioning-based biodegradation platform used in this study is the consistent pairing of biotic and abiotic test vials. For some lavender oil constituents this pairing was insufficient to separate the biodegradation from other dissipation processes. Many essential oil constituents can be converted into each other through abiotic transformations such as hydrolysis, autoxidation and isomerization processes, which can occur rapidly even at room temperature.44 Only a few studies are currently available on the biodegradation of essential oils or essential oil constituents,45 and this is thus an area where further research is needed.
Conclusion
This study was initiated to investigate the effect of test concentration on the primary biodegradation kinetics of constituents in two hydrophobic complex mixtures, diesel oil and lavender oil, and couple this to the mixture effect on bacterial productivity. Diesel oil was observed to stimulate the bacterial productivity at both low and high test concentrations, and no marked effect of increasing the test concentration was observed on the biodegradation kinetics of the constituents. Lavender oil, on the other hand, stimulated the bacterial productivity at the low concentration but inhibited the bacterial productivity at the high test concentration. This coincided with a delayed and limited biodegradation that can best be explained by toxicity near the solubility limit. The hypothesis that the biodegradation kinetics of mixture constituents can decrease at high concentration (near saturation) due to mixture toxicity was thus confirmed for lavender oil, but not for diesel oil.
The observations of this study show the potentially complex relationship between the concentration of a complex mixture of substrates and complex microbial communities, and demonstrate that it is essential to control and understand the effect of the test concentration in biodegradation testing of UVCB substances. Ideally, all mixture constituents should be fully dissolved and below toxic levels when conducting biodegradation studies with complex mixtures for environmental risk assessment purposes, given that this is the case in most aquatic environments. The analytical methods that are widely available today make it possible to test even hydrophobic chemicals orders of magnitude below their solubility limit, and we thus advocate that the persistence and biodegradability of UVCB substances should be tested at low concentrations more similar to those that are found in the relevant aquatic environments.
Conflicts of interest
There are no conflicts to declare.
Acknowledgements
We are grateful to Hanne Bøggild for technical assistance in the laboratory and to Karen Jenner, Delina Lyon, Marc Fernandez, Sandrine Deglin and Aaron Redman for their comments on the draft manuscript. This study was mainly funded by Concawe and the Research Institute for Fragrance Materials (RIFM) with additional funding from CEFIC LRI (ECO 42).
References
-
ECHA, What is a substance?, https://echa.europa.eu/en/support/substance-identification/what-is-a-substance, accessed Jun 4 2020 Search PubMed.
- D. Salvito, M. Fernandez, K. Jenner, D. Y. Lyon, J. de Knecht, P. Mayer, M. MacLeod, K. Eisenreich, P. Leonards and R. Cesnaitis,
et al., Improving the Environmental Risk Assessment of Substances of Unknown or Variable Composition, Complex Reaction Products, or Biological Materials (UVCBs), Environ. Toxicol. Chem., 2020 DOI:10.1002/etc.4846.
- H. Birch, A. D. Redman, D. J. Letinski, D. Y. Lyon and P. Mayer, Determining the Water Solubility of Difficult-to-Test Substances: A Tutorial Review, Anal. Chim. Acta, 2019, 1086, 16–28, DOI:10.1016/j.aca.2019.07.034.
- R. Hammershøj, H. Birch, K. Knudsmark Sjøholm and P. Mayer, Accelerated Passive Dosing of Hydrophobic Complex Mixtures – Controlling the Level and Composition in Aquatic Tests, Environ. Sci. Technol., 2020, 54(8), 4974–4983 CrossRef.
- R. Hammershøj, H. Birch, A. D. Redman and P. Mayer, Mixture Effects on Biodegradation Kinetics of Hydrocarbons in Surface Water: Increasing Concentrations Inhibited Degradation Whereas Multiple Substrates Did Not, Environ. Sci. Technol., 2019, 53(6), 3087–3094, DOI:10.1021/acs.est.9b00638.
- V. H. Edwards, The Influence of High Substrate Concentrations on Microbial Kinetics, Biotechnol. Bioeng., 1970, 679–712, DOI:10.1002/bit.260120504.
- R. Boethling and M. Alexander, Effect of Concentration of Organic Chemicals on Their Biodegradation by Natural Microbial Communities, Appl. Environ. Microbiol., 1979, 37(6), 1211–1216 CrossRef CAS.
- L. Toräng, N. Nyholm and H. J. Albrechtsen, Shifts in Biodegradation Kinetics of the Herbicides MCPP and 2,4-D at Low Concentrations in Aerobic Aquifer Materials, Environ. Sci. Technol., 2003, 37(14), 3095–3103, DOI:10.1021/es026307a.
- K. E. C. Smith, A. Rein, S. Trapp, P. Mayer and U. G. Karlson, Dynamic Passive Dosing for Studying the Biotransformation of Hydrophobic Organic Chemicals: Microbial Degradation as an Example, Environ. Sci. Technol., 2012, 46(9), 4852–4860, DOI:10.1021/es204050u.
- M. Gharasoo, F. Centler, P. Van Cappellen, L. Y. Wick and M. Thullner, Kinetics of Substrate Biodegradation under the Cumulative Effects of Bioavailability and Self-Inhibition, Environ. Sci. Technol., 2015, 49(9), 5529–5537, DOI:10.1021/es505837v.
- T. Egli, How to Live at Very Low Substrate Concentration, Water Res., 2010, 44(17), 4826–4837, DOI:10.1016/j.watres.2010.07.023.
- S. N. Schmidt and P. Mayer, Linking Algal Growth Inhibition to Chemical Activity: Baseline Toxicity Required 1% of Saturation, Chemosphere, 2015, 120, 305–308, DOI:10.1016/j.chemosphere.2014.07.006.
- R. C. Prince, K. M. McFarlin, J. D. Butler, E. J. Febbo, F. C. Y. Wang and T. J. Nedwed, The Primary Biodegradation of Dispersed Crude Oil in the Sea, Chemosphere, 2013, 90, 521–526, DOI:10.1016/j.chemosphere.2012.08.020.
- R. C. Prince, J. D. Butler and A. D. Redman, The Rate of Crude Oil Biodegradation in the Sea, Environ. Sci. Technol., 2017, 51(3), 1278–1284, DOI:10.1021/acs.est.6b03207.
- R. C. Prince, T. F. Parkerton and C. Lee, The Primary Aerobic Biodegradation of Gasoline Hydrocarbons, Environ. Sci. Technol., 2007, 41(9), 3316–3321, DOI:10.1021/es062884d.
- F. Solano-Serena, R. Marchal, M. Ropars, J. M. Lebeault and J. P. Vandecasteele, Biodegradation of Gasoline: Kinetics, Mass Balance and Fate of Individual Hydrocarbons, J. Appl. Microbiol., 1999, 86(6), 1008–1016, DOI:10.1046/j.1365-2672.1999.00782.x.
- F. Solano-Serena, R. Marchal, T. Huet, J. M. Lebeault and J. P. Vandecasteele, Biodegradability of Volatile Hydrocarbons of Gasoline, Appl. Microbiol. Biotechnol., 2000, 54(1), 121–125, DOI:10.1007/s002530000354.
- R. Marchal, S. Penet, F. Solano-Serena and J. P. Vandecasteele, Gasoline and Diesel Oil Biodegradation, Oil Gas Sci. Technol., 2003, 58(4), 441–448, DOI:10.2516/ogst:2003027.
- R. C. Prince, C. Haitmanek and C. C. Lee, The Primary Aerobic Biodegradation of Biodiesel B20, Chemosphere, 2008, 71, 1446–1451, DOI:10.1016/j.chemosphere.2007.12.010.
- H. Birch, R. Hammershøj and P. Mayer, Determining Biodegradation Kinetics of Hydrocarbons at Low Concentrations: Covering 5 and 9 Orders of Magnitude of Kow and Kaw, Environ. Sci. Technol., 2018, 52(4), 2143–2151, DOI:10.1021/acs.est.7b05624.
- S. Achermann, P. Falås, A. Joss, C. B. Mansfeldt, Y. Men, B. Vogler and K. Fenner, Trends in Micropollutant Biotransformation along a Solids Retention Time Gradient, Environ. Sci. Technol., 2018, 52(20), 11601–11611, DOI:10.1021/acs.est.8b02763.
- K. Fenner, C. Screpanti, P. Renold, M. Rouchdi, B. Vogler and S. Rich, Comparison of Small Molecule Biotransformation Half-Lives between Activated Sludge and Soil: Opportunities for Read-Across?, Environ. Sci. Technol., 2020, 54, 3148–3158, DOI:10.1021/acs.est.9b05104.
- H. Birch, H. R. Andersen, M. Comber and P. Mayer, Biodegradation Testing of Chemicals with High Henry's Constants – Separating Mass and Effective Concentration Reveals Higher Rate Constants, Chemosphere, 2017, 174, 716–721, DOI:10.1016/j.chemosphere.2017.02.003.
- Z. Li and M. S. Mclachlan, Biodegradation of Chemicals in Unspiked Surface Waters Downstream of Wastewater Treatment Plants, Environ. Sci. Technol., 2019, 53, 1884–1892, DOI:10.1021/acs.est.8b05191.
- M. T. Pérez, P. Hörtnagl and R. Sommaruga, Contrasting Ability to Take up Leucine and Thymidine among Freshwater Bacterial Groups: Implications for Bacterial Production Measurements, Environ. Microbiol., 2010, 12, 74–82, DOI:10.1111/j.1462-2920.2009.02043.x.
- J. J. Modrzyński, J. H. Christensen, P. Mayer and K. K. Brandt, Limited Recovery of Soil Microbial Activity after Transient Exposure to Gasoline Vapors, Environ. Pollut., 2016, 216, 826–835, DOI:10.1016/j.envpol.2016.06.054.
- S. Brosche and T. Backhaus, Toxicity of Five Protein Synthesis Inhibiting Antibiotics and Their Mixture to Limnic Bacterial Communities, Aquat. Toxicol., 2010, 99, 457–465, DOI:10.1016/j.aquatox.2010.06.008.
- K. K. Brandt, A. Amézquita, T. Backhaus, A. Boxall, A. Coors, T. Heberer, J. R. Lawrence, J. Lazorchak, J. Schönfeld and J. R. Snape,
et al., Ecotoxicological Assessment of Antibiotics: A Call for Improved Consideration of Microorganisms, Environ. Int., 2015, 85, 189–205, DOI:10.1016/j.envint.2015.09.013.
- K. K. Brandt, N. O. G. Jørgensen, T. H. Nielsen and A. Winding, Microbial Community-Level Toxicity Testing of Linear Alkylbenzene Sulfonates in Aquatic Microcosms, FEMS Microbiol. Ecol., 2004, 49, 229–241, DOI:10.1016/j.femsec.2004.03.006.
-
The Danish Environmental Protection Agency, MiljøGIS for Vandområdeplanerne 2015-2021 at, http://miljoegis.mim.dk/cbkort?%26profile=vandrammedirektiv2-bek-2019, accessed Sep 16 2019 Search PubMed.
-
Helmholz Centre for Environmental Research - UFZ. UFZ-LSER Database, https://www.ufz.de/index.php?en=31698%26contentonly=1%26m=0%26lserd_data[mvc]=Public/start, accessed Aug 5 2019 Search PubMed.
- A. K. T. Kirschner and B. Vellmirov, Modification of the 3H-Leucine Centrifugation Method for Determining Bacterial Protein Synthesis in Freshwater Samples, Aquat. Microb. Ecol., 1999, 17, 201–206, DOI:10.3354/ame017201.
- E. Bååth, M. Pettersson and K. H. Söderberg, Adaptation of a Rapid and Economical Microcentrifugation Method to Measure Thymidine and Leucine Incorporation by Soil Bacteria, Soil Biol. Biochem., 2001, 33(11), 1571–1574 CrossRef.
-
ECHA, Registration dossier for linalyl acetate, https://echa.europa.eu/registration-dossier/-/registered-dossier/14484, accessed Oct 23 2019 Search PubMed.
-
ECHA, Registration dossier for linalool, https://echa.europa.eu/registration-dossier/-/registered-dossier/14501, accessed Oct 23 2019 Search PubMed.
-
ECHA, Registration dossier for cineole, https://echa.europa.eu/registration-dossier/-/registered-dossier/13231/, accessed Oct 23 2019 Search PubMed.
-
R. P. Schwarzenbach, P. M. Gschwend and D. M. Imboden, Biological Transformations, in Environmental Organic Chemistry, John Wiley & Sons, Inc., Hoboken, NJ, USA, 2005, pp. 687–773. DOI:10.1002/0471649643.ch17.
- L. N. Trac, K. K. Sjøholm, H. Birch and P. Mayer, Passive Dosing of Petroleum and Essential Oil UVCBs - Linking Mixture Toxicity to Well-Defined Exposure, Environ. Sci. Technol., 2020 Search PubMed.
- H. M. A. Cavanagh and J. M. Wilkinson, Biological Activities of Lavender Essential Oil, Phyther. Res., 2002, 16, 301–308, DOI:10.1002/ptr.1103.
- A. Winding, J. J. Modrzyński, J. H. Christensen, K. K. Brandt and P. Mayer, Soil Bacteria and Protists Show Different Sensitivity to Polycyclic Aromatic Hydrocarbons at Controlled Chemical Activity, FEMS Microbiol. Lett., 2019, 366(17) DOI:10.1093/femsle/fnz214.
- I. M. Head, D. M. Jones and W. F. M. Röling, Marine Microorganisms Make a Meal of Oil, Nat. Rev. Microbiol., 2006, 4(3), 173–182, DOI:10.1038/nrmicro1348.
- F. Brillet, M. Cregut, M. J. Durand, C. Sweetlove, J. C. Chenèble, J. L'Haridon and G. Thouand, Biodegradability Assessment of Complex Chemical Mixtures Using a Carbon Balance Approach, Green Chem., 2018, 20, 1031–1041, 10.1039/c7gc03386a.
-
OECD, Revised Introduction to the OECD Guidelines for Testing of Chemicals, Section 3; OECD Guidelines for the Testing of Chemicals, Section 3, OECD, 2006, DOI:10.1787/9789264030213-en.
- C. Turek and F. C. Stintzing, Stability of Essential Oils: A Review, Compr. Rev. Food Sci. Food Saf., 2013, 12(1), 40–53, DOI:10.1111/1541-4337.12006.
- K. J. Jenner, G. Kreutzer and P. Racine, Persistency Assessment and Aerobic Biodegradation of Selected Cyclic Sesquiterpenes Present in Essential Oils, Environ. Toxicol. Chem., 2011, 30(5), 1096–1108, DOI:10.1002/etc.492.
Footnote |
† Electronic supplementary information (ESI) available: The following files are available free of charge: list of mixture constituents and tentative identifications, passive dosing loading kinetics, characterization of the surface water inoculum, mass balance calculations, degradation curves and kinetics for diesel oil constituents, degradation curves and kinetics for lavender oil constituents, and Fig. 2 and 3 with R2 values (PDF). See DOI: 10.1039/d0em00288g |
|
This journal is © The Royal Society of Chemistry 2020 |