Assessing the prevalence, products, and pathways of dissolved organic matter partial photo-oxidation in arctic surface waters†
Received
1st November 2019
, Accepted 10th February 2020
First published on 11th February 2020
Abstract
In sunlit waters, photodegradation of dissolved organic matter (DOM) yields completely oxidized carbon (i.e., CO2) as well as a suite of partially oxidized compounds formed from oxygen incorporation (i.e., partial photo-oxidation). Of these two groups of DOM photo-products, more studies focus on CO2 (a greenhouse gas) than on partially oxidized DOM, which is likely a diverse group of compounds with poorly constrained roles in aquatic carbon cycling or biogeochemistry. The objective of this study is to address knowledge gaps on the prevalence, products, and pathways of DOM partial photo-oxidation. Here we traced the photochemical incorporation of isotopically labelled 18O2 into DOM isolated from Alaskan Arctic surface waters using high-resolution mass spectrometry. Complete and partial photo-oxidation of DOM was also quantified as CO2 production and O2 consumption. The majority of 18O-containing partial oxidation photo-products were classified as carboxylic rich alicyclic molecules (CRAM) and overlapped in composition with previously reported photo-products known to result from the oxidation of DOM by singlet oxygen. These results support a previously proposed hypothesis that photo-oxidation by singlet oxygen may contribute to the formation of CRAM, a compound class of DOM ubiquitously observed in surface waters. The novel application of an isotopic tracer for oxygen incorporation with a mass balance approach to quantify complete and partial photo-oxidation of DOM revealed that less than one mol of O2 is required to produce one mol of CO2. A sensitivity analysis based on this new knowledge demonstrated that the magnitude of DOM partial photo-oxidation may be underestimated by up to four-fold. Consequently, partial photo-oxidation likely plays a more prominent role in shaping DOM composition in sunlit waters of the Arctic than previously understood. Therefore, partial photo-oxidation should be increasingly incorporated into the experimental framework of studies focused on DOM composition in surface waters.
Environmental significance
Sunlight exposure shapes the chemical composition of DOM in surface waters. By tracing the photochemical incorporation of isotopically labelled dissolved oxygen into arctic freshwater DOM using mass spectrometry, we add new knowledge of the prevalence, products, and pathways of DOM photo-oxidation. Partial photo-oxidation of DOM yields a class of oxidized molecules (CRAM) found in all sunlit waters. Rates of DOM partial photo-oxidation reported in the literature are substantially underestimated, indicating that sunlight plays a more prominent role in shaping the chemical composition of DOM in arctic surface waters than previously understood.
|
1. Introduction
Recent work demonstrated that nearly 75% of dissolved organic matter (DOM) degradation in sunlit waters of the Arctic is due to partial photo-oxidation,1 a process that alters the composition of DOM due to the incorporation of oxygen.1–6 Partial photo-oxidation of DOM is quantified as the difference between photochemical O2 consumption and CO2 production by DOM, on a per mol C basis.1,2,5,6 However, this approach to quantify partial photo-oxidation of DOM on a per mol C basis requires one key assumption about the O2 requirements for CO2 production (i.e., 1 mol O2 per mol CO2). Although this assumption is hypothesized to be conservative,1 it has never been explicitly tested, raising the possibility that rates of DOM partial photo-oxidation may be systematically over or underestimated.
For example, in Imnavait Creek, a small headwater stream in the Arctic, the ratio of photochemical O2 consumption to CO2 production is consistently less than or equal to 1, suggesting no partial photo-oxidation of DOM.1,6,7 However, other evidence suggests substantial changes in chemical composition of DOM in Imnavait Creek remaining after photodegradation.7,8 For example, when DOM from the Imnavait Creek basin was exposed to sunlight, microbes shifted their metabolic rates and pathways in response to changes in the oxygen content of DOM (compared to dark controls).9 This response suggests that partial photo-oxidation of DOM is a poorly understood control on DOM lability to microbes in arctic freshwaters like Imnavait Creek.
In addition to the challenge of quantifying partial photo-oxidation of DOM, too little is known about the products to understand the impact of partial photo-oxidation on biogeochemical processes such as microbial degradation of DOM. Carboxylic-rich alicyclic molecules (CRAM) are a class of partially oxidized DOM observed ubiquitously in aquatic ecosystems and thought to be produced by photo-oxidation, among other pathways. For example, the abundance of CRAM has been reported to increase upon exposure of DOM to sunlight.4,6,10,11 Evidence suggests that CRAM is relatively recalcitrant to microbial degradation.12 Thus, photochemical production of CRAM could contribute to some responses of microbes to photo-oxidized DOM.4
Finally, too little is known about the pathways by which DOM is partially photo-oxidized to evaluate its influence on DOM composition in surface waters. Reactive oxygen species (ROS) produced photochemically by DOM, such as singlet oxygen, hydroxyl radical, or superoxide, likely oxidize DOM,3,4,13–18 although the relative importance of each ROS-mediated oxidation pathway is poorly understood. For example, singlet oxidation of DOM has been hypothesized to account for a substantial fraction of DOM photo-oxidation.3,4 Exposure of DOM to sunlight in the presence of chemical sensitizers for singlet oxygen (1O2) yielded partially oxidized products that were operationally defined as CRAM.4,18 This finding raised the possibility that singlet oxygen is a critical intermediate in the formation of CRAM. However, there is no evidence in support of this hypothesis in the absence of chemical sensitizers for singlet oxygen.
Here we address knowledge gaps on the prevalence, products and pathways of DOM partial photo-oxidation. Specifically, we exposed DOM isolated from arctic surface waters to 18O-labelled O2 and sunlight, characterized the 18O-containing photo-products using high-resolution mass spectrometry, and quantified complete and partial photo-oxidation of DOM. The majority of DOM photo-products classified as CRAM and were consistent with partial oxidation of DOM by singlet oxygen. Our experimental results demonstrated that less than one mol of O2 is required to photochemically produce one mol CO2. A sensitivity analysis based on this new knowledge suggests that rates of partial photo-oxidation of DOM have been substantially underestimated in arctic freshwaters.
2. Materials and methods
2.1 DOM sample preparation
Two freeze-dried XAD-8 extracted fulvic acid isolates were used in this study, Imnavait Creek and Toolik Lake DOM. Both water bodies are located on the North Slope of Alaska and are routinely monitored by the Toolik Lake Long-Term Ecological Research program (https://arc-lter.ecosystems.mbl.edu/). Imnavait Creek (68.61°N, 149.32°E) is a first-order headwater stream and was sampled on 23-June-2002. Toolik Lake (68.63°N, 149.59°E) is a 150 Ha, 25 m deep, oligotrophic kettle lake and was sampled on 19-June-2002. Isolation of the fulvic acid fraction of both DOM samples was previously described.19 Elemental analysis and 13C NMR of the isolates were previously reported.19 Preparation of DOM used in the experimental work proceeded as follows: reconstitution of DOM in Milli-Q water at a target DOC concentration of 10 mg C L−1,7,8 pH adjustment to 7.0 ± 0.1 using dilute sodium hydroxide, equilibration with the atmosphere overnight on a stir-plate at room temperature, and filtration (pre-combusted GF/F, nominal 0.7 μm, Whatman).
2.2 DOC concentration and optical characterization of DOM
Dissolved organic carbon concentration (DOC) was quantified as CO2 after high-temperature combustion using a Shimadzu 5000A TOC analyzer.20 Absorption spectra were collected using a 1 cm pathlength UV-visible spectrophotometer (Aqualog; Horiba Scientific). Naperian absorption coefficients were calculated by multiplying absorbance (A) by 2.303 and dividing by the pathlength (m) of the quartz cuvette. Specific UV-visible absorbance as 254 nm (SUVA254; L mg C−1 m−1) were calculated as previously described.21 The spectral slope ratio was calculated as the ratio of the slope from 275 to 295 nm to the slope from 350 to 400 nm.22 Protocols for collecting DOM fluorescence excitation-emission matrices are previously described.23–25 All bulk measurements (DOC and optical spectroscopy) were conducted prior to PPL solid-phase extraction for FT-ICR MS analysis.
2.3 Photochemical O2 consumption and CO2 production
Air-equilibrated DOM was transferred to pre-combusted, air-tight 12 mL borosilicate vials with no headspace (Labco, Inc.; catalog # 9RK8W). Light transmission of the vials is ≥70% at 320 nm.26 The vials were placed horizontally in an Atlas XLS + solar simulator along the horizontal profile of the long-arc lamp, which minimized irradiance variability in the chamber. During the photo-exposure the temperature of the vials was maintained at 20 °C, similar to the room temperature outside of the chamber where the foil-wrapped dark controls were kept. The exposure time was six hours, equivalent to ∼15 hours of natural clear-sky, mid-day June sunlight at Toolik Field Station, AK.9 Consistent with previous studies,4,6 addition of catalase following light exposure (200 units per mL) did not result in detectable differences in dissolved O2 (i.e., <1 μM O2 production from H2O2 decomposition). These results indicate that H2O2 production had no detectable influence on photochemical O2 consumption to CO2 production ratios reported in this study. The short exposure time was selected to ensure the detection of a change in DOM chemical composition, photochemical O2 consumption, and photochemical CO2 production, while minimizing the amount of time that DOM composition is altered in the absence of microbes. Photodegradation of DOM in sunlit waters occurs in the presence of microbes, and the interactions between sunlight and microbes in arctic surface waters are well documented.27 Moreover, there is currently no evidence for large shifts in photochemical O2 consumption to CO2 production ratios over long periods of sunlight exposure, upwards of 21 days.28 Consistently, kinetic isotope fractionation of O2 during photochemical oxidation of DOM is constant with increasing irradiation time (upwards of two weeks of natural sunlight exposure), indicating that some processes of DOM photodegradation remain the same with increasing light exposure.29 Therefore, the results from our short experimental exposure times should apply to the relatively short residence times of DOM in sunlit surface waters of the Arctic.7,30
Following irradiation, photochemical CO2 production and O2 consumption was quantified. CO2 production was quantified as the light minus dark difference in dissolved inorganic carbon (AS-C3 DIC analyzer; Apollo SciTech, Inc.). Oxygen consumption was quantified as the dark minus light difference in dissolved oxygen using membrane inlet mass spectrometry (MIMS; Bay Instruments, Inc.).31
2.4 Photochemical incorporation of 18O2 into DOM
Six mL of DOM was placed into four pre-combusted, air-tight 12 mL borosilicate vials and purged with ultra-high purity N2 until O2 (mass 32) was non-detectable using MIMS. Ten cm3 of 97 atom percent 18O2 (Sigma Aldrich) was injected into two of the four vials (referred to as “18O2 treatment”). Monitoring of masses 32 (16O2) and 36 (18O2) on the MIMS revealed that 91% of total O2 in the 18O2 treatment experimental vial (i.e., 16O2 + 18O2) was isotopically labelled. Ten cm3 of lab air was injected into the two remaining vials (referred to as “16O2 treatment”). One vial of each treatment was kept in the dark, while the other vial of each treatment was exposed to six hours of simulated sunlight, as described above. The 18O2 treatments were made by addition of pure O2 while the 16O2 treatments were made by addition of lab air (∼20% O2). It is unlikely that differences in initial O2 concentrations between these treatments impacted the products of photo-oxidation because O2 was not limiting, as indicated by ∼20 μM O2 consumption for samples equilibrated with lab air (<10% depletion; Fig. 1). Following the exposure, the DOM was acidified with trace metal grade HCl, placed on ice and shipped overnight to the Environmental Molecular Sciences Laboratory (EMSL) at the Pacific Northwest National Laboratory for high resolution mass spectrometry analysis.
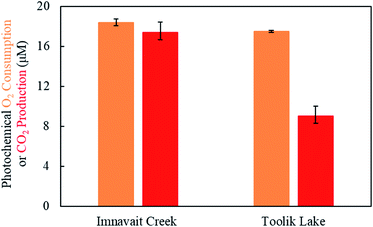 |
| Fig. 1 Photochemical oxygen consumption and carbon dioxide production by Imnavait Creek (left) and Toolik Lake (right) DOM. Error bars represent one standard deviation from the mean (N = 3). | |
Prior to analysis, the DOM was loaded onto PPL solid-phase cartridges (Agilent) to minimize ionization suppression by residual salts that were incompletely removed during the XAD-8 isolation. Due to limited volume, the extraction efficiency of DOM by the PPL solid-phase was not quantified. Previous studies of nearly a dozen DOM sources from Alaskan Arctic surface and soil waters reported approximately 60% extraction efficiency by PPL solid-phase.6,9,25 The DOM PPL extracts were analyzed using a 12 T Bruker SolariX FT-ICR mass spectrometer. The DOM extracts were ionized using electrospray ionization conducted in negative mode. Elemental formulas were assigned to internally calibrated masses followed previously described approaches,4–6,9,25 with one important exception. Elemental formulas including 16O were initially assigned (considering C, H, O, N, and S), and then the unassigned masses were subjected to additional formula assignment including 18O. Only formulas that agreed within an error of <0.5 ppm to the calculated exact mass of the formula were accepted. Formulas were classified as aromatic using the modified aromaticity index (AIMOD).32 Formulas were classified as CRAM if: DBE/C = 0.30–0.68, DBE/H = 0.20–0.95, and DBE/O = 0.77–1.75.3318O-containing photo-products were only detected in the light-exposed 18O2 treatment, indicating that isotopically labelled 18O2 and irradiation were required to yield 18O-containing photo-products. Due to the cost of 18O2 and limited FT-ICR MS instrument time, replicates of 18O2 treatments and controls were not conducted. Previous work on FT-ICR MS of SPE-DOM demonstrated high reproducibility in peak intensity and formula assignments from terrestrially-derived DOM leached from soils within the Imnavait Creek watershed.25
3. Results
3.1 Chemical composition of Imnavait Creek and Toolik Lake DOM
Although both Imnavait Creek and Toolik Lake DOM are terrestrially-derived, there were notable differences in their chemical compositions. Optical spectroscopy and high-resolution mass spectrometry analyses indicated that Imnavait Creek DOM was more aromatic and had a higher average molecular weight than Toolik Lake DOM. Specific ultraviolet absorbance at 254 nm (SUVA254), average double bond equivalents normalized to carbon number, and AIMOD was higher for Imnavait Creek compared to Toolik Lake DOM indicating that Imnavait Creek DOM was more aromatic than Toolik Lake DOM (Tables 1 and 2). Slope ratio was lower for Imnavait Creek compared to Toolik Lake DOM (Table 1) consistent with a higher average molecular weight for Imnavait Creek compared to Toolik Lake DOM as determined by high-resolution mass spectrometry (Table 2).
Table 1 Dissolved gas concentrations and bulk properties of dark-control and light-exposed Imnavait Creek and Toolik Lake DOM. O2 = dissolved oxygen, DIC = dissolved inorganic carbon, O2
:
CO2 = photochemical O2 consumption to CO2 production ratio, DOC = dissolved organic carbon, SUVA254 = specific UV absorbance at 254 nm, INT CDOM = naperian absorbance integrated from 300–400 nm, SR = slope ratio, INT fluor = integrated fluorescence from 240–600 nm (after removing first and second order Rayleigh and Raman scattering peaks), and FI = fluorescence index. ± 1SD from the mean of triplicates
Treatment |
Units |
Imnavait Creek |
Toolik Lake |
Dark |
Light |
Dark |
Light |
O2 |
(μM) |
274 ± <1 |
255 ± <1 |
272 ± <1 |
255 ± <1 |
DIC |
(μM) |
60 ± 1 |
77 ± 1 |
55 ± <1 |
64 ± 1 |
O2 : CO2 |
— |
1.1 ± 0.1 |
2.0 ± 0.1 |
DOC |
(μM) |
839 |
— |
1046 |
— |
SUVA254 |
(L mg C−1 m−1) |
4.1 ± <0.1 |
— |
3.7 ± <0.1 |
— |
INT CDOM |
(m−1) |
3157 ± 33 |
2702 ± 244 |
2435 ± 35 |
2167 ± 4 |
S
R
|
— |
0.68 ± 0.01 |
0.75 ± <0.01 |
0.79 ± 0.01 |
0.86 ± <0.01 |
INT fluor |
(RU) |
19 942 ± 74 |
13 131 ± 74 |
20 931 ± 503 |
15 657 ± 706 |
FI |
— |
1.40 ± 0.01 |
1.26 ± 0.01 |
1.37 ± <0.01 |
1.32 ± <0.01 |
Table 2 Average chemical characteristics of (i) all formulas detected within Imnavait Creek DOM, (ii) formulas within Imnavait Creek DOM that were produced by sunlight and contained 18O, (iii) all formulas detected within Toolik Lake DOM, (iv) formulas within Toolik Lake DOM that were produced by sunlight and contained 18O, (v) the common subset of formulas detected in both (ii) and (iv), and (vi) singlet oxidation photo-products detected within Suwanee River and Pony Lake fulvic acid.4 Mass = daltons, C # = the number of carbon atoms, O/C = atomic oxygen to carbon ratio, H/C = atomic hydrogen to carbon ratio, DBE = double bond equivalents, DBE/C = double bond equivalents normalized to carbon number, and AIMOD = modified aromaticity index. ± 1SD from the mean of all formulas
DOM sources |
N
|
Mass |
C # |
O/C |
H/C |
DBE |
DBE/C |
AIMOD |
(i) Imnavait |
3187 |
494 ± 126 |
24 ± 7 |
0.45 ± 0.20 |
1.00 ± 0.31 |
14 ± 6 |
0.55 ± 0.16 |
0.41 ± 0.23 |
(ii) Imnavait 18O |
379 |
348 ± 79 |
16 ± 5 |
0.53 ± 0.15 |
1.03 ± 0.27 |
9 ± 3 |
0.40 ± 0.18 |
0.40 ± 0.18 |
(iii) Toolik Lake |
3032 |
440 ± 108 |
24 ± 7 |
0.33 ± 0.19 |
1.09 ± 0.38 |
12 ± 6 |
0.51 ± 0.19 |
0.39 ± 0.28 |
(iv) Toolik Lake 18O |
287 |
382 ± 111 |
20 ± 7 |
0.39 ± 0.12 |
1.19 ± 0.23 |
9 ± 4 |
0.47 ± 0.12 |
0.34 ± 0.14 |
(v) IMN + TL 18O common |
159 |
353 ± 62 |
17 ± 4 |
0.44 ± 0.12 |
1.15 ± 0.19 |
8 ± 2 |
0.49 ± 0.10 |
0.34 ± 0.13 |
(vi) Singlet oxidation products |
167 |
442 ± 30 |
23 ± 2 |
0.41 ± 0.10 |
1.22 ± 0.16 |
10 ± 2 |
0.44 ± 0.08 |
0.29 ± 0.09 |
For both DOM sources, sunlight degraded chromophores and fluorophores within the DOM pool. Exposing Imnavait Creek DOM to sunlight decreased integrated absorbance by 14 ± 8%, integrated fluorescence by 34 ± 1%, and fluorescence index by 10 ± 1%, and increased slope ratio by 10 ± 1% (Table 1). Exposing Toolik Lake DOM to sunlight decreased integrated absorbance by 11 ± 1%, integrated fluorescence by 25 ± 4%, and fluorescence index by 4 ± 1%, and increased slope ratio by 10 ± 2% (Table 1).
3.2 Photochemical O2 consumption and CO2 production
Exposure of Imnavait Creek DOM to sunlight resulted in a similar amount of O2 consumption as CO2 production (Fig. 1, p = 0.14, two-tailed paired Students t-test; O2 consumption = 18 ± <1 μM, CO2 production = 17 ± 1 μM, N = 3, ±SD). In contrast, Toolik Lake DOM consumed two-fold greater O2 than CO2 it produced (Fig. 1, p = 0.002, two-tailed paired Students t-test; O2 consumption = 18 ± <1 μM, CO2 production = 9 ± 1 μM, N = 3, ±SD). Accordingly, the ratio of photochemical O2 consumption to CO2 production was significantly lower for Imnavait Creek DOM compared to Toolik Lake DOM (p-value = 0.001, two-tailed unpaired Students t-test; Imnavait Creek = 1.1 ± 0.1, Toolik Lake = 2.0 ± 0.1, N = 3, ±SD).
3.3 Detection of 18O photo-products using high resolution mass spectrometry
No masses corresponding to 18O-containing photo-products were detected in 16O dark-control and light-exposed treatments. In contrast, in the 18O treatments hundreds of 18O-containing formulas were produced by sunlight and detected within the Imnavait Creek and Toolik Lake DOM mass spectra (Fig. 2; Table 2). On a number basis, 18O photo-products accounted for ∼10% of total formulas detected in each DOM after exposure to sunlight. The majority of 18O photo-products contained one 18O atom rather than two atoms (Imnavait Creek = 69%, Toolik Lake = 80%). The majority (>75%) of 18O-formulas contained only CHO for both Imnavait Creek and Toolik Lake DOM. 18O was incorporated into Imnavait Creek DOM that was more oxidized (higher O/C), more conjugated (higher DBE/C), and more aromatic (higher AIMOD) than Toolik Lake DOM (Fig. 3, Table 2). DOM partial photo-oxidation products primarily classified as carboxylic-rich alicyclic molecules (CRAM),33,34 comprising 61 and 83% of 18O photo-products detected in Imnavait Creek and Toolik Lake DOM, respectively.
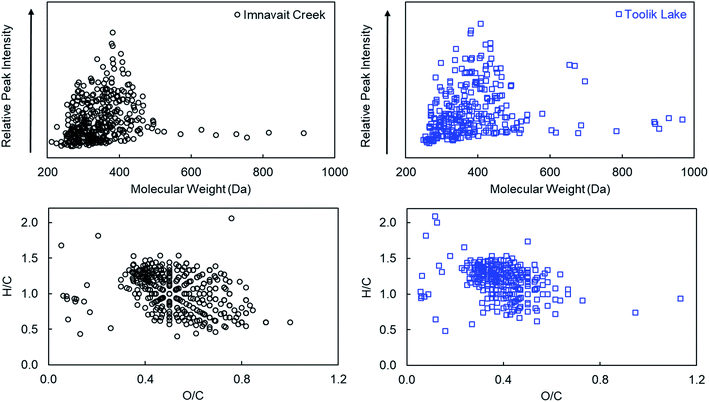 |
| Fig. 2 Molecular-level compositional characteristics of 18O-containing products after exposing Imnavait Creek (black circles) and Toolik Lake DOM (blue squares) to sunlight. Top: molecular weight vs. relative peak intensity of 18O photo-products. Bottom: van Krevelen plots of 18O photo-products. | |
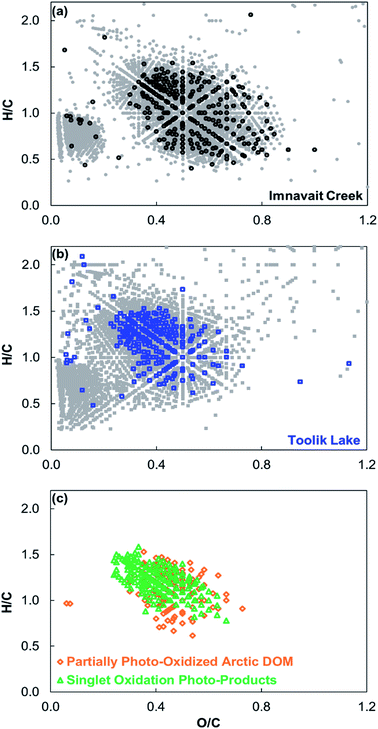 |
| Fig. 3 (a) Comparison of 18O-containing photo-products for Imnavait Creek DOM (foreground black circles) to all formulas detected in Imnavait Creek DOM (background grey circles). (b) Comparison of 18O-containing photo-products for Toolik Lake DOM (foreground blue squares) to all formulas detected in Toolik Lake DOM (background grey squares). (c) Comparison of 18O-containing formulas produced by sunlight in both Imnavait Creek and Toolik Lake DOM (orange diamonds) versus18O-containing formulas produced through the singlet oxidation of Suwannee River and Pony Lake Fulvic Acid (green triangles4). | |
The average compositional characteristics of 18O photo-products detected in both Imnavait Creek and Toolik Lake DOM spanned a narrower range of H/C and O/C ratios in van Krevelen space compared to all formulas detected in each DOM (Fig. 3; Table 2). Imnavait Creek 18O photo-products generally clustered from O/C 0.4–0.8 and H/C 0.5–1.4, while all Imnavait Creek formulas spanned a wider range from O/C 0.1–0.9 and H/C 0.4–1.8. Toolik Lake 18O photo-products generally clustered from O/C 0.3–0.6 and H/C 0.6–1.5, while all Toolik Lake formulas spanned a wider range from O/C 0.1–0.9 and H/C 0.2–2.0.
4. Discussion
4.1 Evidence for partial photo-oxidation of DOM
Three lines of evidence indicate that the 18O-containing formulas categorized as partial oxidation products were produced by sunlight. First, there were no masses categorized as 18O-containing photo-products in the 16O dark-controls and light-exposed treatments, indicating that 18O2 and sunlight exposure was required to yield 18O-containing photo-products. Second, no masses corresponding to 18O-containing photo-products were detected in the 18O2 dark controls. This result is expected because isotopic exchange does not occur between O2 and organically bound oxygen within DOM, and auto-oxidation of DOM is negligible. Third, the 18O-containing photo-products had similar chemical compositions as formulas produced by the partial photo-oxidation of other sources of terrestrial DOM, specifically Suwannee River DOM4 and Great Dismal Swamp DOM.18 Together, these results demonstrate that the 18O-containing formulas detected in this study were products of partial photo-oxidation formed through the incorporation of 18O2 into DOM, consistent with the partial photo-oxidation of DOM by reactive oxygen species.3,4,18
There are several reasons why the number of 18O-containing photo-products identified by high resolution mass spectrometry is conservative. First, 18O-containing photo-products were identified on DOM following SPE-PPL extraction, during which some relatively hydrophilic partially-oxidized DOM may not have been retained.25,35 Second, a substantial fraction of high O/C compounds are likely not detected by FT-ICR MS analysis of DOM.25,35 Third, others have estimated that about 20% of photo-oxidized DOM of terrestrial origin are low molecular weight compounds such as acetate,36 which would not be detected by FT-ICR MS. Thus, we infer that the 18O-containing photo-products identified in this study provide a conservative assessment of the number and type of compounds produced by partial photo-oxidation of DOM.
4.2 Evidence for singlet oxidation of DOM
Singlet oxygen (1O2) is hypothesized to be an important oxidant of DOM in sunlit surface waters. Using DOM derived from Sigma Aldrich humic acid, it was first reported that steady state concentrations of (1O2) are orders of magnitude higher in the hydrophobic microenvironments of DOM compared to the bulk aqueous phase.37 These results were later corroborated using Suwannee River and Pony Lake fulvic acids (SRFA and PLFA, respectively).38 Subsequent studies revealed that under conditions that artificially sensitize the formation of 1O2, a wide range of DOM sources (including SRFA and PLFA) are susceptible to singlet oxidation,4,18 at rates independent of DOM source.4
The results from this study add additional support to the hypothesis that 1O2 is an important oxidant of DOM. Imnavait Creek and Toolik Lake 18O photo-products overlapped in chemical composition with singlet oxidation products of SRFA and PLFA (Fig. 3; Table 2),4 and lignin-derived DOM from the Great Dismal Swamp.18 In van Krevelen space (i.e., O/C and H/C) there is considerable overlap in chemical composition between Imnavait Creek and Toolik Lake 18O photo-products and singlet oxidation products of SRFA and PLFA (Fig. 3). Accordingly, the majority of Imnavait Creek and Toolik Lake 18O photo-products are classified as CRAM, similar to singlet oxidation products of SRFA and PLFA.4 The overlap in products produced by photo-oxidation in this study with those known to result from 1O2 (i) support prior evidence for singlet oxidation of DOM as a potential pathway to form CRAM,4 a class of DOM detected ubiquitously in surface waters,33,34 and (ii) contributes to the mounting body of evidence that 1O2 may be an important oxidant of DOM in sunlit surface waters.
Alternative DOM partial oxidation pathways include reaction with hydroxyl radical (˙OH) and superoxide (O2˙−). Photochemical production of ˙OH in arctic surface waters has previously been reported to account for up to 4% of total photochemical mineralization of DOM to CO2.15 Considering that photochemical O2 consumption to CO2 production ratios are ≥1, and conservatively assuming that 50% of hydroxyl radical production comes from an O2 dependent pathway,39 it is very unlikely that ˙OH contributes substantially to the partial photo-oxidation of DOM in arctic surface waters. The role of O2˙− in the photo-oxidation of arctic DOM has never been tested. However, unlike the substantial overlap between 18O photo-products reported in this study and 1O2 products of lignin-derived DOM from the Great Dismal Swamp,18 the overlap between 18O photo-products and O2˙− oxidation products of lignin-derived DOM is low.18 This qualitative assessment suggests that O2˙− likely does not play a major role in the oxidation of Imnavait Creek or Toolik Lake DOM reported in this study. However, given the well-established limitations of electrospray FT-ICR MS, such as SPE extraction and ionization efficiency, this conclusion warrants further testing with more quantitative approaches and DOM spanning a broader range of compositions than in this study.
4.3 Evaluating the potential for underestimation of DOM partial photo-oxidation
A key assumption to the current approach to quantify partial photo-oxidation of DOM1 is that one mol of O2 is photochemically consumed per mol of CO2 produced. A stoichiometry of 1 mol O2 consumed per mol of CO2 produced for photo-oxidation of DOM has been assumed because the average oxidation state of DOM is likely close to zero40 and the oxidation state of CO2 is +4. In cases where photochemical O2 consumption is greater than CO2 production (e.g., freshwaters),1 the excess O2 consumed is interpreted as molecular incorporation into DOM (i.e., partial photo-oxidation). Therefore, in cases where O2 consumption is equal to or less than CO2 production (e.g., headwaters streams; Fig. 1), partial photo-oxidation of DOM is assumed to be negligible. Consistent with our previous findings,1,6,7 the stoichiometry of photochemical O2 consumption per CO2 production from Imnavait Creek DOM was ∼1 (Fig. 1), suggesting that it had low or no susceptibility to partial photo-oxidation. However, hundreds of 18O photo-products were detected, indicating that Imnavait Creek DOM was susceptible to partial photo-oxidation despite its low photochemical O2 to CO2 ratio (Fig. 2 and 3, Table 2). The only way to explain this discrepancy is that less than one mol of O2 is photochemically consumed per mol of CO2 produced.
Photo-decarboxylation of DOM to CO2 is a plausible explanation for the low O2 requirement for CO2 production from Imnavait Creek DOM. Photo-decarboxylation (eqn (1)) is a reaction where organic acids are oxidized to CO2 in the presence of sunlight with relatively low O2 consumption.
| R-COOH + (XO2) → R-H + CO2 | (1) |
Estimates from model compounds and experimental manipulations suggest that photo-decarboxylation reactions require 0 to 0.5 mol O2 per mol of CO2 produced,28,41–43 much lower than currently assumed for DOM.1 Photo-decarboxylation has been implicated in the photo-oxidation of terrestrial DOM to CO2.6,11,28,42,44 Quantitatively, photo-decarboxylation is estimated account for 40–90% of Imnavait Creek DOM mineralized to CO2 (ref. 6) and up to 100% of temperate river DOM converted to CO2.28
The low O2 requirement of photo-decarboxylation reactions has substantial implications for quantifying the magnitude of DOM partial photo-oxidation. For example, assuming (i) a typical ratio of photochemical O2 consumption to CO2 production of 1.5 (ref. 1) (between the ratios observed for Imnavait Creek and Toolik Lake DOM in Fig. 1 and Table 1), (ii) that photo-decarboxylation accounts for 10 to 100% of CO2 production, and (iii) that photo-decarboxylation requires 0.5 mol O2 per mol of CO2, partial photo-oxidation is underestimated by 1.3 to 4-fold, respectively (relative to a scenario where no CO2 production comes from photo-decarboxylation; Table S1†). This underestimation of partial photo-oxidation decreases as the relative importance of partial to complete photo-oxidation increases. For example, as partial photo-oxidation increases relative to complete photo-oxidation to CO2, as indicated by a high photochemical O2 consumption to CO2 production ratio of ∼5, partial photo-oxidation is underestimated only by 4 to 38% (Table S1†). In other words, when partial photo-oxidation dominates DOM photodegradation, any assumption made about the O2 needed for complete oxidation to CO2 has a relatively small impact on the quantification of partial photo-oxidation.
5. Environmental implications and remaining knowledge gaps
The results of this study challenge a common assumption made to estimate partial photo-oxidation of DOM in surface waters, and, in turn, reveal that the magnitude of partial photo-oxidation of DOM could be underestimated by up to 4-fold (Table S1†). The identification of 18O-containing partial photo-oxidation products (Fig. 2 and 3) at a 1
:
1 ratio of photochemical O2 consumption per CO2 production from DOM (Fig. 1) demonstrates that less than 1 mol O2 may be required to produce 1 mol CO2. Therefore, quantification of partial photo-oxidation as the O2 consumption in excess of photochemical CO2 production (based on the assumed 1
:
1 stoichiometry) may result in underestimation of partial photo-oxidation.
This finding implies that estimates of partial photo-oxidation of DOM have been too conservative, particularly in high iron freshwaters of the Arctic7,15 where there is almost always less photochemical O2 consumption than CO2 production.1,6,7 Iron is hypothesized28 to catalyze the photo-decarboxylation of DOM to CO2 (by ligand-to-metal charge transfer reactions); consistent with less O2 consumption than CO2 production in high iron waters of the Arctic. There was likely relatively less influence of iron on the ratio of O2 consumption to CO2 production for the fulvic acid fraction of DOM in this study compared to the DOM in the source waters due to loss of iron during the extraction process. Thus, in high iron waters of the Arctic,1,6,7 when photochemical O2 consumption is less than or equivalent to CO2 production our results imply that partial photo-oxidation is altering the composition of the DOM.
The results from this study suggest that partial photo-oxidation of DOM is a widespread and underestimated process influencing DOM composition in arctic surface waters. For example, even when using the assumptions shown here to be markedly conservative, partial photo-oxidation dominated the water-column processing of DOM in arctic freshwaters, occurring at rates up to 4-fold faster than photomineralization of DOM and 2 to 15-fold faster than rates of bacterial respiration.1 Consequently, partial photo-oxidation of DOM, possibly involving singlet oxygen (Fig. 3), is a more important process controlling DOM composition in sunlit surface waters than previously understood. Therefore, studies of DOM photodegradation that quantify only complete oxidation of DOM to CO2 (i.e., photomineralization) are potentially missing partial photo-oxidation, a major process that alters the chemical composition of DOM remaining after sunlight exposure.
Results of this study suggest additional work is needed to address another key uncertainty used to quantify partial photo-oxidation of DOM. For example, partial photo-oxidation of DOM is quantified assuming that the average O/C of partial photo-oxidation products is 0.5, which in turn is based on the average O/C of bulk DOM (i.e., ∼0.5). Results from high resolution mass spectrometry qualitatively suggest a range of O/C of partially photo-oxidized DOM. For example, the average O/C of SRFA and PLFA singlet oxidation photo-products was 0.42 and 0.32, respectively.4 In this study, the average O/C of partial photo-oxidation products for Imnavait Creek and Toolik Lake DOM was 0.53 and 0.39, respectively (Fig. 2, Table 2). These findings suggest that the average O/C of partial photo-oxidation production could deviate substantially from the assumed 0.5 value, and this deviation could have major implications for quantifying the magnitude of DOM partial photo-oxidation. However, given the widely documented biases of FT-ICR MS, such as SPE extraction and ionization efficiency, future research should prioritize quantification of the O/C stoichiometry of partial photo-oxidation products.
Partial photo-oxidation of DOM has been hypothesized as a pathway for the formation of CRAM given that formulas characterized as CRAM increase in abundance following photo-exposure.4,6,10,11 In this study, the majority of 18O-containing partial oxidation photo-products were classified as CRAM (Fig. 2) and overlapped in composition with previously reported photo-products known to result from 1O2 (Fig. 3).4 Therefore, our results provide indirect support for the hypothesized pathway of CRAM formation: partial photo-oxidation of DOM by singlet oxygen.
Understanding how partial photo-oxidation alters the chemical composition of DOM is important because DOM composition is a major control on microbial community structure and function.8,9,45–51 In arctic lakes46 and rivers,47 DOM composition is one of the strongest predictors of microbial community composition. Likewise, minor photochemical alterations to DOM draining Arctic permafrost soils (<10% of the total C) substantially shifts microbial metabolic rates and pathways.9 The influence of photochemical alterations of DOM on microbial processing of DOM likely applies to sunlit freshwaters outside the Arctic.52 For example, less than one hour of sunlight exposure to temperate stream water had substantial impacts on microbial respiration of DOM due to changes in the chemical composition of DOM (i.e., partial photo-oxidation).51 Therefore, partial photo-oxidation of DOM may be a widespread process that impacts the structure and function of microbial communities in inland surface waters, a hypothesis that should be explicitly tested in future work. Partial photo-oxidation of DOM should be increasingly incorporated into experimental frameworks in order to accurately estimate its role in shaping DOM composition and driving biogeochemical processes in sunlit waters.
Conflicts of interest
The authors declare no conflicts of interest
Acknowledgements
We thank K. Harrold, L. Treibergs, K. Yuhas, and G. Kling for assistance with the experimental design and work. Thanks to M. Tfaily for assisting with the mass spectrometry analysis, which was performed using EMSL, a DOE Office of Science User Facility sponsored by the Office of BER and located at PNNL. Funding for this work was provided by NSF CAREER 1351745, DEB 1147378, 1347042, 0639790, 1147336, 1026843, PLR 1504006, the Frank and Lisina Hoch Endowed Fund (CPW), the Andrew W. Mellon Foundation Endowed Fund for Innovative Research (CPW), and the Camille and Henry Dreyfus Foundation Postdoctoral Program in Environmental Chemistry (CPW and RMC).
References
- R. M. Cory, C. P. Ward, B. C. Crump and G. W. Kling, Sunlight controls water column processing of carbon in arctic fresh waters, Science, 2014, 345, 925–928 CrossRef CAS PubMed.
- S. Andrews, S. Caron and O. Zafiriou, Photochemical oxygen consumption in marine waters: a major sink for colored dissolved organic matter?, Limnol. Oceanogr., 2000, 45, 267–277 CrossRef CAS.
- R. M. Cory, J. B. Cotner and K. McNeill, Quantifying interactions between singlet oxygen and aquatic fulvic acids, Environ. Sci. Technol., 2009, 43, 718–723 CrossRef CAS PubMed.
- R. M. Cory, K. McNeill, J. P. Cotner, A. Amado, J. M. Purcell and A. G. Marshall, Singlet oxygen in the coupled photochemical and biochemical oxidation of dissolved organic matter, Environ. Sci. Technol., 2010, 44, 3683–3689 CrossRef CAS PubMed.
- C. P. Ward, R. L. Sleighter, P. G. Hatcher and R. M. Cory, Insights into the complete and partial photooxidation of black carbon in surface waters, Environ. Sci.: Processes Impacts, 2014, 16, 721–731 RSC.
- C. P. Ward and R. M. Cory, Complete and partial photo-oxidation of dissolved organic matter draining permafrost soils, Environ. Sci. Technol., 2016, 50, 3545–3553 CrossRef CAS PubMed.
- R. M. Cory, K. H. Harrold, B. T. Neilson and G. W. Kling, Controls on DOM degradation in a headwater stream: the influence of DOM amount and lability, light attenuation and exposure, Biogeosciences, 2015, 12, 6669–6685 CrossRef CAS.
- R. M. Cory, B. C. Crump, J. A. Dobkowski and G. W. Kling, Surface exposure to sunlight stimulates CO2 release from permafrost soil carbon in the Arctic, Proc. Natl. Acad. Sci. U. S. A., 2013, 110, 3429–3434 CrossRef CAS PubMed.
- C. P. Ward, S. G. Nalven, B. C. Crump, G. W. Kling and R. M. Cory, Photochemical alteration of organic carbon draining permafrost soils shifts microbial metabolic pathways and stimulates respiration, Nat. Commun., 2017, 8, 1–8 CrossRef PubMed.
- M. Gonsior, N. Hertkorn, M. H. Conte, W. J. Cooper, D. Bastviken, E. Druffel and P. Schmitt-Kopplin, Photochemical production of polyols arising from significant photo-transformation of dissolved organic matter in the oligotrophic surface ocean, Mar. Chem., 2014, 163, 10–18 CrossRef CAS.
- M. Gonsior, P. Schmitt-Kopplin and D. Bastviken, Depth-dependent molecular composition and photo-reactivity of dissolved organic matter in a boreal lake under winter and summer conditions, Biogeosciences, 2013, 10, 6945–6956 CrossRef CAS.
- R. L. Sleighter, R. M. Cory, L. A. Kaplan, H. A. N. Abdulla and P. G. Hatcher, A coupled geochemical and biogeochemical approach to characterize the bioreactivity of dissolved organic matter from a headwater stream, J. Geophys. Res.: Biogeosci., 2014, 119, 1520–1537 CrossRef CAS.
- B. A. Southworth and B. M. Voelker, Hydroxyl radical production via the photo-fenton reaction in the presence of fulvic acid, Environ. Sci. Technol., 2003, 37, 1130–1136 CrossRef CAS PubMed.
- E. M. White, P. P. Vaughan and R. G. Zepp, Role of the photo-Fenton reaction in the production of hydroxyl radicals and photobleaching of colored dissolved organic matter in a coastal river of the southeastern United States, Aquat. Sci., 2003, 65, 402–414 CrossRef CAS.
- S. E. Page, J. R. Logan, R. M. Cory and K. McNeill, Evidence for dissolved organic matter as the primary source and sink of photochemically produced hydroxyl radical in arctic surface waters, Environ. Sci.: Processes Impacts, 2014, 16, 807–822 RSC.
- J. V. Goldstone and B. M. Voelker, Chemistry of superoxide radical in seawater: CDOM associated sink of superoxide in coastal waters, Environ. Sci. Technol., 2000, 34, 1043–1048 CrossRef CAS.
- J. Ma, H. Zhou, S. Yan and W. Song, Kinetics studies and mechanistic considerations on the reactions of superoxide radical ions with dissolved organic matter, Water Res., 2019, 149, 56–64 CrossRef CAS PubMed.
- D. C. Waggoner, A. S. Wozniak, R. M. Cory and P. G. Hatcher, The role of reactive oxygen species in the degradation of lignin derived dissolved organic matter, Geochim. Cosmochim. Acta, 2017, 208, 171–184 CrossRef CAS.
- R. M. Cory, D. M. McKnight, Y.-P. Chin, P. Miller and C. L. Jaros, Chemical characteristics of fulvic acids from Arctic surface waters: microbial contributions and photochemical transformations, J. Geophys. Res., 2007, 112, 1–14 Search PubMed.
- G. W. Kling, G. W. Kipphut, M. M. Miller and J. W. O'Brien, Integration of lakes and streams in a landscape perspective: the importance of material processing on spatial patterns and temporal coherence, Freshwater Biol., 2000, 43, 477–497 CrossRef.
- J. L. Weishaar, G. R. Aiken, B. A. Bergamaschi, M. S. Fram, R. Fujii and K. Mopper, Evaluation of specific ultraviolet absorbance as an indicator of the chemical composition and reactivity of dissolved organic carbon, Environ. Sci. Technol., 2003, 37, 4702–4708 CrossRef CAS PubMed.
- J. R. Helms, A. Stubbins, J. D. Ritchie, E. C. Minor, D. J. Kieber and K. Mopper, Absorption spectral slopes and slope ratios as indicators of molecular weight, source, and photobleaching of chromophoric dissolved organic matter, Limnol. Oceanogr., 2008, 53, 955–969 CrossRef.
- R. M. Cory and D. M. McKnight, Fluorescence spectroscopy reveals ubiquitous presence of oxidized and reduced quinones in dissolved organic matter, Environ. Sci. Technol., 2005, 39, 8142–8149 CrossRef CAS PubMed.
- M. P. Miller, B. E. Simone, D. M. McKnight, R. M. Cory, M. W. Williams and E. W. Boyer, New light on a dark subject: comment, Aquat. Sci., 2010, 72, 269–275 CrossRef CAS.
- C. P. Ward and R. M. Cory, Chemical composition of dissolved organic matter draining permafrost soils, Geochim. Cosmochim. Acta, 2015, 167, 63–79 CrossRef CAS.
- C. P. Ward, C. M. Sharpless, D. L. Valentine, D. P. French-McCay, C. Aeppli, H. K. White, R. P. Rodgers, K. M. Gosselin, R. K. Nelson and C. M. Reddy, Partial Photochemical Oxidation Was a Dominant Fate of Deepwater Horizon Surface Oil, Environ. Sci. Technol., 2018, 52, 1797–1805 CrossRef CAS PubMed.
- R. M. Cory and G. W. Kling, Interactions between sunlight and microorganisms influence dissolved organic matter degradation along the aquatic continuum, Limnol. Oceanogr. Lett., 2018, 3, 102–116 CrossRef CAS.
- H. Xie, O. C. Zafiriou, W.-J. Cai, R. G. Zepp and Y. Wang, Photooxidation and its effects on the carboxyl content of dissolved organic matter in two coastal rivers in the southeastern United States, Environ. Sci. Technol., 2004, 38, 4113–4119 CrossRef CAS PubMed.
- C. P. Ward, C. M. Sharpless, D. L. Valentine, C. Aeppli, K. M. Sutherland, S. D. Wankel and C. M. Reddy, Oxygen Isotopes (δ18O) Trace Photochemical Hydrocarbon Oxidation at the Sea Surface, Geophys. Res. Lett., 2019, 46, 6745–6754 CrossRef CAS.
- B. T. Neilson, M. B. Cardenas, M. T. O'Connor, M. T. Rasmussen, T. V. King and G. W. Kling, Groundwater Flow and Exchange Across the Land Surface Explain Carbon Export Patterns in Continuous Permafrost Watersheds, Geophys. Res. Lett., 2018, 45, 7596–7605 CrossRef.
- T. M. Kana, C. Darkangelo and M. D. Hunt, Membrane inlet mass spectrometer for rapid high-precision determination of N2, O2, and Ar in environmental water samples, Anal. Chem., 1994, 66, 4166–4170 CrossRef CAS.
- B. P. Koch and T. Dittmar, From mass to structure: an aromaticity index for high-resolution mass data of natural organic matter, Rapid Commun. Mass Spectrom., 2016, 30, 250 CrossRef CAS.
- N. Hertkorn, R. Benner, M. Frommberger, P. Schmitt-Kopplin, M. Witt, K. Kaiser, A. Kettrup and J. I. Hedges, Characterization of a major refractory component of marine dissolved organic matter, Geochim. Cosmochim. Acta, 2006, 70, 2990–3010 CrossRef CAS.
- B. Lam, A. Baer, M. Alaee, B. Lefebvre, A. Moser, A. Williams and A. J. Simpson, Major structural components
in freshwater dissolved organic matter, Environ. Sci. Technol., 2007, 41, 8240–8247 CrossRef CAS PubMed.
- W. C. Hockaday, J. M. Purcell, A. G. Marshall, J. a. Baldock and P. G. Hatcher, Electrospray and photoionization mass spectrometry for the characterization of organic matter in natural waters: a qualitative assessment, Limnol. Oceanogr.: Methods, 2009, 7, 81–95 CrossRef.
- R. G. Wetzel, P. G. Hatcher and T. S. Bianchi, Natural photolysis by ultraviolet irradiance of recalcitrant dissolved organic matter to simple substrates for rapid bacterial metabolism, Limnol. Oceanogr., 1995, 40, 1369–1380 CrossRef CAS.
- D. E. Latch, B. L. Stender, J. L. Packer, W. A. Arnold and K. McNeill, Photochemical fate of pharmaceuticals in the environment: cimetidine and ranitidine, Environ. Sci. Technol., 2003, 37, 3342–3350 CrossRef CAS PubMed.
- M. Grandbois, D. E. Latch and K. Mcneill, Microheterogeneous concentrations of singlet oxygen in natural organic matter isolate solutions, Environ. Sci. Technol., 2008, 42, 9184–9190 CrossRef CAS PubMed.
- S. E. Page, W. a. Arnold and K. McNeill, Assessing the contribution of free hydroxyl radical in organic matter-sensitized photohydroxylation reactions, Environ. Sci. Technol., 2011, 45, 2818–2825 CrossRef CAS PubMed.
- D. E. LaRowe and P. Van Cappellen, Degradation of natural organic matter: a thermodynamic analysis, Geochim. Cosmochim. Acta, 2011, 75, 2030–2042 CrossRef CAS.
- M. Xu and P. Wan, Efficient photodecarboxylation of aroyl-substituted phenylacetic acids in aqueous solution: a general photochemical reaction, Chem. Commun., 2000, 2147–2148 RSC.
- C. Miles and P. Brezonik, Oxygen consumption in humic-colored waters by a photochemical ferrous-ferric catalytic cycle, Environ. Sci. Technol., 1981, 15, 1089–1095 CrossRef CAS PubMed.
- D. Budac and P. Wan, Photodecarboxylation: mechanism and synthetic utility, J. Photochem. Photobiol., A, 1992, 67, 135–166 CrossRef CAS.
- Y. Gu, A. Lensu, S. Perämäki, A. Ojala and A. V. Vähätalo, Iron and pH Regulating the Photochemical Mineralization of Dissolved Organic Carbon, ACS Omega, 2017, 2, 1905–1914 CrossRef CAS PubMed.
- V. Amaral, D. Graeber, D. Calliari and C. Alonso, Strong linkages between DOM optical properties and main clades of aquatic bacteria, Limnol. Oceanogr., 2016, 61, 906–918 CrossRef CAS.
- B. Crump, G. Kling, M. Bahr and J. Hobbie, Bacterioplankton community shifts in an arctic lake correlate with seasonal changes in organic matter source, Appl. Environ. Microbiol., 2003, 69, 2253–2268 CrossRef PubMed.
- B. C. Crump, B. J. Peterson, P. A. Raymond, R. M. W. Amon, A. Rinehart, J. W. McClelland and R. M. Holmes, Circumpolar synchrony in big river bacterioplankton, Proc. Natl. Acad. Sci. U. S. A., 2009, 106, 21208–21212 CrossRef CAS PubMed.
- K. E. Judd, B. C. Crump and G. W. Kling, Variation in dissolved organic matter controls bacterial production and community composition, Ecology, 2006, 87, 2068–2079 CrossRef PubMed.
- D. L. Kirchman, A. I. Dittel, S. E. G. Findlay and D. Fischer, Changes in bacterial activity and community structure in response to dissolved organic matter in the Hudson River, New York, Aquat. Microb. Ecol., 2004, 35, 243–257 CrossRef.
- H. J. Smith, M. Dieser, D. M. McKnight, M. D. SanClements and C. M. Foreman, Relationship between dissolved organic matter quality and microbial community composition across polar glacial environments, FEMS Microbiol. Ecol., 2018, 94, fiy090 CAS.
- J. C. Bowen, L. A. Kaplan and R. M. Cory, Photodegradation disproportionately impacts biodegradation of semi-labile DOM in streams, Limnol. Oceanogr., 2019, 1–14 CAS.
- M. A. Moran and R. G. Zepp, Role of photoreactions in the formation of biologically compounds from dissolved organic matter, Limnol. Oceanogr., 1997, 42, 1307–1316 CrossRef CAS.
Footnote |
† Electronic supplementary information (ESI) available. See DOI: 10.1039/c9em00504h |
|
This journal is © The Royal Society of Chemistry 2020 |