DOI:
10.1039/D0DT01517B
(Paper)
Dalton Trans., 2020,
49, 9239-9253
Zero-concentration quenching: a novel Eu3+ based red phosphor with non-layered crystal structure for white LEDs and NaSrY(MoO4)3:Sm3+ based deep-red LEDs for plant growth†
Received
25th April 2020
, Accepted 26th May 2020
First published on 26th May 2020
Abstract
Oxide based highly efficient narrow band red emitting phosphors are still a bottleneck in white LED applications. Trivalent europium ion based phosphors could be a better choice, however their weak oscillator strength restricts their use in white light emitting diodes (LEDs). Herein, we report a novel red emitting NaSrEu(MoO4)3 (NSEuM) phosphor with zero concentration quenching (non-layered crystal structure). The phosphors (NaSrY1−xEux(MoO4)3, x = 0.1–1, in increments of 0.1) were synthesized through a traditional solid-state reaction and their phase formations were analyzed by powder X-ray diffraction (PXRD) followed by Rietveld refinement. Under 395 nm excitation, all the phosphors showed sharp emission at 616 nm (full width at half maximum, FWHM ∼4–5 nm) owing to the 5D0 → 7F2 electric dipole transition of the Eu3+ ion. A concentration dependent photoluminescence (PL) study revealed that there is no concentration quenching of the systems, leading to them having superior emission characteristics over those of commercial red phosphors as well as a reported Eu3+ phosphor with a layered structure. The color purity of the synthesized phosphor was observed to be 96.32% and it shows excellent thermal stability at 423 K, retaining 64.6% of the emission intensity of its initial room temperature. The NSEuM phosphor shows a high absolute quantum yield of 79.7%. Besides this, a red LED (near UV (NUV) LED chip with the NaSrEu(MoO4)3 phosphor) as well as a hybrid white LED (NUV LED chip with an organic yellow dye + red NSEuM phosphor) were fabricated and their optical properties were studied. After the inclusion of the red phosphor in the hybrid white LED, the color rendering index (CRI)/correlated color temperature (CCT) were improved significantly (60/9333 K vs. 79/6004 K, respectively). In addition, to show the potential use of the system in plant growth application, we systematically investigated the Sm3+ activation in NaSrY(MoO4)3 and found that the phosphor shows orange red emission with an intense deep red emission (645 nm (4G5/2 → 6H9/2)). We fabricated a hybrid red/deep red LED by integrating a NUV LED with a mixed Sm3+ and Eu3+ phosphor and the spectral lines were well matched with the phytochrome (Pr) absorption spectrum. The presently investigated phosphor showed potential in a white LED as well as a deep red/orange-red LED for plant growth.
Introduction
High performance red emitting oxide phosphors are still a bottleneck in the area of efficient white light emitting diodes (LEDs).1,2 Line-like red emission can be produced by organo-europium complexes, but the design and synthesis of suitable ancillary ligands is still a difficult task. Thus, solid state phosphor materials have gained much attention.3,4 Most the red phosphors that have been reported for LED applications are based on sulfides or nitrides (including oxy-nitrides). However, both host lattices have significant problem in terms of stability as well as synthetic conditions. To overcome this, numerous oxide based red emitting phosphors were synthesized and their optical properties studied. Oxide based red phosphors are easy to synthesize, and have high thermal stability and chemical stability, whereas other hosts require tough synthetic procedures and have low chemical stability. Apart from Eu2+ based sulfides and nitrides, the trivalent europium (Eu3+) ion has attracted great attention in the production of red emission (λmax = 610–620 nm, owing to its 5D0 → 7F2 electric dipole (ED) transition) with a small full width at half maximum (FWHM) (highly essential for getting a better luminous output).5 It is also worth noting that Eu3+ activated red phosphors can effectively be synthesized in an air atmosphere, which diminishes the manufacturing costs compared to those of Eu2+ activated red phosphors. However, the oscillator strength of the Eu3+ ion is very poor, due to 4f–4f electronic transitions (forbidden ones) and it has low absorption in the near ultraviolet (NUV) region, where LED emission occurs. The most difficult task is to decrease the loss of NUV excitation and convert it into red light. However, much as could be expected, this has not yet been appropriately resolved. In order to enhance the absorption strength and overall luminescence efficiency of the Eu3+ based phosphor, a suitable host lattice needs to be found. This host lattice should absorb the incident photons from the LED and convert them into red emission. The approach has two steps: one is to find a suitable lattice which has a charge transfer band in the near UV region so that it can participate in the energy transfer process, the second way is to find a lattice which can accommodate a rich concentration of Eu3+ ion with zero concentration quenching.
Simultaneous achievement of good charge transfer band and zero concentration quenching in a Eu3+ substituted oxide based host lattice has rarely been reported. The former can be achieved by having a host lattice with a MO6 or MO4 group (M = Mo, W, good absorption in the NUV region due to a metal–oxygen charge transfer (CT) band), whereas the latter can be achieved with a lattice with a layered structure. The replacement of tungsten with molybdenum in a host lattice leads to a shift in the absorption edge towards the NUV region, due to their difference in electronegativity. For example, in the case of AgGd(MO4) and its solid solution with a scheelite structure (structure consisting of tetrahedra (MO4)), the edge is shifted from 320 to 350 nm,5,6 whereas in its double perovskite structure (structure consisting of octahedra (MO6)), the edge is shifted from 380 to 420 nm.7–10 Further shifting of the absorption edge towards the soft UV/blue region is highly challenging. Hence, it is important to search for a lattice which can accommodate rich Eu ions in the structure with non-concentration quenching. Fig. 1 shows a schematic representation of the MO4 to MO6 energy level diagram.1,11 There are three categories of the host lattice for Eu3+ activation or substitution to achieve high emission intensity: (i) low Eu3+ doping, where the concentration quenching (CQ) could occur (few mole percentage),12 (ii) medium Eu3+ doping, CQ could occur at ∼50%,13 (iii) rich Eu3+ substitution, zero or negligible CQ.14 In particular, the Eu3+ rich host lattice can improve the absorption or oscillator strength of the Eu3+ ion (5L6–7F0 (∼394 nm) and 5D2–7F0 (∼465 nm) choices of interest). A schematic representation of the Eu3+ ions (low, medium and high concentrations) in the host lattice and their concentration quenching behavior is shown in Fig. 2.
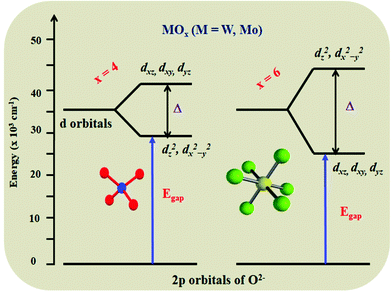 |
| Fig. 1 Schematic representation of the MO4 to MO6 energy level diagram. | |
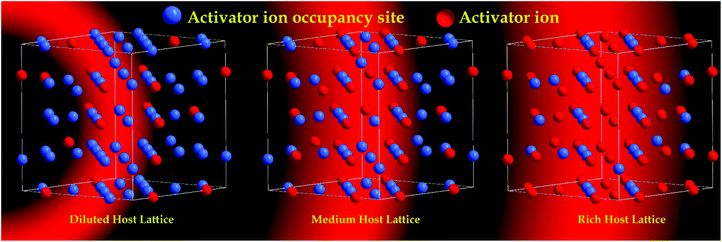 |
| Fig. 2 Schematic representation of the Eu3+ ions (low, medium and high concentrations) in a host lattice and their concentration quenching behavior. | |
The main objective of the present study is to design and synthesize a highly efficient red phosphor to increase the overall efficiency of a white LED by achieving a high concentration of the activator ion in the host lattice. However, a few layered structured has previously been reported with no concentration quenching of the Eu3+ ion. Our group recently studied Li3BaSrLn3(WO4)8 (Ln = Y, La, Gd; M = W and Mo) and its solid solution with a stratified scheelite structure as a potential red emitting phosphor for warm white LEDs that has nearly zero concentration quenching, where certain compositions even show zero thermal quenching.15–17 For example, in a Li3BaSrGd3−xEux(MO4)8 (M = W and Mo) phosphor, the shortest distance between Gd3+ is 3.909 Å, whereas the distance varies significantly in the different layers (i.e.) the shortest distance between Gd3+ is 8.292 Å and they are isolated by MO4 groups. A greater distance between Eu–Eu in different layers favours zero concentration quenching.16 Very recently, non-concentration quenching was found in a Eu3+ substituted layer structured Ba6Gd2Ti4O17 host lattice.18 This further motivated us to find a layered or non-layered host lattice that can incorporate a higher concentration of Eu3+.
The other imperative area of application for red or deep LED emissive devices is that they have potential to be used for plant growth. In general, light delivers enough energy at ambient temperature to the environment for plant growth via photosynthesis, which is viewed as one of the most vital natural parameters for plant growth and advancement.19–22 Besides this, it controls many plant cell processes, since blue light (400–500 nm) promotes photosynthesis, red light (610–690 nm) is responsible for phototropism and far-red light (700–740 nm) is responsible for photomorphogenesis processes.23,24 Solid-state lighting plays an important role in the plant growth process, since its spectral wavelength overlaps with plant photoreceptors and promotes plant growth production.25,26 Specifically, red LEDs have gained great attention due to their overlap of LEDs with phytochrome (Pr) absorption. Aside from Mn4+ red phosphors, Eu3+ activated red phosphors also influence the growth of plants.27
Very recently, we have explored a Eu3+ activated NaSrLa(MO4)3 (M = Mo and W) red emitting phosphor for white light emitting diode (WLED) applications. The NaSrLa(MoO4)3 host lattice showed concentration quenching at 80%, whereas the NaSrLa(WO4)3 host lattice exhibited zero concentration quenching.28 Specifically, the molybdenum containing host lattice showed promising NUV absorption, good thermal stability and absolute quantum efficiency. These excellent outcomes triggered us to further examine the same host lattice with different substitutions in the La (active) site. Up to now, there have been no reports on Eu3+ activated NaSrY(MoO4)3 (NSYM) red phosphors. However, a slight modification to the host lattice will have a great impact on the photophysical properties. In this present study, Eu3+ doped NSYM red phosphors were synthesized via a traditional solid-state route. The NSYM:Eu3+ phosphors exhibited intense red emission at 616 nm under excitation at 395 nm. Also, other optical properties such as the asymmetric ratio, concentration quenching, Judd–Ofelt (J–O) calculation, and lifetime and thermal stability were also examined in detail. The occurrence of non-concentration quenching in the present host lattice was explained from a crystallographic point-of-view. Finally, a red LED was fabricated using the synthesized red phosphor with a NUV LED chip. These results revealed that the obtained NaSrEu(MoO4)3 phosphor could be a potential red component for use in WLEDs. Efforts have also been made to fabricate a hybrid white LED via the combination of a blue LED + yellow organic dye + red NaSrEu(MoO4)3 phosphor. This combination shows a superior color rendering index (CRI) and Commission Internationale d'Eclairage (CIE) value compared to that of a hybrid white LED without a red phosphor. In addition, the emission intensity of the currently synthesized phosphor was also compared with that of a red phosphor with a layered structure (Ba6Gd2Ti4O17:Eu3+).18 The emission of the fabricated red LED matches the Pr absorption spectrum. However, in order to cover the entire range, we attempted to synthesis a Sm3+ activated NSYM phosphor, to utilize for the first time the deep red emission at 645 nm for plant growth (due to 4G5/2 → 6H9/2, choice of interest for plant growth). A systematic investigation revealed that the Sm3+ and Eu3+ phosphor combined with NUV LED efficiently covered the entire region of Pr absorption. The synthetic procedures of the phosphor and physico-chemical characterization techniques are given in the ESI.†
Results and discussion
Crystal structure and phase analysis
The NaSrRE(MoO4)3 (RE = Y and Eu) phosphors were crystallized in the tetragonal phase29 in the space group I41/a, where the parameters of the phosphors are very near to those of CaMoO4
30 and Na0.5La0.5MoO4.31 The powder X-ray diffraction (PXRD) patterns of all the phosphors were found to be consistent with the standard Joint Committee on Powder Diffraction Standards (JCPDS) pattern (08-0490) and no other impurity diffraction peaks were observed, which suggests that Eu3+ ions were effectively incorporated at the Y3+ sites (Fig. 3a). Fig. 3c exhibits the Rietveld refinement of the NSYM phosphor with low R factor values and the refined parameters are presented in Table 1. The NSYM phosphor shows a shift in diffraction peaks to the right with respect to those of JCPDS (08-0490) (Fig. 3b), which can be explained by the Shannon and Prewitt table.32 The diffraction peaks were moved towards a lower angle upon enhancing the Eu3+ ion concentration in the NYSM host lattice. When the Eu3+ ion concentration in the NYSM host lattice was increased, the diffraction peaks shifted towards a lower angle. The shift in the diffraction peaks was observed possibly due to the difference in the ionic radius of Y3+ and Eu3+ for a corresponding 8-coordination site. The activator Eu3+ ion (ionic radius = 1.066 Å) favors the occupation of Y3+ (ionic radius = 1.019 Å) sites owing to the similar ionic radii of these Ln3+ ions.32 Upon the substitution of Eu3+ ions in the Y3+ site, the diffraction peaks were shifted towards to a lower angle. Doping the larger Eu3+ ions in the place of Y3+ leads to an obvious expansion of the lattice owing to the larger ionic radii of the Eu3+ ions. Therefore, the lattices of the above Eu3+ containing materials will be bigger than that of undoped systems. This implies that the ‘d’ spacings within the lattice will also change. Because of this, there is a shift in the 2θ position towards lower 2θ values.
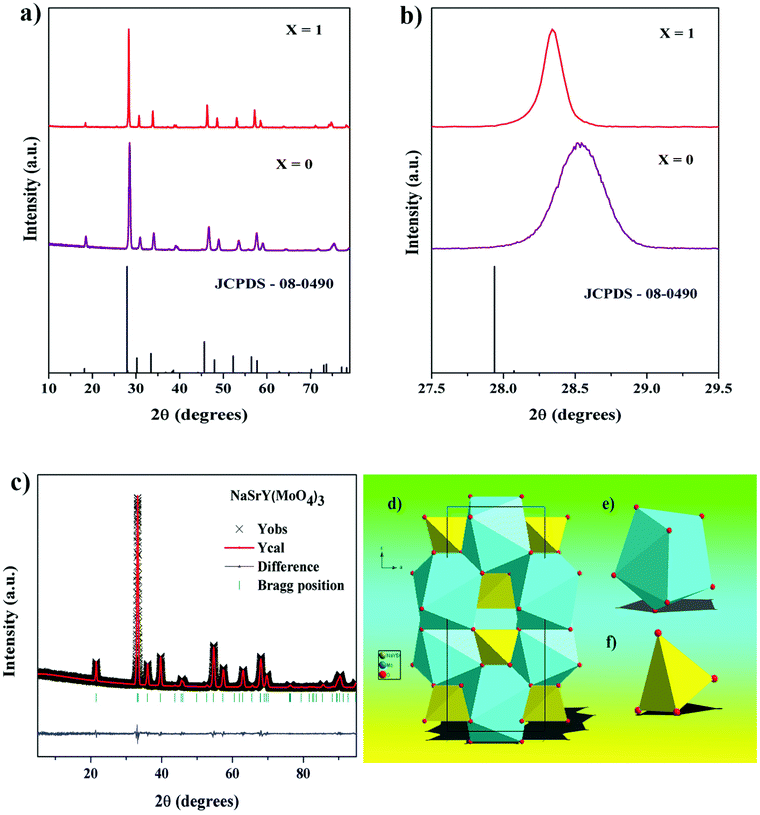 |
| Fig. 3 (a) PXRD pattern of the Eu3+ activated NYSM, (b) magnified PXRD pattern from 27.5°–29.5° and (c) Rietveld refinement of the NSYM phosphor. (d) Crystal structure of the NSYM phosphor, (e and f) coordination and bond lengths of Na/Sr/Y–O8 and Mo–O4, respectively. | |
Table 1 Refinement parameters of the NSYM phosphors
Compound |
NSYM |
Crystal system |
Trigonal |
Space group |
I41/a (88) |
a & b (Å) |
5.2747 (1) |
c (Å) |
11.5872 (3) |
α = β = γ |
90° |
V (Å3) |
322.4 (1) |
Z
|
4 |
2θ-Interval |
10–100° |
R
wp, % |
8.83 |
R
p, % |
6.33 |
GOF |
1.23 |
Fig. 3d shows the crystal structure of NSYM, which is identical to the structure of CaMoO4.30 In this crystal structure, the Na, Sr, and Y occupy one cation site named as M, which is shared by 1/3 of each atom and coordinated by nine oxygen atoms. The coordinations of M–O and Mo–O are shown in Fig. 3e and f, respectively. The distances of the M–O and Mo–O bonds are 2.522 to 2.533 Å and 1.717 Å, respectively. The MoO4 tetrahedra are coordinated by four (Sr/Na/Y)O8 square antiprisms.33
Surface morphology studies
Fig. S1(a–d)† shows a scanning electron microscopy (SEM) micrograph of the NSYM and NaSrEu(MoO4)3 (NSEuM) phosphors, which indicates that the phosphor particles are in the micro range and are agglomerated. It can be seen that the NSEuM phosphor particle size is larger than that of the NSYM phosphor, which arises due to Eu3+ having a greater ionic radius Y3+ in the host lattice.34–36 Fig. S1e and f† exhibit the elemental mapping and energy-dispersive X-ray spectroscopy (EDX) of the NSYM phosphor, respectively. The elemental mapping shows that all the elements are homogenously distributed in the surface. The EDX spectrum confirms the presence of all the elements in the phosphor. The EDX spectra display that a maximum amount of Na and O ions are employed in the K shell at around 1.2 and 0.5 keV, respectively. Other elements, such as Sr, Y and Mo, are employed in the L shell at 2, 2.1 and 2.5 keV, respectively.
Fourier-transform IR spectroscopy study
Detection of the molybdate stretching frequency was achieved by recording the FT-IR spectrum of NSYM (Fig. S2†). The stretching vibration of the O–Mo–O of MoO4 was characterised by the presence of a strong and intense peak at around 438.3 cm−1. Mostly, the MoO4 group stretching vibrations are observed at less than 500 cm−1 along with those of the Y–O bonds.37 There may be a feasible overlap between the MoO4 group and Y–O stretching vibrations. The solid band at 830 and 852 cm−1 in the spectra was designated to the stretching vibrations of the Mo–O bond.38 On account of the existence of H2O molecules in the environment, a stretching (O–H) band at around 1650 cm−1 was detected in the spectrum.
Photoluminescence properties, asymmetric ratio and concentration quenching studies
Fig. 4a illustrates the excitation spectrum of NSEuM phosphors ranging from 220 to 350 nm and a series of intense lines from 350 to 550 nm. The broad band in the spectrum is a result of the charge transfer (CT) absorption, whereas the sharp lines are indicative of the Eu3+ ion 4f–4f electronic transition. Here, the CT band is represents the electronic transition from O2− (2p-orbital) to Eu3+ (4f-orbital) and the transition from the O2− (2p orbital) to Mo6+ (5d orbital) in the MoO42− group.39 It is also possible to estimate the ligand to metal charge transfer (LMCT) band using the empirical formula proposed by Reisfeld and Jorgensen.40 | Ect (cm−1) = [χopt(X) − (χopt(M)] × 30 000 cm−1 | (1) |
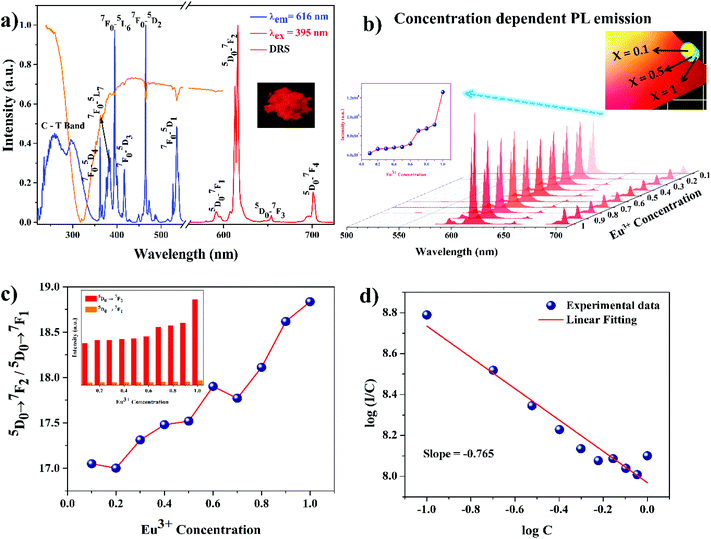 |
| Fig. 4 (a) PL excitation (λem = 616 nm), emission (λexc = 395 nm) and DRS spectra of the NSEuM phosphor, (b) PL emission spectra of the NSYM:Eu3+ phosphors, (c) asymmetric ratio calculation of the NSYM:Eu3+ phosphor, (d) linear fitting of log(C) vs. log(I/C) for the NSYM:Eu3+ phosphors. | |
Here, χopt(X) refers to the optical electronegativity of the anion, which is equal to the Pauling electronegativity value, 3.2 and χopt(M) corresponds to the optical electronegativity of the central metal ion (Mo = 2.10121 and Eu = 1.74).41 From eqn (1), the LMCT of O2−–Mo6+ is around ∼303 nm and the O2−–Eu3+ LMCT is at ∼228 nm. The calculation clearly indicates that the estimations agree well with the experimental results. However, emission of the MoO42− group does not occur in the emission spectrum, which indicates that the MoO42− group transfers absorbed energy to the Eu3+ ion non-radiatively. This is the well-known phenomenon of host sensitized energy transfer.42 This can be further confirmed by the appearance of the CT band of MoO4 in the excitation spectrum upon monitoring the Eu3+ emission (ED transition). With regards to the 4f–4f electronic transition of Eu3+, the intense peak at 395 nm is due to 7F0 → 5L6. The other sharp peaks at 366, 464, and 535 nm can be assigned to the 7F0 → 5D4, 7F0 → 5D2 and 7F0 → 5D1 transitions of the Eu3+ ion, respectively. The peaks at 395 and 465 nm are much greater in intensity than the other peaks in the excitation spectrum. These lines require special consideration because the peaks lie in the NUV and blue spectral region. Besides this, the diffuse reflectance spectroscopy (DRS) spectrum shows good overlap with the PL excitation spectrum. The concentration dependent excitation spectrum is shown in Fig. S3a.† Upon increasing the concentration of the Eu3+ ions, the absorption strength at ∼395 and ∼465 nm is greatly enhanced, which is essential for LEDs (Fig. S3b†). Fig. 4a shows the emission spectra of the NSEuM phosphors under excitation at 395 nm. The emission at 616 nm can be attributed to the 5D0 → 7F2 electronic transition of the Eu3+ ion. Some minor peaks are observed at 579–598, 653 and 701 nm, which can be assigned to the 5D0 → 7F1, 5D0 → 7F3 and 5D0 → 7F4 electronic transitions of the Eu3+ ions, respectively. All the Eu3+ doped phosphors exhibited a sharp red emission at 616 nm, while upon increasing the concentration of Eu3+ ions the emission intensity also enhanced gradually, with a maximum at x = 1 (Fig. 4b). No concentration quenching was observed upon Eu3+ ion doping (an explanation for this is given in the concentration quenching studies). Upon the varying the Eu3+ ion concentration in the lattice, no changes were observed in the nature of the emission.
Some of the electronic transitions are highly sensitive to the crystal lattice surroundings and are termed as hypersensitive transitions. The (5D0 → 7F2) electric dipole transition (ED) is highly hypersensitive, and the ligand ions in the crystals affect this ED transition intensity.5 In general, when the Eu3+ ions occupy in a non-centrosymmetric site, the 5D0–7F2 emission transition rules in the emission spectrum.43 As a result of the occupancy of the Eu3+ ion at the inversion site in the NSYM lattice, the emission intensity of the ED (5D0–7F2) transition is greater than the magnetic dipole (MD) (5D0–7F1) transition.
In terms of the local structural characteristics, the asymmetric ratio (β) can be a useful parameter. The asymmetric ratio (AR) is defined as the intensity ratio between the ED and MD transitions. Fig. 4c shows the AR of the NSYM:Eu3+ phosphors, which provides an assessment of the distorted environment around the Eu3+ ions in the host lattice.44–46 The ARs of all the synthesised phosphors shown are greater than 1, which indicates that the Eu3+ ions are non-centrosymmetric in the presently studied host lattice. Moreover, the AR of NSEuM is 18.8354, whereas the AR of a commercial Y2O3:Eu3+ phosphor is 8.75,47 which indicates that the as-synthesised phosphor AR is much better than the commercial phosphor. The AR keeps on increasing from 17.0489 to 18.8354 with an increase in doping concentration, indicating that the current phosphors show greatly saturated red emission.
The luminescence characteristics and nature of phosphor materials are affected by the activator (Eu3+) ion doping concentration.48 Hence, the optimal doping concentration of Eu3+ ions needs to be determined. The linear relationship between the relative emission intensity of the 5D0 → 7F2 transition and Eu3+ ion concentration in the NSYM phosphor under excitation at 395 nm is exhibited in the Fig. 4b inset. The relative emission intensity of the 5D0 → 7F2 transition increases along with the change in the concentration of the Eu3+ ions in the present system, and achieves a maximum upon the full doping with Eu3+ ions. No concentration quenching was observed, which implies that the crystal structure (arrangement of the activator ions) plays a vital role. A similar observation was also made in our previous studies.16,29 In depth, the concentration quenching phenomenon can be explained by careful observation of the host lattice crystal structure, specifically, the bond distance of Y–O and Eu–O in the lattice. Fig. 5a and b illustrate the non-layer structures (2 × 2 × 2) of the NSYM and NSEuM phosphors along the a-axis directions, respectively. The inter layer Y sites distance is around 9.0649 Å (horizontal layer) and 5.2643 Å (vertical layer), whereas the Eu site distance is around 9.1254 Å (horizontal layer) and 5.2940 Å (vertical layer), which indicates that the horizontal and vertical bond distances of the Y sites increase upon substituting with the Eu ion. Also, in the single unit cell (ab plane) the intra layer distances of the Y and Eu sites are around 3.9101 and 3.9345 Å, respectively (Fig. 5c and d). Moreover, the Y–O bond distances are in the range of 2.517–2.528 Å (Fig. 5e), while the Eu–O bond distances in the NSEuM phosphor are in the range of 2.531–2.544 Å (Fig. 5f). The bond distance is notably enhanced on going from the Y to the Eu site and it restrains the energy migration direction, which could restrict the occurrence of concentration quenching and facilitate the zero concentration quenching in the lattice.
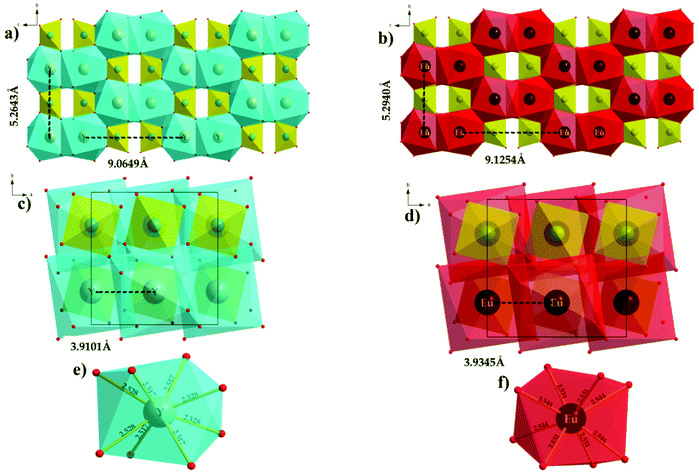 |
| Fig. 5 Crystal structures of (a) NSYM and (b) NSEuM indicating the bond distance/lengths (a and b) intra layer Y/Eu–O distances, (c and d) interlayer distances of Y/Eu–O, and (e and f) Y/EuO8 polyhedra. | |
The interpretation of the energy transfer mechanism entails knowing fundamental information of the critical distance (Rc), so using the Blasse equation the critical distance was calculated between two adjacent Eu3+ ions in the lattice:
| 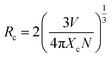 | (2) |
In eqn (2), V defines the unit cell volume, Rc denotes the critical distance, Xc is the critical concentration and N is the number of vacant cationic sites in the unit cell that can be occupied by activator ions.49,50 Here, V is taken to be 326.3 Å3, Xc = 1.0 (because non concentration quenching occurs, so x = 1.0) and the number of vacant available cationic sites for the Eu3+ is 4. The concentration at which the non-radiative transfer rate is equivalent to the internal decay rate (radiative rate) is known as concentration quenching.51 The critical distance of the present system was found to be Rc = 5.382 Å, the obtained value is close to 5 Å, which indicates that the multipolar interactions are the most important factor in concentration quenching.
Huang et al. gave theoretical expressions for a plot of luminescence intensity of an ED transition against the doping concentration of activator ions,52 which are:
| 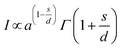 | (3) |
| 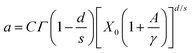 | (4) |
where
A and
X0 are constants, and
Γ(1 +
s/
d) is a
Γ function,
γ is the intrinsic transition probability of the sensitizer and
s is the index for electric multipole, electric dipole–dipole (D–D), electric dipole–quadrupole (D–Q), and electric quadrupole–quadrupole (Q–Q)interactions; when
s = 6, 8, or 10, respectively.
| 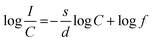 | (5) |
In eqn (5) (inferred from eqn (3) and (4)), c defines the concentration of Eu3+ in the host lattice and f is independent of doping concentration. Fig. 4d shows a plot of log(I/C) vs. log(C) and as per the above equation, calculations were performed for the 5D0 → 7F2 electric dipole transitions (616 nm) of the Eu3+ ions in the NSYM red phosphors. Upon using linear fitting, the slope (−s/d) was calculated to be −0.765, which is almost near 1 (i.e., s = 3). The calculated slope value and its s value clarifies that energy transfer is occurring between the Eu3+ ions in the NSYM phosphors.
Energy transfer study
Fig. S4† illustrates the schematic energy transfer phenomenon that occurs from MoO42− groups to Eu3+ ions in the NSYM system. For the most part, the MoO42− group shows a solid tendency to procure electrons in large numbers from an oxygen ligand, in this way the least measure of energy is adequate for the LMCT process. Upon NUV light excitation, the MoO42− group absorbs the photons of the UV light and gets excited to the 1T1 excited state from the 1A1 ground state, afterwards the excited state energy transfers to the Eu3+ ion 5D0 excited state non-radiatively. Subsequently, the energy comes to the Eu3+ ions ground states (7F1, 7F2, 7F3 and 7F4) from the first excited (5D0) state of the Eu3+ ion via radiative transition.53,54
Judd–Ofelt (J–O) parameter calculations
Judd–Ofelt theory is an important tool for studying the impact that the chemical environment has on the luminescence properties of Eu3+ ions. Investigation of the Judd–Ofelt parameters unveils information about the parity-forbidden rates of the ED radiative transition between the various levels of the lanthanide ions.51,55 By taking the 5D0–7F1 transition as a reference, the intensity parameters for the 5D0–7F2 and 5D0–7F4 transitions were calculated, and all other relevant results were deduced in previous reports.56,57 The determined J–O spectral parameters are given in Table ST1.† The Ω2 values being higher than Ω4 indicates that the 5D0 → 7F2 transition is dominant. The Ω2 parameter shows dependence on the covalency between Ln3+ cations and ligand field anions, which reveal about the local environment of the Eu3+ site and its asymmetry in the lattice. Hence, Ω2 is very sensitive to the ligand environment, as can be observed via the changes made to the Eu3+ ion concentration. A higher value of Ω2 implies greater asymmetry or high covalency of the Eu3+ ions and ligand bonds in the present host lattice. Variation in the Ω2 parameters was detected owing to the distortions that exist around the occupied Eu3+ ion sites.
PL decay curve analysis
The decay curves of the NSYM:Eu3+ phosphors were measured under excitation at 395 nm along with the corresponding lifetime values for each Eu3+ concentration, as shown in Fig. 6. By using the single exponential decay function all of the decay curves of the samples were fitted using the single exponential expression shown below: |  | (6) |
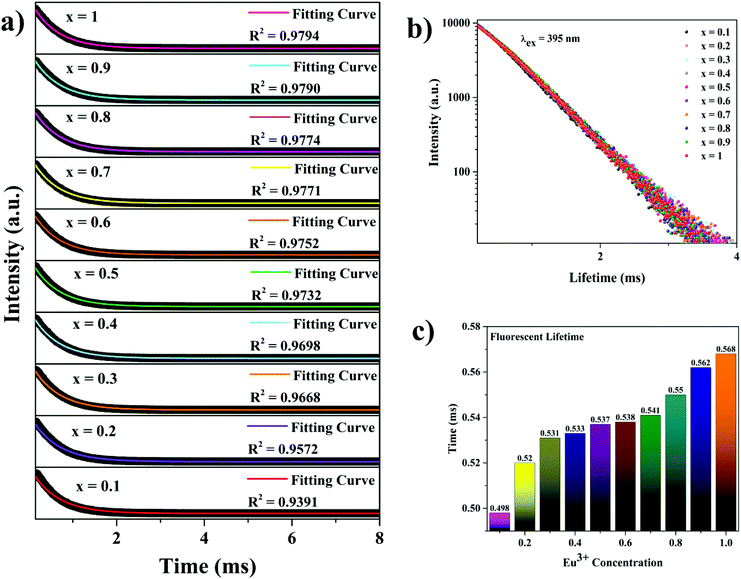 |
| Fig. 6 (a) Decay curve analysis of the NSYM:Eu3+ red phosphors under excitation at 395 nm with first exponential fitting. (b) The linear behaviour of the logarithmmic decay curves. (c) A lifetime value bar diagram of the NSYM:Eu3+ red phosphors. | |
Here, I0 represents the initial intensity, I(t) is the intensity at a given time t and τ denotes the lifetime of the phosphor.58 With enhanced Eu3+ concentration in the NSYM host lattice, the lifetime values increased from 0.498 to 0.568 ms, which could be attributed to efficient energy transfer occurring among the Eu3+ ions in the lattice.
Thermal properties of the NSEuM phosphor
Thermal stability is one of the most important pre-requisites of the synthesized phosphor to be used in a LED, as temperature greatly affects the emission output and CRI. Fig. 7a shows the thermal stability spectrum of the NSEuM phosphor monitored in the range of 298–483 K under excitation at 395 nm. As seen from Fig. 7a, the PL intensity diminishes with increasing temperature owing to the effect of thermal quenching, since the emission peak locations were constant. Fig. S5† shows the configuration diagram for the thermal quenching phenomenon. At high temperature, the electron–phonon interactions are estimated to be greater. Initially, phonon communication activated the Eu3+ ions in the excited level. The route for the 5D0 excited state thermal quenching is via the Eu3+–O2− charge transfer state band. With the assistance of phonons, a few electrons can overcome the activation energy upon an increase in the temperature and feed into the 7FJ states, resulting in a nonradiative process. Hence, the emission intensity diminishes as the temperature increases.59Fig. 7b shows a plot of PL intensity vs. temperature where the PL intensity at 483 K is approximately half of that at 283 K (room temperature). The NSEuM phosphor exhibits an excellent thermal stability, retaining 64.2% at 423 K. For a better understanding of the impact of temperature on the PL emission intensity of the phosphors, the energy of activation (Ea) was determined using the Arrhenius equation: | 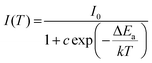 | (7) |
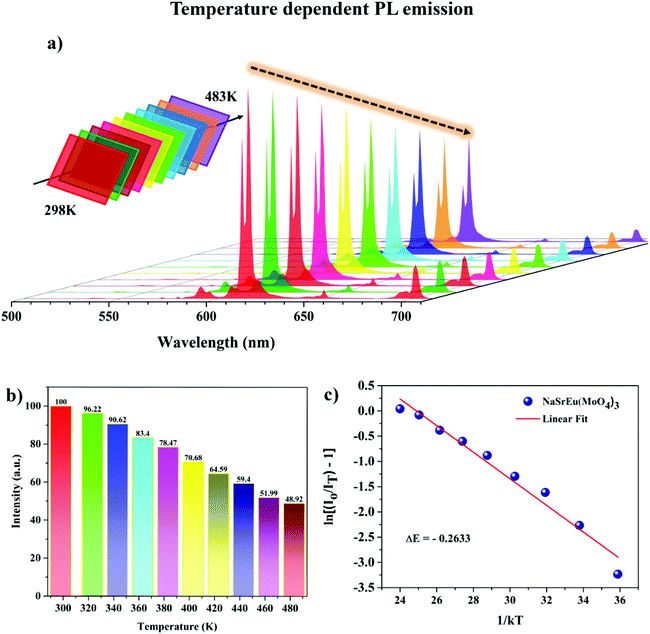 |
| Fig. 7 (a) Temperature dependent PL spectra of NSEuM under 395 nm. (b) Normalized intensity of the 615 nm emission peak vs. temperature. (c) Plot of ln[(I0/I) − 1] vs. 1/kT for the NSEuM phosphor. | |
In eqn (7), I0 is related to the PL emission initial intensity of the phosphor at room temperature, I(T) is the PL intensity at different temperatures, c is a constant for the host, ΔEa denotes the energy of activation for thermal quenching and k denotes the Boltzmann constant in eV K−1. Upon specific rearrangements, eqn (7) can be rewritten as:
| 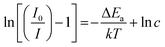 | (8) |
To calculate the Ea, ln[(I0/I) − 1] vs. 1/kT was plotted and is depicted in Fig. 7c. The slope of the fitting line was calculated to be −0.263, the energy of activation was determined from the slope and the value was found to be ∼0.263. The obtained energy of activation value is much greater than that of other Eu3+ activated red phosphors such as CaB2Si2O8, Ba2LiGa(P2O7)2 and Li3Ba2La3(WO4)8 (ΔEa: 0.195, 0.238 and 0.24 eV).60–62 The greater energy of activation indicates that the currently synthesized phosphor has higher thermal stability. This concludes that the as-synthesized phosphor shows better thermal stability and can be used in WLEDs.
CIE color coordinates, color purity study and quantum yield
From the emission spectra of the NSYM:Eu3+ phosphors, the CIE coordinates were calculated and the CIE plot is shown in the Fig. 4a inset. All the Eu3+ activated NSYM phosphors exhibited red emission with good color saturation and these chromaticity coordinates are in good agreement with the National Television System Committee (NTSC) standards.63 Among all the phosphor chromaticity coordinates, the NSEuM phosphor chromaticity coordinate values indicate that it has better red emission than that of other phosphors, also the CIE value is the nearest to the NTSC standard value (x = 0.67, y = 0.33). In order to analyze the potential application of the synthesized phosphor, the NSEuM red phosphor was compared with Eu3+ activated commercial red phosphors such as Y2O3 (Nichia) and Y2O2S (Nichia) (in the absence of commercial narrow band red emission phosphors). The comparative emission spectra (λex = 395 nm) of the NSEuM, Y2O3 and Y2O2S phosphors are depicted in Fig. S6a.†
These results conclude that the synthesized phosphor could be an efficient red phosphor for use in WLED fabrication. Besides this, the emission spectrum of the non-layered structure NSEuM phosphor was compared with that of the recently reported layer structured Ba6Gd2Ti4O17:Eu3+ phosphor (Fig. S6b†). Comparatively, the presently studied phosphor with a non-layered structure shows higher red emission than that of the layer structured phosphor and the corresponding CIE is also shown in the Fig. S6b† inset. It clearly indicates that the red colored saturation of the non-layered system is more superior compared with the reported red phosphor with a layered structure.
Furthermore, the color purity of the NSEuM phosphor was determined to better understand the emissive color properties, which can be determined via the below equation:64,65
| 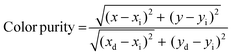 | (9) |
In the above equation, (x, y) denotes the CIE chromaticity coordinates of the NSEuM phosphor. (xi, yi) represents the CIE white light illumination (xi = 0.310, yi = 0.316,) and the (xd, yd) values refer to the chromaticity coordinate dominant wavelength points (xd = 0.682, yd = 0.317). In the present study, the obtained CIE values for the NSEuM phosphor are x = 0.668, y = 0.331, and thus the color purity was found to be 96.32%. Fig. S6c† illustrates the color purity of all of the red phosphors, which exhibit values of greater than 93.6%. The presently studied NSEuM phosphor exhibits excellent color purity over other reputed red phosphors (KBaGd(MoO4)3:Eu3+ (94%)66 and SrMoO4:Eu3+ (85.8%)).67 This result suggests that the NSEuM red phosphor shows superior color coordinates with greater color purity, which indicates that the phosphor can perform as a suitable red component in WLEDs. The absolute quantum yield was also measured for the best composition of the synthesized sample, which was observed to be 79.7% (Fig. S7†). Upon comparison, the photoluminescence quantum yield (PLQY) of the commercial phosphor Y2O3:Eu3+ has a value of 9.6% under 394 nm,68 whereas the synthesized red phosphor is 5.75 times superior than the Y2O3:Eu3+ commercial red phosphor.
Red and white LED device fabrication and quantum yield
For the practical applications of the synthesized phosphor, red and white LED devices were fabricated by combining the near UV InGaN and blue LED with the best composition of the synthesized phosphor and yellow emitting organic dye. The red LED was fabricated in the NUV LED, because the LED emission exactly matched one of the dominant excitation wavelengths (395 nm) of the NSEuM phosphor (Fig. 9a). The electroluminescence (EL) spectrum of the red LED (forward bias at 20 mA) is shown in Fig. 8a. The spectrum shows a peak at 401 nm (due to NUV LED emission), besides this the spectrum shows a sharp peak at 616 nm (corresponding to the emission of the NSEuM phosphor). The corresponding CIE and digital image of the red LED are displayed in the Fig. 8a inset. Fig. 8d shows a schematic diagram of the red LED fabrication. Prior to the fabrication of the WLED, we analyzed the excitation and emission characteristics of the individual entity. Fig. 9a illustrates the excitation and emission spectra of the NSEuM, organic yellow dye, NUV and blue LEDs respectively. It is possible to fabricate a hybrid WLED based on a blue LED, since the organic dye (yellow) and the red phosphor show good absorption in the blue region. Hybrid WLEDs were achieved using the blue LED with a yellow dye and red phosphor; because the blue LED emission precisely matches the excitation of the red phosphor (465 nm) and yellow dye. A white LED was achieved by combining a blue LED with a red phosphor. The yellow organic dye was prepared according to our previous report69 and the experimental details are given in the ESI.† For comparison, the warm WLED was fabricated by the combination of a blue LED and yellow organic dye (Fig. 8b). However, it shows a low CRI (60) and high correlated color temperature (CCT) (9333 K) values, as shown in the corresponding schematic LED fabrication diagram in Fig. 8e, and the respective CIE diagram and digital image of the fabricated WLED are shown in the Fig. 8b inset. The hybrid WLED approach was made to increase the CRI value, which was achieved via the combination of a blue LED with an appropriate amount of yellow organic dye and red phosphor. The hybrid LED shows warm white light with notably improved CIE (0.318, 0.389), the CRI (79) and CCT (6004 K) values (Fig. 8c), where a schematic diagram of the hybrid LED fabrication is exhibited in Fig. 8f. The above excellent outcomes suggest that the synthesized phosphor could be applied in WLED applications.
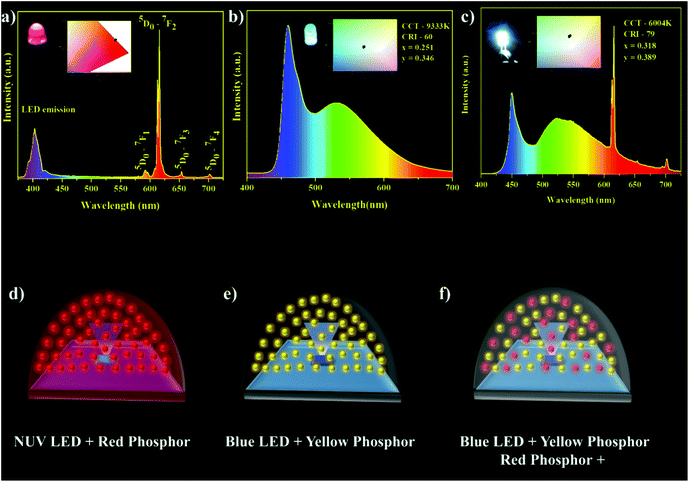 |
| Fig. 8 (a and d) Red LED EL spectrum and schematic representation of the red LED fabrication. (b and e) EL spectrum of a WLED using a blue LED with a yellow dye and a schematic diagram of the fabrication. (c and f) EL spectrum of a hybrid white LED made using a blue LED with a yellow dye and NSEuM red phosphor. | |
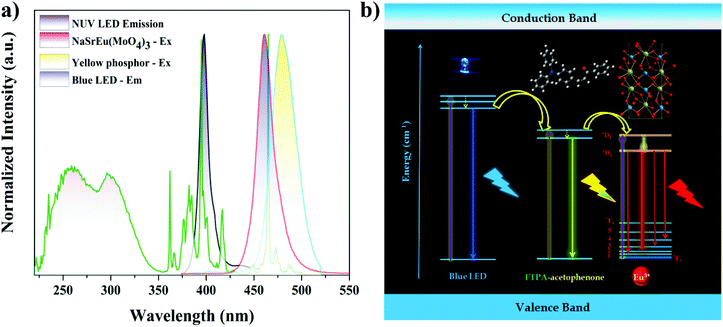 |
| Fig. 9 (a) Excitation and emission spectra of the NSEuM red phosphor, yellow dye, NUV LED and blue LEDs, respectively. (b) Schematic energy transfer mechanism of the hybrid WLED. | |
Fig. 9b shows a schematic energy transfer diagram of the hybrid WLED. Initially, the blue photons from the InGaN blue LED chip partially transfer their energy to the higher singlet state of the organic yellow dye non-radiatively. Successive vibrational cascading of the photons to the ground state from the first excited state results in yellow emission. In addition, it is also expected that the energy from the LED as well as the yellow dye excited state are transferred to the higher level of the europium ion (5D0/5D1). Now one can expect red emission from the higher europium excited levels. The hybrid WLED simultaneously emits primary colors (blue, greenish-yellow and red), which leads to white emission.
As mentioned earlier, solid state lighting is useful for plant growth. As a case study, the presently fabricated red LED emission spectrum was compared with that of phytochrome (Pr) absorption (Fig. S8†). As one can see, the fabricated red LED emission matches absorption of Pr and clearly indicates that the red LED could be suitable for use in the plant growth field. The Pr spectrum shows two absorptions at around 405 and 655 nm, which are equivalent to the NUV as well as red LED emission bands (in particular the emission at 400 nm is responsible for the NUVLED and the emissions at 550–700 nm correspond to the Eu3+ ions). Similar observations have also been documented in Bi3+ and Eu3+ co-doped Lu2GeO5 and Ca3Al4ZnO10:Bi3+,Mn4+ red phosphors. Sun et al. reported the Bi3+ and Eu3+ co-doped Lu2GeO5 red phosphor for plant growth, which shows two emission bands at around 400 and 612 nm. The blue emission band can be attributed to Bi3+ emission, whereas the red emission is related to Eu3+ ion emission.27 Similarly, the Ca3Al4ZnO10:Bi3+,Mn4+ phosphor was reported for use in plant growth.70 In general, blue and red emission play important roles in plant growth, whereas green emission is inactive since the plants themselves are green because of the color of chlorophyll. Mostly, the different types of emissive light are used during plant growth to accomplish various objectives. For instance, blue light supports vegetative leaf development. When red light is combined with blue, this enable plants to blossom. Likewise, the current red LED has blue emission as well as red emission, thus we conclude that the red LED could be a potential candidate for use in plant growth.
Sm3+ activated NaSrY(MoO4)3 – orange red emitting phosphor
The Eu3+ substituted phosphor shows potential applicability for use in WLEDs as well as plant growth (due to an overlap of the LED spectrum with Pr absorption). However, the full coverage of the Pr absorption is not convincing. Thus, Sm3+ activated or simultaneous activation of a Sm3+ and Eu3+ containing lattice (NSEuM) could be a potential phosphor for plant growth. In the present investigation, we extended the optical study of the Sm3+ activated NSYM and fabricated a LED using this system as well as mixing it with a Eu3+ containing phosphor to cover the entire region of Pr absorption. Fig. 10a shows the PL excitation and emission of the NaSrY0.95(MoO4)3:0.05Sm3+ phosphor. The excitation band was monitored under 645 nm, where the spectrum shows a broad band at 220–350 nm due to the charge transfers of O2−–Sm3+ and O2−–Mo6+. The former absorption is observed in the short UV region, whereas the latter is observed at ∼310 nm. In addition, the spectrum consists of several sharp spectral lines at 362, 377, 406, 421 and 474 nm, which correspond to the 6H5/2 → 4L15/2, 6H5/2 → 6P7/2, 6H5/2 → 4F7/2, 6H5/2 → 4P7/2, 6H5/2 → 4I11/2 electronic transitions of Sm3+, respectively. The 406 nm (6H5/2 → 4F7/2) peak is more dominant than the other peaks, and is expected to have good overlap with the near UV LED emission. The emission spectrum was monitored under 406 nm, which shows sharp intense peaks at 562, 601, 645 and 705 nm due to the electronic 4G5/2 → 6H5/2, 4G5/2 → 6H7/2, 4G5/2 → 6H9/2 and 4G5/2 → 6H11/2 transitions, respectively. Among all the emission bands, the band at 645 nm shows the greatest intensity, which could cover the Pr absorption spectrum effectively. The CIE coordinates of the corresponding emission spectrum were calculated (0.627, 0.371) and are shown alongside a digital photograph of the orange red phosphor under a 365 nm UV lamp. A concentration quenching study was executed on NSY1−xM:xSm3+ (x = 0.01, 0.03, 0.05, 0.1, 0.15, 0.2, 0.25 and 1) and the emission spectra of the phosphors are shown in Fig. 10b. It is also worth noting that all the compositions show a dominant emission at 645 nm (4G5/2 → 6H9/2, choice of interest for plant growth) and concentration quenching was observed at x = 0.05 (Fig. 10b inset). Fig. 10c shows the EL spectrum of the NSY0.95M:0.05Sm3+ phosphor integrated with a NUV-LED, which clearly shows that the Sm3+ electronic transitions are dominant over the NUV-LED emission, and digital images of the LED and CIE also shown in the inset. An orange-deep red LED was also fabricated by conjugating with a red + orange-deep red phosphor (mixing of NSEuM and NSY0.95M:0.05Sm3+) and the corresponding EL spectrum is shown in Fig. 10d, where a digital image of LED and the CIE diagram are also shown in the inset. Here, the Eu3+ and Sm3+ emission is higher in intensity than that of the LED emission and the 616 nm emission is due to the ED of the Eu3+ ion.
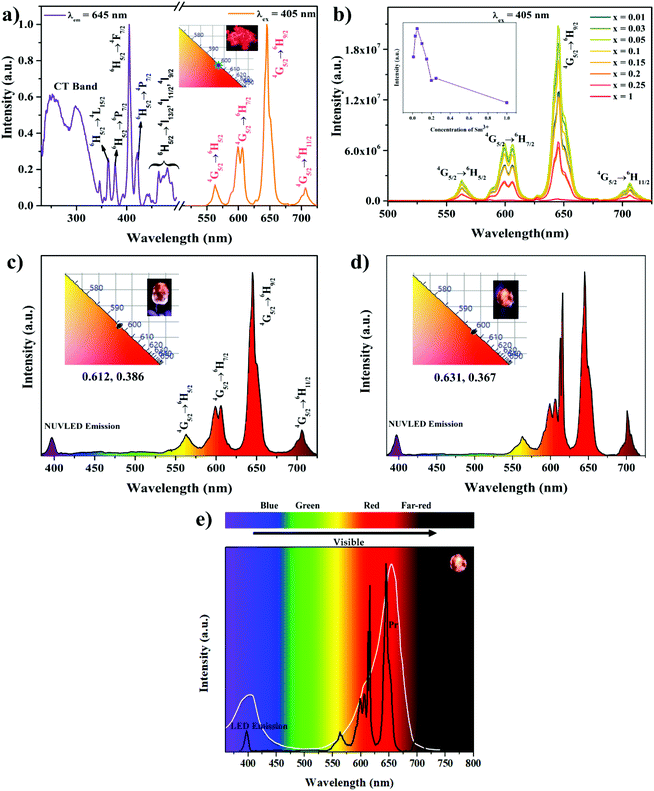 |
| Fig. 10 (a) PLE and PL spectra of the NSY0.95M:0.05Sm3+ phosphor (inset CIE and digital image of the phosphor under 365 nm). (b) PL spectra of the NSY1−xM:xSm3+ phosphors (inset plot of the intensity vs.4G5/2 → 6H9/2 transition). (c) EL spectrum of orange red LED (NUV LED + NSY0.95M:0.05Sm3+ phosphor). (d) EL spectrum of the orange-deep red LED (NUV LED + NSEuM and NSY0.95M:0.05Sm3+ phosphor). (e) EL spectrum of the orange-deep red LED (NUV LED + NSEuM and NSY0.95Sm0.05M phosphor) and absorption spectrum of Pr. | |
As mentioned earlier, the phosphor composition may find potential application in plant growth, if the spectral lines of the red LEDs can be matched to the Pr absorption spectrum. In the presently fabricated orange-deep red (NUV LED + Eu3+ and Sm3+ phosphor mixed) LED, the EL spectrum shows good overlap with the absorption of Pr, which is depicted in Fig. 10e. However, the EL spectrum of the Eu3+ and Sm3+ phosphor mixed LED emission fully covers the absorption spectrum of Pr compared with the LED made by integrating NUV with the red NSEuM phosphor.
Conclusion
A series of NSYM:Eu3+ phosphors with non-layered structures were synthesized and found to have excellent red emission at 616 nm (due to ED transition, revealing the presence of non-centrosymmetric sites). The NSEuM phosphor exhibited excellent thermal stability (retaining 64.2% at 423 K) as well as greater emission characteristics compared to those of commercial or reported red phosphors. Red and hybrid WLEDs were fabricated and the red LED exhibited intense red emission and the hybrid WLED showed efficient white light emission with reasonably good CIE (0.318, 0.389), CRI (79) and CCT (6004 K) values. The fabricated red LED was further analyzed for use in plant growth, where the EL spectrum was found to nearly cover the entire photoreceptor Pr absorption spectrum. Furthermore, to cover the full range of the Pr absorption, Sm3+ activated NSYM – orange red phosphors were synthesized. The fabricated orange-deep red LED (combination of the NUV LED with mixed Eu3+ and Sm3+ phosphors) EL spectrum precisely overlap with the absorption spectral line of Pr. These outcomes reveal that NSYM:Eu3+ phosphors could be decent contenders in the fields of tricolor based white LEDs and plant growth applications.
Conflicts of interest
There are no conflicts to declare.
Acknowledgements
The authors gratefully acknowledge Dr Dwarak Viswanathan and Prof. C. K. Jayasankar, Department of Physics, S.V. University, Tirupati, India, for extending the use of their lifetime experimental facility (facility supported by BRNS, DAE, India (MoU-DAE-BRNS Project (No. 2009/34/36/BRNS/3174)).
References
- V. Sivakumar and S. Kasturi, Eu3+-Based Orange-Red-Emitting Inorganic Color Convertors: An Overview, Phosphors, 2018, 219–324 Search PubMed.
- J. Liu, A. M. Kaczmarek and R. Van Deun, Chem. Soc. Rev., 2018, 47, 7225–7238 RSC.
- B. Rajamouli, R. Devi, A. Mohanty, V. Krishnan and V. Sivakumar, New J. Chem., 2017, 41, 9826–9839 RSC.
- B. Rajamouli, P. Sood, S. Giri, V. Krishnan and V. Sivakumar, Eur. J. Inorg. Chem., 2016, 24, 3900–3911 CrossRef.
- V. Sivakumar and U. V. Varadaraju, J. Electrochem. Soc., 2005, 152, H168–H171 CrossRef CAS.
- V. Sivakumar and U. V. Varadaraju, J. Electrochem. Soc., 2006, 153, H54–H57 CrossRef CAS.
- V. Sivakumar and U. V. Varadaraju, Electrochem. Solid-State Lett., 2006, 9, H35–H38 CrossRef CAS.
- V. Sivakumar and U. V. Varadaraju, J. Electrochem. Soc., 2007, 154, J28–J31 CrossRef CAS.
- V. Sivakumar and U. V. Varadaraju, J. Solid State Chem., 2008, 181, 3344–3351 CrossRef CAS.
-
V. Sivakumar, PhD Thesis, Indian Institute of Technology, Madras, 2007.
- P. Dorenbos, J. Lumin., 2005, 111, 89–104 CrossRef CAS.
- M. Buijs, A. Meyerink and G. Blasse, J. Lumin., 1987, 37, 9–20 CrossRef CAS.
- R. Marikumar and V. Sivakumar, New J. Chem., 2020, 44, 5354–5365 RSC.
- G.-H. Li, N. Yang, J. Zhang, J.-Y. Si, Z.-L. Wang, G.-M. Cai and X.-J. Wang, Inorg. Chem., 2020, 59, 3894–3904 CrossRef CAS PubMed.
-
S. Kasturi, PhD thesis, National Institute of Technology, Rourkela, India, 2019.
- K. Singh and S. Vaidyanathan, ChemistrySelect, 2016, 1, 5448–5462 CrossRef CAS.
- K. Singh and S. Vaidyanathan, ChemistrySelect, 2017, 2, 5143–5156 CrossRef CAS.
- J. Li, Q. Liang, Y. Cao, J. Yan, J. Zhou, Y. Xu, L. Dolgov, Y. Meng, J. Shi and M. Wu, ACS Appl. Mater. Interfaces, 2018, 10, 41479–41486 CrossRef CAS PubMed.
- Z. Zhou, J. Zheng, R. Shi, N. Zhang, J. Chen, R. Zhang, H. Suo, E. M. Goldys and C. Guo, ACS Appl. Mater. Interfaces, 2017, 9, 6177–6185 CrossRef CAS PubMed.
- T. Han, V. Vaganov, S. Cao, Q. Li, L. Ling, X. Cheng, L. Peng, C. Zhang, A. N. Yakovlev, Y. Zhong and M. Tu, Sci. Rep., 2017, 7, 45944 CrossRef CAS PubMed.
- J. Xiang, J. Chen, N. Zhang, H. Yao and C. Guo, Dyes Pigm., 2018, 154, 257–262 CrossRef CAS.
- J. Chen, N. Zhang, C. Guo, F. Pan, X. Zhou, H. Suo, X. Zhao and E. M. Goldys, ACS Appl. Mater. Interfaces, 2016, 8, 20856–20864 CrossRef CAS PubMed.
- M. Olle and A. Viršile, Agric. Food Sci., 2013, 22, 223–234 CrossRef.
- L. Ma, D. Wang, Z. Mao, Q. Lu and Z. Yuan, Appl. Phys. Lett., 2008, 93, 144101 CrossRef.
- A. Agarwal and S. D. Gupta, Curr. Biotechnol., 2016, 5, 28–43 CrossRef CAS.
- R. C. Morrow, LED Lighting in Horticulture, HortScience, 2008, 43, 1947–1950 Search PubMed.
- L. Sun, B. Devakumar, J. Liang, S. Wang, Q. Sun and X. Huang, J. Lumin., 2019, 214, 116544 CrossRef CAS.
- M. Rajendran and S. Vaidyanathan, J. Alloys Compd., 2019, 789, 919–931 CrossRef CAS.
- C. S. Lim, A. S. Aleksandrovsky, M. S. Molokeev, A. S. Oreshonkov and V. V. Atuchin, J. Alloys Compd., 2017, 713, 156–163 CrossRef CAS.
- R. M. Hazen, L. W. Finger and J. W. E. Mariathasan, J. Phys. Chem. Solids, 1985, 46, 253–263 CrossRef CAS.
- S. B. Stevens, C. A. Morrison, T. H. Allik, A. L. Rheingold and B. S. Haggerty, Phys. Rev. B: Condens. Matter Mater. Phys., 1991, 43, 7386–7394 CrossRef CAS PubMed.
- R. Shannon, Acta Crystallogr., Sect. A: Cryst. Phys., Diffr., Theor. Gen. Crystallogr., 1976, 32, 751–767 CrossRef.
- C. S. Lim, V. V. Atuchin, A. S. Aleksandrovsky and M. S. Molokeev, Mater. Lett., 2016, 181, 38–41 CrossRef CAS.
- D. Chen, Y. Yu, F. Huang, P. Huang, A. Yang and Y. Wang, J. Am. Chem. Soc., 2010, 132, 9976–9978 CrossRef CAS PubMed.
- X. Feng, D. C. Sayle, Z. L. Wang, M. S. Paras, B. Santora, A. C. Sutorik, T. X. T. Sayle, Y. Yang and Y. Ding, Science, 2006, 312, 1504–1508 CrossRef CAS PubMed.
- X. Wang, Y. Wang, J. Yu, Y. Bu and X. Yan, Opt. Express., 2018, 26, 21950–21959 CrossRef CAS PubMed.
- N. M. Kozhevnikova and O. A. Kopylova, Russ. J. Inorg. Chem., 2011, 56, 935–938 CrossRef CAS.
- Y. Liu, S. Ma, H. Zuo, J. Li, X. Shi and M. Zhao, Ceram. Int., 2016, 42, 16499–16504 CrossRef CAS.
- F. Lei, B. Yan, H. H. Chen and J. T. Zhao, Inorg. Chem., 2009, 48, 7576–7584 CrossRef CAS PubMed.
-
R. Reisfeld and C. K. Jørgensen, Lasers and Excited States of RareEarth[M], Springer, Berlin, 1977, p. 45 Search PubMed.
- X. Gao, Y. Wang, D. Wang and B. Liu, J. Lumin., 2009, 129, 840–843 CrossRef CAS.
- M.-Z. Su and X.-P. Sun, Mater. Res. Bull., 1987, 22, 89–94 CrossRef CAS.
-
B. Shionoya and M. Yen, Phosphor Handbook, CRC Press, Boca Raton, FL, 1999, pp. 88–179 Search PubMed.
- B.-S. Tsai, Y.-H. Chang and Y.-C. Chen, Electrochem. Solid-State Lett., 2005, 8, H55–H57 CrossRef CAS.
- C.-H. Liang, Y.-C. Chang and Y.-S. Chang, J. Electrochem. Soc., 2009, 156, J303–J307 CrossRef CAS.
- N. Rakov, F. E. Ramos, G. Hirata and M. Xiao, Appl. Phys. Lett., 2003, 83, 272–274 CrossRef CAS.
- G. S. R. Raju, E. Pavitra, S. K. Hussain, D. Balaji and J. S. Yu, J. Mater. Chem. C, 2016, 4, 1039–1050 RSC.
- D. Boyer, G. Bertrand and R. Mahiou, J. Lumin., 2003, 104, 229–237 CrossRef CAS.
- G. Blasse, J. Solid State Chem., 1986, 62, 207–211 CrossRef CAS.
- Y. Tian, Y. Liu, R. Hua, L. Na and B. Chen, Mater. Res. Bull., 2012, 47, 59–62 CrossRef CAS.
- G. S. Ofelt, J. Chem. Phys., 1962, 37, 511–520 CrossRef CAS.
- S. H. Huang and L. R. Lou, Chin. J. Lumin., 1990, 11, 1–7 Search PubMed.
- W. Luo, D. Ling, S. Bao, H. Xiong, R. Zhang, B. Li and H. Wu, Luminescence, 2018, 33, 312–317 CrossRef CAS PubMed.
- Maheshwary, B. P. Singh, J. Singh and R. A. Singh, RSC Adv., 2014, 4, 32605–32621 CAS.
- B. R. Judd, Phys. Rev., 1962, 127, 750–761 CrossRef CAS.
- K. Singh and S. Vaidyanathan, Mater. Chem. Front., 2017, 1, 550–561 RSC.
- K. Singh, R. Boddula and S. Vaidyanathan, Inorg. Chem., 2017, 56, 9376–9390 CrossRef CAS PubMed.
- Y.-C. Chang, C.-H. Liang, S.-A. Yan and Y.-S. Chang, J. Phys. Chem. C, 2010, 114, 3645–3652 CrossRef CAS.
- L. Wang, W. Guo, Y. Tian, P. Huang, Q. Shi and C. Cui, Ceram. Int., 2016, 42, 13648–13653 CrossRef CAS.
- X. Liu, B. Shen, B. Chen, Y. Zhang and J. Hu, Opt. Mater., 2019, 96, 109353 CrossRef CAS.
- Y. N. Li, D. Zhao, R. J. Zhang, F. F. Li, L. Y. Shi, Q. X. Yao, X. Y. Han and X. Q. Cui, Dalton Trans., 2019, 48, 13780–13788 RSC.
- J. Hu, X. Gong, J. Huang, Y. Chen, Y. Lin, Z. Luo and Y. Huang, Opt. Mater. Express|, 2016, 6, 181–190 CrossRef CAS.
- C. H. Liang, Y. C. Chang and Y. S. Chang, Appl. Phys. Lett., 2008, 93, 211902 CrossRef.
- B. Wei, Z. Liu, C. Xie, S. Yang, W. Tang, A. Gu, W.-T. Wong and K.-L. Wong, J. Mater. Chem. C, 2015, 3, 12322–12327 RSC.
- P. Du, L. K. Bharat and J. S. Yu, J. Alloys Compd., 2015, 633, 37–41 CrossRef CAS.
- M. Song, L. Wang, Y. Feng, H. Wang, X. Wang and D. Li, Opt. Mater., 2018, 84, 284–291 CrossRef CAS.
- P. Du and J. S. Yu, RSC Adv., 2015, 5, 60121–60127 RSC.
- S. Long, J. Hou, G. Zhang, F. Huang and Y. Zeng, Ceram. Int., 2013, 39, 6013–6017 CrossRef CAS.
- A. B. Kajjam, S. Giri and S. Vaidyanathan, Mater. Chem. Front., 2017, 1, 512–520 RSC.
- Z. Zhou, Y. Zhong, M. Xia, N. Zhou, B. Lei, J. Wang and F. Wu, J. Mater. Chem. C, 2018, 6, 8914–8922 RSC.
Footnote |
† Electronic supplementary information (ESI) available. See DOI: 10.1039/d0dt01517b |
|
This journal is © The Royal Society of Chemistry 2020 |