DOI:
10.1039/C9DT04851K
(Frontier)
Dalton Trans., 2020,
49, 2397-2402
Lanthanide conjugates as versatile instruments for therapy and diagnostics
Received
22nd December 2019
, Accepted 10th January 2020
First published on 14th January 2020
Abstract
Lanthanides have demonstrated outstanding properties in many fields of research including biology and medicinal chemistry. Their unique luminescence and magnetic properties make them the metals of choice for next generation theranostics that efficiently combine the two central pillars of medicine – diagnostics and therapy. Attached to targeting units, lanthanide complexes pave the way for real-time imaging of drug uptake and distribution as well as specific regulation of subcellular processes with few side effects. This enables individualized treatment options for severe diseases characterized by altered cell expression. The highly diverse results achieved as well as insights into the challenges that research in this area has to face in the upcoming years will be summarized in the present review.
Introduction
Modern theranostics require highly selective, multifunctional materials that are easily detectable in the surrounding tissue and capable of reliably transporting diverse cargoes. Due to their unique properties, lanthanides are promising imaging components of next generation diagnostics paving the way for individualized therapeutics with few side effects. Their long-lived luminescence in the visible to near-infrared range, large Stokes and anti-Stokes shifts, outstanding photo-stability and narrow emission regardless of the surrounding ligands make lanthanides the metals of choice for in vivo imaging and real-time tracking of drug uptake and distribution.1
Lanthanide luminescence results from Laporte-forbidden f–f-transitions that result in long-lived excited states, but also need organic receptors that transfer energy towards the metal centre.2–5 This so-called antenna effect is crucial as the rare earths are characterized by low absorption coefficients. Due to versatile luminescence properties along the lanthanide row,6,7 a broad range of emission wavelengths is covered by choosing an appropriate representative.8
Not only luminescence, but also magnetic resonance imaging (MRI) is related to lanthanides.9,10 The most prominent MRI contrast agents possessing clinical relevance are the paramagnetic gadolinium(III) ion complexes. Unlimited penetration depth and the absence of ionizing radiation are the unique selling points of MRI-based in vivo imaging agents.9,11
As strong emitters that are easily distinguishable from background noise, rare earths fulfil various purposes in biological and medicinal research. Besides their manifold diagnostic applications, the lanthanides can function as therapeutics in radiation or photodynamic therapy. Linked to targeting motifs that transport the rare earths into desired tissues, the lanthanides are effective theranostics as consistently demonstrated by their fascinating research outcomes.
Lanthanide conjugates in diagnostics and therapy
Chemotherapeutics harm the entire organism due to severe side effects since the reliable targeting of diseased cells is still challenging. Several diseases are characterized by degenerated cells possessing altered expression patterns of distinct surface proteins. Conjugation to units that target these proteins paves the way for cell-specific targeting which can be monitored by means of lanthanides. This can be achieved, e.g., by using peptidomimetics such as functionalized peptoids.12–16
To avoid the cytotoxicity of free metal ions, which is mainly caused by binding to endogenous metalloproteins and subsequent structure disruption, the metals are tightly complexed with multidentate ligands.17–20 The most prominent chelators are the macrocyclic 1,4,7,10-tetraazacyclododecane-1,4,7,10-tetraacetate (DOTA) and its analogs (Fig. 1).21,22
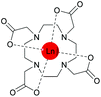 |
| Fig. 1 The macrocyclic DOTA as a common leading structure for tight lanthanide complexation. | |
Recently, the high specificity in vivo targeting of tumour cells using a biotin-conjugated gadolinium(III)–DOTA (Gd(III)–DOTA) complex has been reported (Fig. 2).23 Gd(III)–DOTA and its analogs are commonly known to be efficient contrast agents in clinical MRI and find use in many theranostic applications.24–30 The described biotin-labelled Gd(III)–DOTA conjugate successfully delivered a highly potent cytostatic, camptothecin, into cancer cells and allowed for real-time imaging of its distribution and cellular uptake in vivo. Administration of the pure anti-tumour drug resulted in severe side effects due to the induced apoptosis of healthy cells. As the prodrug is linked to the transporter-conjugate, the entire organism is not harmed as internalization is realized only in biotin-specific receptor overexpressing cancer cells. Once it reaches the target, the intracellular environment triggers the reductive cleavage of the incorporated disulfide bond and the subsequent release of the cytostatic agent, revealing its therapeutic capability.
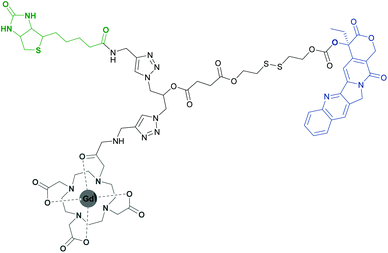 |
| Fig. 2 A biotin-conjugated (green) Gd(III)–DOTA complex (grey) that efficiently delivered camptothecin (blue) into tumour cells.23 | |
Lanthanides do not only serve as imaging agents for drug tracking or diagnostic purposes,31 but also function as therapeutics on their own. Lutetium-177 is a medium-energy β-emitter which permits monitoring via scintigraphy and concurrent tissue irradiation. Transporter-conjugates of Lu-177 complexes enable tailored radiotherapy with slight systemic toxicity. In particular, peptides and related foldamers such as peptoids that target certain receptors are linked to the radionuclide, initiating their accumulation in malignant tissues.32 The method has been shown to be feasible for inoperable neuroendocrine tumours that are efficiently targeted by somatostatin and gastrin analogues.33–36 Clinical trials have further revealed the promising effect of Lu-177 linked to agonists of the prostate-specific membrane antigen. Targeting this glycoprotein has shown to enable specific radionuclide therapy of metastasized castration-resistant prostate cancer.37–40
Lanthanide-doped nanoparticles enhance specificity
Although lanthanide conjugates work well in terms of specificity, their pharmacokinetic properties can be further improved. Encapsulation into nanoparticles permits the tuning of solubility, lowers systemic toxicity and promotes transmembrane crossing.41 Surface decoration with targeting units heightens the valency of interacting moieties and therefore the affinity of the entire particle.42 Due to these advantages, a broad field of research deals with different kinds of lanthanide-doped nanoparticles that can be easily functionalized with distinct targeting motifs.43 However, their uptake is restricted to certain cell types, which allows for tailored visualization and therapy with few side effects.44
A common target for multifunctional nanophosphors is the folate receptor, overexpressed in several human cancer cells such as colon, lung, breast and ovarian.45,46 By masking nanoparticles with folic acid (Fig. 3), receptor-mediated uptake is triggered, which exclusively transports the particles into cancer cells while leaving the healthy ones untouched. This specificity paves the way for targeted treatment of several diseases based on cellular abnormalities. The principle mentioned is applied to a variety of nanophosphors like nanocrystals47,48 or silica nanoparticles.49–51
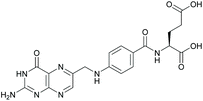 |
| Fig. 3 Folic acid as a common targeting motif for several human cancer types. | |
Among these nanocomposites, particles with unique luminescence properties exist.52 Persistent luminescence nanoparticles (PLNPs) are characterized by a luminescence decay that lasts at least several seconds rendering background-free detection in biological systems with a high signal-to-noise ratio.53 The afterglow stems from the storage of excitation energy by charged defects and subsequent slow release resulting in long-lived luminescence that can even last for days.41 Conjugation to folic acid permits tumour visualization clearly distinguishable from the autofluorescence of surrounding tissue.54,55
Another extensively investigated class of nanophosphors are lanthanide-doped up-conversion nanoparticles (UCNPs). By sequential absorption of photons or energy transfer, near-infrared radiation is converted to visible light of higher energy, which allows for minimized autofluorescence, low phototoxicity and sufficient tissue penetration while maintaining suitable luminescence. The properties of UCNPs as well as their limitations from a physicochemical point of view have been comprehensively discussed elsewhere.8 Folic acid conjugation triggers sole UCNP uptake in folate receptor overexpressing cancer cell lines in vitro and in vivo.56–61
Besides folic acid, conjugation of nanophosphors to various targeting units has been reported. Antibodies, functionalisable glycoproteins, are known for their outstandingly high affinity binding to certain targets ranging from metabolites to receptors.62 Several working groups have benefited from their function when masking nanoparticles.63–67 Niu et al. visualized the induced apoptosis of CD33 receptor overexpressing myeloid leukemia cells caused by a humanized monoclonal antibody conjugated to a lanthanide oxyfluoride nanoparticle. Apoptotic events, induced by additionally conjugated peptide antagonists of negative regulators of the tumour suppressor protein p53, were triggered only in malignant cells, proving the antibody-derived affinity.68 Antibody labelled nanophosphors were moreover tested in vivo. Huang et al. reported the labelling of pancreatic cancer cell xenografts in mice using an antibody against the cell surface proteoglycan glypican-1. The resulting nanoprobe showed efficient targeting without toxic side effects, making it a promising candidate for clinical applications.69
Conjugation of specific peptides and other small biomolecules to nanocomposites has been extensively studied.70–74 The cytokine interleukin-13 was chosen as a reliable sensor for glioblastoma multiforme cells in xenografted mice. Lanthanide-doped metallofullerene-based interleukin-13 nanoparticles paved the way for specific theranostic treatment of this common malignant brain tumour which is characterized by a median survival rate of merely one year.42,75
In the course of photodynamic therapy (PDT), lanthanides serve as photosensitizers, which also makes them applicable as composites for imaging purposes. Due to the heavy atom effect, intersystem crossing from the singlet to triplet state is facilitated after excitation of rare earths.76 In the triplet state, the reaction with molecular oxygen is enabled generating a highly reactive singlet oxygen species that causes severe disruption of the cellular environment, which efficiently leads to programmed cell-death. A lot of research deals with lanthanide-doped UCNPs as they are excitable in the deeper-tissue penetrating NIR region.77–79 Recently, PDT-related apoptosis of certain cancer cell lines after excitation of UCNPs with a low-power 980 nm laser has been reported.80 Nevertheless, quantum yields of the UCNPs as well as their targeting properties still have to be improved. One approach towards the latter is the design of smart nanoprobes that solely exhibit PDT activity in cancerous tissue. Fan et al. stated that the high potential of this concept lies in the luminescence and singlet oxygen generation by cerium oxide based nanoparticles, so-called nanoceria, being successfully turned on after matrix metalloproteinase-2 catalysed cleavage of a certain peptide linker. The enzyme itself serves as a biomarker for cancer diagnosis.81
Nanoceria derivatives do not only have applicability in PDT, but also in the treatment of diseases that are related to damage caused by singlet oxygen. Cerium nanoparticles are characterised by charge deficiencies due to facile interconversion between a trivalent and a tetravalent oxidation state and subsequent surface oxygen vacancies. Redox-cycling allows for scavenging reactive oxygen species which makes them therapeutic candidates against oxidative stress.82 Specific mitochondrial localization of triphenyl-phosphonium-modified nanoceria and significant suppression of Alzheimer's disease related neuronal death in transgenic mice were reported by Kwon et al., confirming the reliability of this concept.83
Due to their manifold properties, lanthanide-doped nanoparticles come along with wide applicability in biology and medicine. But although they are regarded as nontoxic, one has to take into account that their metabolization is still not understood in detail. Further research is required to clarify their metabolic toxicity, avert their accumulation in liver and the reticuloendothelial system and further improve their pharmacokinetics.
Conclusions
Lanthanide conjugates with specific targeting ability have been proved to show applicability as next generation theranostics. Their unique luminescence properties allow for background-free precise real-time imaging of drug uptake and distribution. Their outstanding suitability for MRI indicates their usefulness in deep tissue in vivo imaging. The conjugation of lanthanide complexes to targeting units as well as to medicinal compounds paves the way for simultaneous diagnostics and therapy. Incorporation of rare earths into nanoparticles can enhance their pharmacokinetics, heighten multiplicity and establish extraordinary properties. In certain types of therapy, lanthanides can induce programmed cell death or neutralize reactive oxygen species, offering innovative treatment options for severe diseases.
Their comprehensive properties make the rare earths the metals of choice for theranostic applications, sometimes inspired by systems used in materials sciences.84–89 But despite their manifold advantages, there are still challenges that have to be overcome. Improvement of physicochemical properties like quantum yield and efficiency has to be considered, while irradiation wavelengths and laser power need to be optimized. This assists in lowering undesired side effects such as tissue overheating and systemic toxicity caused by high administered concentrations. The intrinsic toxicity of metabolized lanthanide conjugates is still not fully clarified and demands further efforts. Research on the rare earths holds huge promise in biomedical applications and will continually reveal fascinating results in upcoming years.
Conflicts of interest
There are no conflicts to declare.
Acknowledgements
We acknowledge the support from the KIT and the DFG (GRK 2039).
References
- J.-C. G. Bünzli, Trends Chem., 2019, 1130, 97–105 Search PubMed.
- Y. Liu, D. Tu, H. Zhu and X. Chen, Chem. Soc. Rev., 2013, 42, 6924–6958 RSC.
- L. Ancel, A. Niedźwiecka, C. Lebrun, C. Gateau and P. Delangle, C. R. Chim., 2013, 16, 515–523 CrossRef.
- S. Lahdenpera, Q. Wang, J. Vainio and T. Soukka, Analyst, 2017, 142, 2411–2418 RSC.
- J.-C. G. Bünzli, Chem. Rev., 2010, 110, 2729–2755 CrossRef PubMed.
- D. T. Thielemann, A. T. Wagner, Y. Lan, P. Ona-Burgos, I. Fernandez, E. S. Roesch, D. K. Kölmel, A. K. Powell, S. Bräse and P. W. Roesky, Chem. – Eur. J., 2015, 21, 2813–2820 CrossRef PubMed.
- D. T. Thielemann, A. T. Wagner, E. Roesch, D. K. Kölmel, J. G. Heck, B. Rudat, M. Neumaier, C. Feldmann, U. Schepers, S. Bräse and P. W. Roesky, J. Am. Chem. Soc., 2013, 135, 7454–7457 CrossRef PubMed.
- M. V. DaCosta, S. Doughan, Y. Han and U. J. Krull, Anal. Chim. Acta, 2014, 832, 1–33 CrossRef PubMed.
- M. C. Heffern, L. M. Matosziuk and T. J. Meade, Chem. Rev., 2014, 114, 4496–4539 CrossRef PubMed.
- S. Aime, D. D. Castelli, S. G. Crich, E. Gianolio and E. Terreno, Acc. Chem. Res., 2009, 42, 822–831 CrossRef PubMed.
- S. Lacerda and É. Tóth, ChemMedChem, 2017, 12, 883–894 CrossRef PubMed.
- A. B. Braun, I. Wehl, D. K. Kölmel, U. Schepers and S. Bräse, Chem. – Eur. J., 2019, 25, 7998–8002 CrossRef PubMed.
- D. K. Kölmel, D. Fuerniss, S. Susanto, A. Lauer, C. Grabher, S. Brase and U. Schepers, Pharmaceuticals, 2012, 5, 1265–1281 CrossRef PubMed.
- D. K. Kölmel, A. Hoerner, F. Roenicke, M. Nieger, U. Schepers and S. Bräse, Eur. J. Med. Chem., 2014, 79, 231–243 CrossRef PubMed.
- B. Rudat, E. Birtalan, I. Thome, D. K. Kölmel, V. L. Horhoiu, M. D. Wissert, U. Lemmer, H.-J. Eisler, T. S. Balaban and S. Brase, J. Phys. Chem. B, 2010, 114, 13473–13480 CrossRef PubMed.
- B. Rudat, E. Birtalan, S. B. L. Vollrath, D. Fritz, D. K. Kölmel, M. Nieger, U. Schepers, K. Muellen, H.-J. Eisler, U. Lemmer and S. Brase, Eur. J. Med. Chem., 2011, 46, 4457–4465 CrossRef PubMed.
- L. L. Brayshaw, R. C. Smith, M. Badaoui, J. A. Irving and S. R. Price, Metallomics, 2019, 11(5), 914–924 RSC.
- L. Wang, J. He, A. Xia, M. Cheng, Q. Yang, C. Du, H. Wei, X. Huang and Q. Zhou, Chemosphere, 2017, 181, 690–698 CrossRef PubMed.
- S. C. Edington, A. Gonzalez, T. R. Middendorf, D. B. Halling, R. W. Aldrich and C. R. Baiz, Proc. Natl. Acad. Sci. U. S. A., 2018, 115, 3126–3134 CrossRef CAS PubMed.
- A. Palasz, Y. Segovia, R. Skowronek and J. J. Worthington, Synapse, 2019, 73, 1–14 Search PubMed.
- Z. Hashami, A. F. Martins, A. M. Funk, V. C. Jordan, S. Petoud, S. V. Eliseeva and Z. Kovacs, Eur. J. Inorg. Chem., 2017, 2017, 4965–4968 CrossRef PubMed.
- S. Prochazkova, V. Kubicek, J. Kotek, A. Vagner, J. Notni and P. Hermann, Dalton Trans., 2018, 47, 13006–13015 RSC.
- Z. Yang, H. Lin, J. Huang, A. Li, C. Sun, J. Richmond and J. Gao, Chem. Commun., 2019, 55, 4546–4549 RSC.
- D. Xu, S.-T. Lu, Y.-S. Li, A. Baidya, H. Mei, Y. He and B. Wu, Drug Des., Dev. Ther., 2018, 12, 3301–3309 CrossRef.
- A. F. Martins, J. F. Morfin, A. Kubickova, V. Kubicek, F. Buron, F. Suzenet, M. Salerno, A. N. Lazar, C. Duyckaerts, N. Arlicot, D. Guilloteau, C. F. Geraldes and E. Toth, ACS Med. Chem. Lett., 2013, 4, 436–440 CrossRef.
- A. F. Martins, D. M. Dias, J. F. Morfin, S. Lacerda, D. V. Laurents, E. Toth and C. F. Geraldes, Chemistry, 2015, 21, 5413–5422 CrossRef.
- A. F. Martins, A. C. Oliveira, J. F. Morfin, D. V. Laurents, E. Toth and C. F. Geraldes, J. Biol. Inorg. Chem., 2016, 21, 83–99 CrossRef.
- A. Sturzu, U. Klose, S. Sheikh, H. Echner, H. Kalbacher, M. Deeg, T. Nägele, C. Schwentner, U. Ernemann and S. Heckl, Eur. J. Pharm. Sci., 2014, 52, 69–76 CrossRef.
- J.-A. Park, Y. J. Lee, I. O. Ko, T.-J. Kim, Y. Chang, S. M. Lim, K. M. Kim and J. Y. Kim, Biochem. Biophys. Res. Commun., 2014, 455, 246–250 CrossRef.
- I. Zigelboim, A. Weissberg and Y. Cohen, J. Org. Chem., 2013, 78, 7001–7012 CrossRef CAS.
- D. K. Kölmel, B. Rudat, U. Schepers and S. Bräse, Eur. J. Org. Chem., 2013, 2761–2765 CrossRef.
- M. F. Tweedle, Acc. Chem. Res., 2009, 42, 958–968 CrossRef CAS.
- B. Kam, J. Teunissen, E. P. Krenning, W. W. de Herder, S. Khan, E. Van Vliet and D. J. Kwekkeboom, Eur. J. Nucl. Med. Mol. Imaging, 2012, 39, 103–112 CrossRef CAS.
- S. F. A. Rizvi, S. A. R. Naqvi, S. Roohi, T. A. Sherazi and R. Rasheed, Mol. Biol. Rep., 2018, 45, 1759–1767 CrossRef CAS.
- P. M. Kasi, A. Sharma and M. K. Jain, Case Rep. Oncol., 2019, 12, 98–103 CrossRef CAS.
- L. Vija, L. Dierickx and F. Courbon, Ann. Endocrinol., 2019, 80, 166–171 CrossRef.
- A. Farolfi, W. Fendler, A. Iravani, U. Haberkorn, R. Hicks, K. Herrmann, J. Walz and S. Fanti, Eur. Urol. Oncol., 2019, 2, 152–162 CrossRef.
- L. Emmett, K. Willowson, J. Violet, J. Shin, A. Blanksby and J. Lee, J. Med. Radiat. Sci., 2017, 64, 52–60 CrossRef.
- J. Zhang, H. R. Kulkarni, A. Singh and R. P. Baum, Clin. Nucl. Med., 2019, 45, 48–50 CrossRef.
- F. Edler von Eyben, A. Singh, J. Zhang, K. Nipsch, D. Meyrick, N. Lenzo, K. Kairemo, T. Joensuu, I. Virgolini, C. Soydal, H. R. Kulkarni and R. P. Baum, Oncotarget, 2019, 10, 2451–2461 Search PubMed.
- B. Sanii, T. K. Haxton, G. K. Olivier, A. Cho, B. Barton, C. Proulx, S. Whitelam and R. N. Zuckermann, ACS Nano, 2014, 8, 11674–11684 CrossRef CAS.
- T. Li, S. Murphy, B. Kiselev, K. S. Bakshi, J. Zhang, A. Eltahir, Y. Zhang, Y. Chen, J. Zhu, R. M. Davis, L. A. Madsen, J. R. Morris, D. R. Karolyi, S. M. LaConte, Z. Sheng and H. C. Dorn, J. Am. Chem. Soc., 2015, 137, 7881–7888 CrossRef CAS.
- H. Dong, S.-R. Du, X.-Y. Zheng, G.-M. Lyu, L.-D. Sun, L.-D. Li, P.-Z. Zhang, C. Zhang and C.-H. Yan, Chem. Rev., 2015, 115, 10725–10815 CrossRef CAS.
- D. Peer, J. M. Karp, S. Hong, O. C. Farokhzad, R. Margalit and R. Langer, Nat. Nanotechnol., 2007, 2, 751 CrossRef CAS.
- Y. G. Assaraf, C. P. Leamon and J. A. Reddy, Drug Resist. Updates, 2014, 17, 89–95 CrossRef.
- A. Cheung, H. J. Bax, D. H. Josephs, K. M. Ilieva, G. Pellizzari, J. Opzoomer, J. Bloomfield, M. Fittall, A. Grigoriadis, M. Figini, S. Canevari, J. F. Spicer, A. N. Tutt and S. N. Karagiannis, Oncotarget, 2016, 7, 52553–52574 Search PubMed.
- S. Setua, D. Menon, A. Asok, S. Nair and M. Koyakutty, Biomaterials, 2010, 31, 714–729 CrossRef CAS.
- R. Kumar, M. Nyk, T. Y. Ohulchanskyy, C. A. Flask and P. N. Prasad, Adv. Funct. Mater., 2009, 19, 853–859 CrossRef CAS.
- J. Ma, P. Huang, M. He, L. Pan, Z. Zhou, L. Feng, G. Gao and D. Cui, J. Phys. Chem. B, 2012, 116, 14062–14070 CrossRef.
- M. H. Chan and H. M. Lin, Biomaterials, 2015, 46, 149–158 CrossRef CAS.
- S. S. Syamchand, R. S. Aparna and S. George, J. Fluoresc., 2017, 27, 1897–1908 CrossRef CAS.
- V. Y. Khudoleeva, V. V. Utochnikova, A. S. Kalyakina, I. M. Deygen, A. A. Shiryaev, L. Marciniak, V. A. Lebedev, I. V. Roslyakov, A. V. Garshev, L. S. Lepnev, U. Schepers, S. Bräse and N. P. Kuzmina, Dyes Pigm., 2017, 143, 348–355 CrossRef CAS.
- X. Sun, J. Shi, X. Fu, Y. Yang and H. Zhang, Sci. Rep., 2018, 8, 10595 CrossRef.
- J. Shi, X. Sun, J. Li, H. Man, J. Shen, Y. Yu and H. Zhang, Biomaterials, 2015, 37, 260–270 CrossRef CAS.
- J. L. Li, J. P. Shi, C. C. Wang, P. H. Li, Z. F. Yu and H. W. Zhang, Nanoscale, 2017, 9, 8631–8638 RSC.
- L.-Q. Xiong, Z.-G. Chen, M.-X. Yu, F.-Y. Li, C. Liu and C.-H. Huang, Biomaterials, 2009, 30, 5592–5600 CrossRef CAS PubMed.
- D. K. Chatterjee, A. J. Rufaihah and Y. Zhang, Biomaterials, 2008, 29, 937–943 CrossRef CAS.
- C. Wang, L. Cheng and Z. Liu, Biomaterials, 2011, 32, 1110–1120 CrossRef CAS.
- H. Hu, L. Xiong, J. Zhou, F. Li, T. Cao and C. Huang, Chem. – Eur. J., 2009, 15, 3577–3584 CrossRef CAS.
- C. Liu, Y. Qi, R. Qiao, Y. Hou, K. Chan, Z. Li, J. Huang, L. Jing, J. Du and M. Gao, Nanoscale, 2016, 8, 12579–12587 RSC.
- L. Pan, M. He, J. Ma, W. Tang, G. Gao, R. He, H. Su and D. Cui, Theranostics, 2013, 3, 210–222 CrossRef CAS.
- G. Köhler and C. Milstein, Nature, 1975, 256, 495–497 CrossRef.
- S. Sinha, W. Y. Tong, N. H. Williamson, S. J. P. McInnes, S. Puttick, A. Cifuentes-Rius, R. Bhardwaj, S. E. Plush and N. H. Voelcker, ACS Appl. Mater. Interfaces, 2017, 9, 42601–42611 CrossRef CAS.
- J. Pansieri, M. Plissonneau, N. Stransky-Heilkron, M. Dumoulin, L. Heinrich-Balard, P. Rivory, J. F. Morfin, E. Toth, M. J. Saraiva, E. Allemann, O. Tillement, V. Forge, F. Lux and C. Marquette, Nanomedicine, 2017, 12, 1675–1687 CrossRef CAS.
- R. Qiao, C. Liu, M. Liu, H. Hu, C. Liu, Y. Hou, K. Wu, Y. Lin, J. Liang and M. Gao, ACS Nano, 2015, 9, 2120–2129 CrossRef CAS.
- C. Liu, Z. Gao, J. Zeng, Y. Hou, F. Fang, Y. Li, R. Qiao, L. Shen, H. Lei, W. Yang and M. Gao, ACS Nano, 2013, 7, 7227–7240 CrossRef CAS.
- Y. Hou, R. Qiao, F. Fang, X. Wang, C. Dong, K. Liu, C. Liu, Z. Liu, H. Lei, F. Wang and M. Gao, ACS Nano, 2013, 7, 330–338 CrossRef CAS.
- F. Niu, J. Yan, B. Ma, S. Li, Y. Shao, P. He, W. Zhang, W. He, P. X. Ma and W. Lu, Biomaterials, 2018, 167, 132–142 CrossRef CAS PubMed.
- X. Huang, C. Fan, H. Zhu, W. Le, S. Cui, X. Chen, W. Li, F. Zhang, Y. Huang, D. Sh, Z. Cui, C. Shao and B. Chen, Int. J. Nanomed., 2018, 13, 2585–2599 CrossRef CAS.
- M. Plissonneau, J. Pansieri, L. Heinrich-Balard, J.-F. Morfin, N. Stransky-Heilkron, P. Rivory, P. Mowat, M. Dumoulin, R. Cohen, É. Allémann, É. Tóth, M. J. Saraiva, C. Louis, O. Tillement, V. Forge, F. Lux and C. Marquette, J. Nanobiotechnol., 2016, 14, 60 CrossRef.
- H. Li, B. D. Gray, I. Corbin, C. Lebherz, H. Choi, S. Lund-Katz, J. M. Wilson, J. D. Glickson and R. Zhou, Acad. Radiol., 2004, 11, 1251–1259 CrossRef.
- J. Xu, L. Xu, C. Wang, R. Yang, Q. Zhuang, X. Han, Z. Dong, W. Zhu, R. Peng and Z. Liu, ACS Nano, 2017, 11, 4463–4474 CrossRef CAS.
- H. Qiao, Z. Cui, S. Yang, D. Ji, Y. Wang, Y. Yang, X. Han, Q. Fan, A. Qin, T. Wang, X. P. He, W. Bu and T. Tang, ACS Nano, 2017, 11, 7259–7273 CrossRef CAS.
- J. Yan, W. He, S. Yan, F. Niu, T. Liu, B. Ma, Y. Shao, Y. Yan, G. Yang and W. Lu, ACS Nano, 2018, 12, 2017–2026 CrossRef CAS.
- H. L. Fillmore, M. D. Shultz, S. C. Henderson, P. Cooper, W. C. Broaddus, Z. J. Chen, C.-Y. Shu, J. Zhang, J. Ge and H. C. Dorn, Nanomedicine, 2011, 6, 449–458 CrossRef CAS.
- X. Sun, J. Sun, B. Dong, G. Huang, L. Zhang, W. Zhou, J. Lv, X. Zhang, M. Liu, L. Xu, X. Bai, W. Xu, Y. Yang, X. Song and H. Song, Biomaterials, 2019, 201, 42–52 CrossRef CAS.
- M. R. Hamblin, Dalton Trans., 2018, 47, 8571–8580 RSC.
- X. Zhang, Z. Guo, X. Zhang, L. Gong, X. Dong, Y. Fu, Q. Wang and Z. Gu, Sci. Rep., 2019, 9, 5212 CrossRef.
- Y. Feng, Y. Wu, J. Zuo, L. Tu, I. Que, Y. Chang, L. J. Cruz, A. Chan and H. Zhang, Biomaterials, 2019, 201, 33–41 CrossRef CAS.
- G. Li, W. Wang, S. Song, Y. Sun, J. Liu, K. Chen, J. Liu and W. Wang, J. Biomed. Nanotechnol., 2019, 15, 462–476 CrossRef CAS.
- Y. Fan, P. Li, B. Hu, T. Liu, Z. Huang, C. Shan, J. Cao, B. Cheng, W. Liu and Y. Tang, Inorg. Chem., 2019, 58, 7295–7302 CrossRef CAS.
- F. Charbgoo, M. B. Ahmad and M. Darroudi, Int. J. Nanomed., 2017, 12, 1401 CrossRef CAS.
- H. J. Kwon, M.-Y. Cha, D. Kim, D. K. Kim, M. Soh, K. Shin, T. Hyeon and I. Mook-Jung, ACS Nano, 2016, 10, 2860–2870 CrossRef CAS.
- A. Kalyakina, V. Utochnikova, A. Trigub, Y. Zubavichus, N. Kuzmina and S. Bräse, J. Phys.: Conf. Ser., 2016, 712, 012137 CrossRef.
- A. S. Kalyakina, V. V. Utochnikova, I. S. Bushmarinov, I. V. Ananyev, I. L. Eremenko, D. Volz, F. Roenicke, U. Schepers, R. Van Deun, A. L. Trigub, Y. V. Zubavichus, N. P. Kuzmina and S. Bräse, Chem. – Eur. J., 2015, 21, 17921–17932 CrossRef CAS.
- A. S. Kalyakina, V. V. Utochnikova, I. S. Bushmarinov, I. M. Le-Deygen, D. Volz, P. Weis, U. Schepers, N. P. Kuzmina and S. Bräse, Chem. – Eur. J., 2017, 23, 14944–14953 CrossRef CAS.
- V. V. Utochnikova, M. S. Abramovich, E. V. Latipov, A. I. Dalinger, A. S. Goloveshkin, A. A. Vashchenko, A. S. Kalyakina, S. Z. Vatsadze, U. Schepers, S. Bräse and N. P. Kuzmina, J. Lumin., 2019, 205, 429–439 CrossRef CAS.
- V. V. Utochnikova, A. S. Kalyakina, I. S. Bushmarinov, A. A. Vashchenko, L. Marciniak, A. M. Kaczmarek, R. Van Deun, S. Bräse and N. P. Kuzmina, J. Mater. Chem. C, 2016, 4, 9848–9855 RSC.
- V. V. Utochnikova, E. V. Latipov, A. I. Dalinger, Y. V. Nelyubina, A. A. Vashchenko, M. Hoffmann, A. S. Kalyakina, S. Z. Vatsadze, U. Schepers, S. Bräse and N. P. Kuzmina, J. Lumin., 2018, 202, 38–46 CrossRef CAS.
|
This journal is © The Royal Society of Chemistry 2020 |
Click here to see how this site uses Cookies. View our privacy policy here.