DOI:
10.1039/D0BM01297A
(Paper)
Biomater. Sci., 2021,
9, 199-211
A 3D tissue model-on-a-chip for studying the effects of human senescent fibroblasts on blood vessels†
Received
3rd August 2020
, Accepted 2nd November 2020
First published on 11th November 2020
Abstract
All human tissues experience aging that eventually causes organ dysfunction and disease. Cellular senescence was discovered in fibroblasts cultured in vitro. In adults, it is a primary defense mechanism against cancer, but also a major contributor to lifespan limits and disorders associated with aging. To assess how human blood vessels change in an aged environment, we developed an elementary tissue model-on-a-chip that comprises an in vitro three-dimensional model of a blood vessel embedded in a collagen gel with young or senescent skin fibroblasts. We found that senescent fibroblasts mechanically altered the surrounding extracellular matrix by exerting excessive traction stress. We then found that senescent fibroblasts induced sprouting angiogenesis of a microvessel via their senescence-associated secretory phenotype (SASP). Finally, we gathered evidence that the mechanical changes of the microenvironment play a role in sustaining SASP-induced angiogenesis. The model proved useful in monitoring morphological changes in blood vessels induced by senescent fibroblasts while controlling the proportion of senescent cells, and enabled the study of SASP inhibitors, a class of drugs useful in aging and cancer research.
1. Introduction
All human tissues experience aging that eventually causes organ dysfunction and disease. Senescent cells play important roles in aging as they accumulate over time and contribute to age-related diseases, while their suppression extends lifespans in animal models.1–3 It has been proposed that senescent cells alter their microenvironment, modify tissues and organ functions, and produce age-related phenotypes.3–5
Cellular senescence, a genetic mechanism that prevents cell proliferation by inducing stable cell-cycle arrest, was discovered in fibroblasts cultured in vitro.6,7 In adults, it is a primary defense mechanism against cancer, but also a major contributor to lifespan limits and disorders associated with aging. The main trigger is the shortening of telomeres that activate irreversible cell-cycle arrest through p53 and CDKN2A/p16INK4A molecular pathways. Non-telomeric DNA damage and other cellular stress can also trigger senescence. Senescent cells are characterized by specific changes in morphology, metabolism, and physiology.8,9 Among these changes, senescent cells can exhibit enhanced secretion of growth factors, proteases, and inflammatory cytokines and chemokines, which constitute the senescence-associated secretory phenotype (SASP). There is no single SASP as the secreted products vary by cell type and tissue.10 SASPs can have beneficial or detrimental effects. There are involved in physiological processes, including the early stages of cutaneous wound healing when they prevent tissue fibrosis while stimulating myofibroblast differentiation and wound closure. However, SASPs also promote tissue dysfunction and tumorigenesis, where they induce epithelial-to-mesenchymal transition, stimulate tumor vascularization, and guide cancer-cell migration and invasion of skin cancer.8,11–13
Senescent human dermal fibroblasts stimulate the invasion and migration of human umbilical vein endothelial cells (HUVEC) in vitro and enhance tumor vascularization in mice by secreting a major angiogenic factor, vascular endothelial growth factor (VEGF).14 However, the effect of senescent fibroblasts on dermal blood vessels and their mechanisms remains unknown, in part because identifying senescent cells in vivo has proven challenging.9 Furthermore, in vivo models do not enable detailed studies of senescent fibroblasts’ roles in tissue aging and microenvironment modifications because the number of senescent cells cannot be adjusted. Two-dimensional (2D) culture models, although useful for understanding molecular interactions, fail to reveal three-dimensional (3D) effects seen at a tissue level.
We assessed how human blood vessels change in an aged environment. We used a tissue-engineering approach to develop an elementary tissue model-on-a-chip that allows for an investigation of the changes induced by senescent dermal fibroblasts on a microvessel. We found that senescent fibroblasts mechanically altered the surrounding extracellular matrix (ECM) by exerting excessive traction stress. We then found that senescent fibroblasts induced sprouting angiogenesis of a microvessel via their SASP. Finally, we gathered evidence that the fibroblast's secreted factors only partially explain the occurrence of angiogenesis, suggesting that mechanical changes of the microenvironment play a role in sustaining SASP-induced angiogenesis. The model also proved useful in monitoring morphological changes in blood vessels induced by senescent fibroblasts while controlling the proportion of senescent cells, and enabled the study of SASP inhibitors, a class of drugs useful in aging and cancer research.
2. Materials and methods
2.1. Cell culture and induction of senescence in skin fibroblasts
All cells were maintained at 37 °C in a humidified atmosphere of 5% CO2/95% air. Primary human umbilical vein endothelial cells (HUVEC; Lonza, Basel, Switzerland) were cultured in Endothelial Cell Growth Medium-2 BulletKit (EGM-2; Lonza) and used between passages 4 to 7. Primary human skin fibroblasts HCA2 (MJ90) were previously isolated from neonatal foreskin by the laboratory of Judith Campisi (University of California San Francisco, USA). HCA2 were cultured in high glucose Dulbecco's Modified Eagle's Medium (DMEM) supplemented with 10% fetal bovine serum (FBS; Biosera, Nuaille, France) and 1% penicillin–streptomycin (pen–strep; FUJIFILM Wako Pure Chemical Corporation, Osaka, Japan). For passaging, cells were rinsed once with 1× Dulbecco's phosphate buffered saline (−) (PBS; FUJIFILM Wako Pure Chemical Corporation), incubated with 0.25 w/v% trypsin-5.3 mmol L−1 EDTA-4Na solution (trypsin; FUJIFILM Wako Pure Chemical Corporation) for up to 5 minutes at 37 °C, and harvested in culture medium.
To induce senescence of skin fibroblasts, 70–80% confluent early passage HCA2 were irradiated in culture medium with 10 Gy of γ-ray by using a MBR-1520R-3 (Hitachi, Tokyo, Japan). Senescent cells were then selected by culturing for 14 days with DMEM containing 10% FBS and 100 nM BI2536 (Plk1 inhibitor; Cayman Chemical, MI, USA), and senescence phenotype was first checked by a canonical β-galactosidase assay.15
2.2. Measurement of fibroblast spreading area (two-dimensional (2D) study)
Normal or senescent fibroblasts were seeded onto culture dishes coated with Cellmatrix Type I-P (pepsin-solubilized collagen derived from porcine tendons, 3 mg mL−1, pH 3; Nitta Gelatin, Osaka, Japan). After 24 hours, phase contrast images of spread cells were taken with an inverted microscope Observer Z1 (Carl Zeiss, GmbH, Oberkochen, Germany) equipped with a 10× objective lens (LD Plan-NeoFluar 20×/0.4 Korr Ph2 M27) and the ZEN 2 blue edition software (version 2.0.0.0, 64 bit, Carl Zeiss). Cells were then fixed with 4% (w/v) paraformaldehyde in PBS (FUJIFILM Wako Pure Chemical Corporation) and processed by immunofluorescence for staining the actin cytoskeleton in green (Alexa Fluor 488 phalloidin; Thermo Fisher Scientific, Waltham, MA, USA) and CDKN2A/p16INK4a in red (ab108349; Abcam, Cambridge, UK). Fluorescence images were taken with the microscope Observer Z1 by using a 20×- and a 10×-objective lens for normal and senescent fibroblasts, respectively. A single cell's spreading area was measured based on the image of actin cytoskeleton and by using the following method in ImageJ software (National Institute of Health, USA): (i) the “split color” function was applied and the green channel image was selected, (ii) a single cell was selected using the “ROI” tool, (iii) the threshold was set, (iv) the function “despeckle” was applied, and (v) the “measure” tool was used to obtain the spreading area. The average single cell spreading area was calculated based on at least 50 cells per condition.
2.3. Traction force microscopy (2D study)
Traction force of young and senescent fibroblasts were measured by calculating poly(acrylamide) (PAAm) gel's deformation caused by the adhesion and detachment of a cell. The deformation was visualized by the displacement of fluorescent microbeads, which were incorporated into the PAAm gel.16,17
A PAAm gel (2.4% w/w acrylamide and 0.3% w/w bisacrylamide) containing fluorescent polystyrene beads ∅ 0.2 μm (Fluoro-max dyed green aqueous fluorescent particles, Thermo Fisher) was polymerized between silanized and siliconized glass coverslips. The Young's modulus of the obtained PAAm gel was 851 Pa as measured with an atomic force microscope (Nano Wizard, JPK Instruments, Berlin, Germany) in contact mode. The gel surface was activated with sulfo-SANPAH (Thermo Fisher) and coated with 0.1 mg mL−1 collagen type I (CellMatrix Type I-C, Nitta Gelatin, Japan). Fibroblasts were then seeded at a density of 2500 cells per dish (260 cells per cm2) and cultured at 37 °C for 24 h.
To calculate the traction force, fluorescent images of the beads were captured by an inverted fluorescent microscope (IX-81, Olympus, Tokyo, Japan) with UPlanSApo lens (20×/NA: 0.75, Olympus). Images were taken before and after cell removal by trypsin-EDTA treatment. Traction stress was calculated from the before/after fluorescent images by Fourier Transform traction cytometry with ImageJ plugin.18 The average and maximum traction stress were calculated for a spreading area of an entire cell. Five young and six senescent fibroblasts were analyzed.
2.4. Collagen gel contraction assay (three-dimensional (3D) study)
A neutralized collagen solution was prepared on ice by mixing at a volume ratio 8
:
1
:
1: Cellmatrix Type I-P, 10× Hank's buffer (Sigma-Aldrich, Saint Louis, MO, USA)., and 10× collagen buffer (NaHCO3 262 mM, NaOH 0.05 M—Kanto Chemical, Tokyo, Japan—and HEPES 20 mM—Sigma-Aldrich).
Droplets of fibroblasts-containing collagen solution (10 μL, 500 cells per droplet) were deposited onto a UV-sterilized parafilm and allow to gelate at 37 °C for 30 minutes, generating cell-encapsulating collagen gel beads. The gel beads were covered with cell culture medium (DMEM with 10% FBS, 1% pen–strep) and cultured for 20 hours. Medium was then drained and remaining liquid was allowed to evaporate for 10 minutes. Phase contrast images were taken with the microscope Observer Z1 equipped with a 5×-objective lens. Collagen spreading area was measured using the ZEN 2 blue edition software. Droplets without embedded cells were used as a negative control. Six collagen beads per condition were measured and the experiment was performed twice.
2.5. Preparation of a 3D microvessel-on-a-chip with fibroblasts in the microenvironment
The polydimethylsiloxane (PDMS)-based chips (25 mm × 25 mm × 5 mm: width × length × height) and their fabrication method were previously described.19 To prepare a 3D model of a microvessel with young or senescent skin fibroblasts in its microenvironment, a PDMS chip was treated with air plasma (basic plasma cleaner; Harrick Plasma, Ithaca, NY, USA) for one minute and sterilized by UV-light under a cell culture hood. A ∅ 200 μm acupuncture needle (No. 02, 0.20 mm × 30 mm, J type; Seirin, Shizuoka, Japan) was coated with 1% BSA in PBS and also sterilized by UV-light. A neutralized collagen solution was prepared as described in the previous section. To prepare the model of co-culture with 0 and 100% senescent fibroblasts, young and senescent HCA2, respectively, were harvested with EGM-2 and resuspended in the collagen solution at a density of 50
000 cells per mL. For the co-culture with 50% senescent fibroblasts, young and senescent HCA2 were harvested, mixed at a ratio 1
:
1 and resuspended at a density of 50
000 cells per mL in the collagen solution, as to ensure an equal number of fibroblasts in each model. Fibroblasts-containing collagen solution was introduced into the microvessel chamber and the BSA-coated acupuncture needle was inserted through the PDMS channel. The chip was incubated at 37 °C for one hour to enable gelation of collagen solution, and the acupuncture needle was withdrawn to leave an empty channel in the collagen gel. 4 μL of a 1 × 107 cells per mL HUVEC suspension were introduced into the channel and the cells were let to attach onto the collagen scaffold at 37 °C for 15 minutes. Warm EGM-2 was then added and the 3D model was cultured at 37 °C until use with medium change every other day.
When necessary, at the end of experiments, microvessels were stained with 20 μg mL−1 Rhodamine-conjugated Ulex europaeus Agglutinin 1 lectin (UEA-1; Vector Laboratories, Burlingame, CA, USA) in EGM-2 for 30 minutes at 37 °C, and imaged with the microscope Observer Z1 piloted with ZEN 2 blue edition software (Carl Zeiss).
2.6. SASP-inhibition assay
To check whether senescent fibroblasts expressed SASP in the 3D model, a previously-reported SASP inhibitor was used. 24-hours after making microvessels with young or senescent fibroblasts in their microenvironments, culture medium was replaced by fresh medium containing 50 nM rapamycin (ab120224, Abcam) or 0.1% DMSO—as a control—and further cultured for three days.
2.7. Conditioned medium assay
To study the effects of soluble factors secreted by young or senescent fibroblasts, conditioned medium was prepared. Confluent young or senescent fibroblasts were cultured with EGM-2, and medium was harvested every other day for six days. Medium was diluted 1
:
2 with fresh EGM2 to obtain the so-called conditioned medium.
3D models were prepared without fibroblasts, i.e., only collagen-gel-embedded microvessels were made. Microvessels were cultured with conditioned medium, with medium renewal every other day, for up to seven days. The experiment was performed twice with three microvessels per condition.
2.8. Permeability assay
The permeability of microvessels cultured with conditioned medium was analyzed as previously reported.20 The microvessel was stained with rhodamine-conjugated UEA-1 as described above and the chip was then set on a confocal microscope (CLSM; Laser Scanning Microscope 700, LSM 700, Carl Zeiss) in an incubation chamber that maintained 37 °C in a humidified 5% CO2/95% air atmosphere (INU Incubation System for Microscopes-model WSKM, Tokai Hit Co., Fujinomiya, Japan). Images were taken using the CLSM equipped with a 10× observation lens (N-Achroplan 10×/0.25 M27). The rhodamine-conjugated UEA-1 staining detected with the 555 nm-wavelength laser was used to focus the center of the microvessel. Fluorescein isothiocyanate-dextran (70 kDa, FITC-dextran from Leuconostoc spp., Mr 450
000–650
000; Sigma-Aldrich; 200 μg mL−1) in EGM-2 was introduced in both wells, and imaging was performed by detecting the fluorescence of FITC with the 488 nm-wavelength laser and an optical section of 77.8 μm. For quantifying the microvessel permeability, CLSM images taken one minute after introduction of FITC-dextran were used. The mean fluorescence intensity of FITC detected in collagen gel was measured as the average values between two regions of interest (0.1 mm × 2 mm, 0.2 mm2). Four microvessels per conditions were analyzed.
2.9. Western blot detection of senescent marker
2D-cultured fibroblasts were directly lysed with Laemmli-buffer (2% SDS, 10% glycerol, 5% 2-mercaptoethanol, 0.002% bromophenol blue, and 62.5 mM Tris HCl at pH 6.8). The whole lysates (20–50 μg) were separated by SDS-PAGE, transferred onto a PVDF (Immobilon-P; Millipore, Burlington, MA, USA) membrane, and then subjected to immunoblotting with anti-CDKN2A/p16Ink4a (ab108349, Abcam) or anti-beta-actin (sc-47778, Santa Cruz Biotechnology, Inc., Dallas, TX, USA) antibody using the ECL detection system.
2.10. Analysis of gene expression in the 3D model by RT-qPCR
To obtain enough RNA to perform a reverse transcriptase quantitative polymerase chain reaction (RT-qPCR), three microvessels in their collagen beds were pooled. Cells were extracted from collagen gel by treatment with collagenase type I (FUJIFILM Wako Pure Chemical Corporation), pelleted, and lysed with ISOGEN (Nippon Gene, Tokyo, Japan). The total RNA was purified by chloroform extraction and ethanol precipitation, and reverse transcribed using High-Capacity cDNA Reverse Transcription Kit (Thermo Fisher Scientific, Waltham, MA, USA). qPCR analysis was conducted using TaqMan Gene Expression Master Mix and the TaqMan probes of the tested genes (Thermo Fisher Scientific; see ESI Table S1†). Gene expression levels were analyzed by the ΔΔCT method.
2.11. Immunofluorescence
The samples (2D or 3D) were fixed with 4% (w/v) paraformaldehyde in PBS (FUJIFILM Wako Pure Chemical Corporation) for 30 minutes, and permeabilized with 0.5% Triton X-100 in PBS for 10 minutes at 25 °C. Blocking was performed by incubating overnight at 4 °C with blocking solution (1% (w/v) BSA in PBS). Samples were then incubated overnight at 4 °C with a primary antibody (vascular endothelial (VE)-cadherin D87F2, Cell Signaling Technology; CDKN2A/p16Ink4a, 108349, Abcam) diluted in blocking solution. After PBS washes, samples were incubated for 2 hours at 25 °C with a secondary antibody (Alexa Fluor 568 goat anti-rabbit; Thermo Fisher Scientific), or Alexa Fluor 488 phalloidin (Thermo Fisher Scientific) for actin staining, diluted in blocking solution. After PBS washes, cell nuclei were stained by incubating for 15 minutes at 25 °C with Hoechst 33342 (Thermo Fisher Scientific) diluted in PBS. After PBS washes, samples were stored at 4 °C.
Images were taken using the microscope Observer Z1 or the CLSM equipped with a 40× water-immersion detection objective lens (LD C-Apochromat 40×/1.1 W Korr M27). Red fluorescence, green fluorescence, and Hoechst 33342 were detected using 488, 555 and 405 nm-wavelength lasers, respectively. Maximum intensity projection (MIP) images were obtained with the ZEN 2 blue edition software.
2.12. Histological analysis
One microvessel per condition were fixed with 4% paraformaldehyde, dehydrated with graded alcohol and graded xylene, embedded in paraffin and cut into 8 μm sections. Sections were then deparaffinized with xylene, rehydrated, and stained with Masson's trichrome. To quantify collagen fibers in the vicinity of fibroblasts co-cultured with a microvessel, images were taken with the microscope Observer Z1 by using a 20×-objective lens and an Axiocam105color camera. Images were analyzed with ImageJ as follow: (i) the blue channel image was processed with the “bandpass filter” function; (ii) a region of interest (ROI) was drawn following the fibroblast's edge, then enlarged with the “enlarge” function to include a 10 μm band between the cell's edge and the limit of the ROI; (iii) the image was binarized; (iv) the cell was erased from the image with the “clear” function; (v) the area occupied by collagen fibers and the size of fibers were measured with the plugins “analyze particles” and “cluster”, respectively. The density of collagen fibers was calculated as the ratio of the area occupied by collagen fibers to the total area of the ROI remaining after the cell was erased, and expressed as a percentage. Regarding the size of fibers, as the analysis was performed on 2D images, it was not possible to know whether the diameter or the length was measured. The “size” expressed thus an estimation of the dimensions of fibers rather than an actual measurement, consequently we use the expression “cluster size”. Results show as mean ± standard deviation from eight ROIs per condition.
2.13. Statistical analysis
Two-tailed Student's t test was used to compare two groups; a p value strictly inferior to 0.05 led to reject the null hypothesis that read as “the means of the two groups are equal”, and therefore to consider the difference between the two tested groups as statistically significant. Dunnett's test was used to compare one group to other groups; a p value strictly inferior to 0.05 indicated that the difference between the test group and the other groups was significant.
The qPCR data were analyzed by a Welch's test when two groups were compared, and by an ANOVA followed with a Tukey-Kramer test when more than two groups were compared. For the Welch's test a p value strictly inferior to 0.05 indicated a significant difference between the two tested groups, and for the ANOVA a p value strictly inferior to 0.05 indicated that the difference between the groups were significant and justified to perform a post hoc test.
3. Results
3.1. Senescent skin fibroblasts cause contractions of collagen gel
We investigated the effects of senescent skin fibroblasts on blood vessels using an in vitro model of a microvessel-on-a-chip. The model, a variation of a previously published version,20 comprises a tubular endothelial tissue embedded in a gel of ECM in which young or senescent fibroblasts are incorporated (Fig. 1).
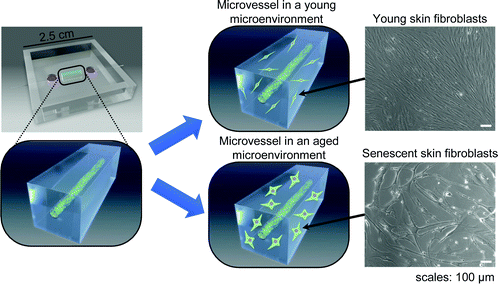 |
| Fig. 1 Conceptual sketch of the study about a 3D model for the monitoring of a microvessel in a young or aged microenvironment. A tubular endothelium is fabricated within a collagen gel in a PDMS chip; young or senescent human-skin fibroblasts are embedded in the collagen gel to make a young or aged environment. | |
To investigate whether senescent fibroblasts physically modify their microenvironment, we focused on a morphological and mechanical analysis of the cells and their ECM. One of the obvious morphological changes experienced by aging fibroblasts is an increase in cell spreading area associated with cytoskeleton changes.21 We first measured the single-cell spreading area of young and ionizing-radiation-induced senescent fibroblasts from immunofluorescence (IF) images of actin cytoskeleton (Fig. 2A). Quantification showed that the area for senescent fibroblasts increased by a factor of 15. A decrease in cell motility was also observed (data not shown), suggesting an alteration to the cytoskeleton. In addition to a wider cell spreading area, a senescence phenotype was visualized in the form of increased expression of the senescent marker CDKN2A/p16INK4a as detected by IF and confirmed by western blot (Fig. 2A and B), and the absence of proliferation (data not shown).
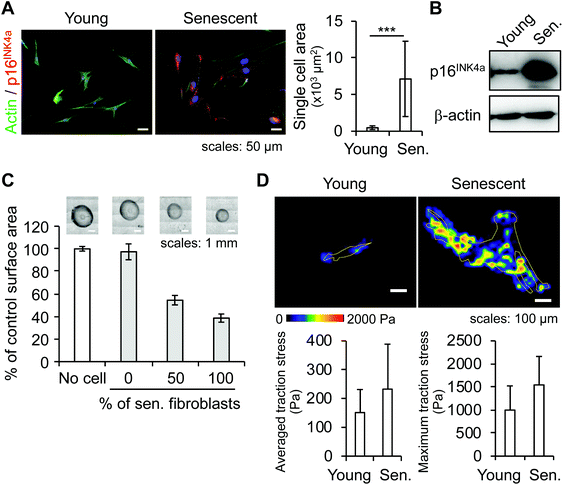 |
| Fig. 2 Characterization of senescent skin fibroblasts. (A & B) Characterization of senescent skin fibroblasts: (A) spreading cell area measurement from actin-IF images of young and senescent fibroblasts. At least 50 cells per condition were analyzed. Error bars: standard deviations, p < 0.001. (B) Western blot detection of the senescent marker protein CDKN2A/p16INK4a; β-actin is used as a loading control. (C & D) Analysis of the mechanical effects of skin fibroblasts on their environment: (C) collagen gel contraction assay: young or senescent fibroblasts were embedded in collagen gel beads; the gel contraction is measured as the size difference between a fibroblast-embedded collagen bead and a bead without cells. Six beads per condition were measured. Error bars: standard deviations. (D) Traction force microscopy: measurement of traction stress exerted by fibroblasts on a PAAm gel (851 Pa). At least five cells per condition were analyzed (see also Fig. S1†). Error bars: standard deviations. | |
To study the mechanical changes caused by skin fibroblasts on their surrounding ECM, a collagen gel contraction assay was performed in which young and senescent fibroblasts were cultured in beads of collagen gel for approximately 20 hours. While young fibroblasts had little effect on the size of the bead, senescent fibroblasts reduced bead size by more than half (Fig. 2C). A co-culture of young and senescent fibroblasts at a ratio 1
:
1 (i.e., 50% of senescent cells in a bead) showed that the reduction level was linked to the amount of senescent fibroblasts present in a bead. This result suggests that senescent fibroblasts exerted abnormally strong traction forces that caused collagen gel to contract.
To characterize the mechanical forces exerted by fibroblasts, we performed traction force microscopy. Averaged traction stresses were 151 ± 80 Pa and 233 ± 156 Pa for young and senescent fibroblasts, respectively, while maximum traction stresses were 999 ± 525 Pa and 1545 ± 616 Pa for young and senescent fibroblasts, respectively (Fig. 2D). Furthermore, the areas with a traction stress equal or superior to 500 Pa represented 4.9% and 12.7% of the total cell spreading area of a young and senescent fibroblast, respectively (Fig. S1A†). This suggests that senescent fibroblasts tended to impose a stronger traction stress on their environment. As senescent fibroblasts spread in multiple directions (Fig. 2D–upper panel), traction stress was also applied in several directions, contrary to the effect in young fibroblasts, in which traction stress occurred mainly in two directions (Fig. S1B†).
These results indicate that morphological changes in senescent fibroblasts led to a mechanical alteration of the ECM, possibly through a stronger traction stress.
3.2. Senescent skin fibroblasts induce sprouting angiogenesis
In sprouting angiogenesis, new blood vessels form from a pre-existing vessel. This phenomenon is orchestrated by DLL4, which is expressed in endothelial cells that detect VEGF. Some senescent fibroblasts reportedly promote tumor vascularization in mice by secreting VEGF, and conditioned medium from senescent fibroblasts stimulated HUVECs to invade a basement membrane.14 To investigate the effects of senescent skin fibroblasts on a blood vessel, we created a tissue model-on-a-chip that consisted of a tubular endothelium approximately 200 μm in diameter (a microvessel) surrounded by a collagen gel with embedded young or senescent skin fibroblasts. The model was cultured for four days and the morphological changes in the microvessel were visualized by fluorescence microscopy.
Previous results (Fig. 2) revealed that senescent fibroblasts mechanically alter their microenvironment, ultimately causing a contraction of collagen gel. In the 3D tissue model with embedded senescent fibroblasts, a contraction of the collagen gel consistently occurred in several, but not all, samples, resulting in a reduction of the microvessel's lumen diameter (Fig. 3A, right panel), and hindering microscopy analysis. When only 50% senescent fibroblasts (1
:
1 ratio of young to senescent fibroblasts) were used, contraction of collagen gel was prevented (Fig. 3A, central panel). Samples for which collagen contraction was limited or absent were therefore used for microscopy analysis.
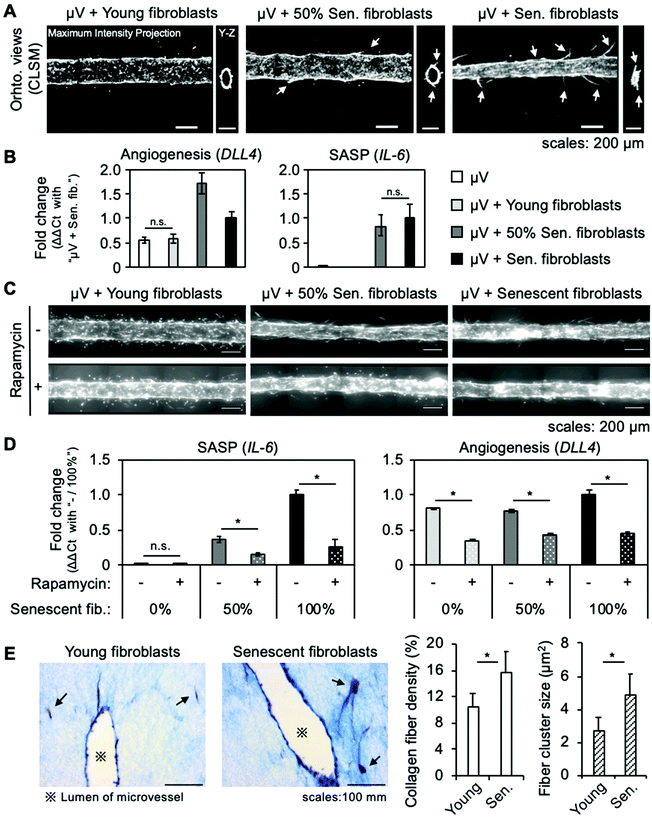 |
| Fig. 3 Effects of a senescent microenvironment on a human microvessel model. (A & B) Microvessels co-cultured with young and senescent skin fibroblasts: (A) orthographic (ortho.) views by CLSM of fixed microvessels visualized by IF detection of actin. Y–Z views reveal the lumen of microvessels. White arrows indicate angiogenic sprouts. This panel shows representative samples among seven microvessels in two experiments. (B) RT-qPCR results of the expression variation of DLL4 and IL-6 genes. Three microvessels were pooled and used for extracting RNA. The target gene's relative expression was measured through a comparison with the B2M gene. The variation of expression (fold change) was calculated by the ΔΔCT method, with the microvessels co-cultured with senescent fibroblasts (black bar) as the reference. IL-6 gene expression was not detected in the microvessels co-cultured with young fibroblasts (light gray bar). Error bars: range of fold change; p < 0.05, n.s.: no significance (ANOVA). (C & D) Fibroblast-co-cultured microvessels treated with 50 nM of the SASP inhibitor rapamycin. (C) Maximum intensity projection (MIP) views by an epifluorescent microscope of non-fixed microvessels visualized by transient staining with rhodamine-conjugated UEA1. White arrows indicate angiogenic sprouts. This panel shows representative samples among four and seven microvessels, for rapamycin, − and + respectively, in two experiments. (D) RT-qPCR results of expression variation of IL-6 and DLL4 genes. Three microvessels were pooled and used for extracting RNA. The target gene's relative expression was measured by comparison with the B2M gene. The variation of expression (fold change) was calculated by the ΔΔCT method, with the microvessels co-cultured with senescent fibroblasts in the absence of rapamycin (plain black bar) as the reference. Error bars: range of fold change; p < 0.05, n.s.: no significance. (E) Collagen fibers visualization and analysis: Masson's trichrome staining with measurement of collagen fibers’ density and cluster size. Collagen fibers’ density is expressed as a percentage of region of interest (ROI). Black arrows indicate young or senescent fibroblasts. Eight ROIs were analyzed. Error bars: standard deviations; p < 0.05. | |
Microvessels co-cultured with young fibroblasts did not show any morphological change (Fig. 3A and ESI Fig. S2,† left panel). With 50% senescent fibroblasts, some newly formed capillary-like structures were observed (Fig. 3A and ESI Fig. S2,† central panel). With only senescent fibroblasts, numerous long capillary-like structures formed (Fig. 3A and ESI Fig. S2,† right panel), suggesting a stronger effect. Furthermore, RT-qPCR showed that co-culturing with young fibroblasts did not increase DLL4 expression (Fig. 3B, left), confirming that it was actually a sprouting angiogenesis mechanism that was activated by the co-culture with senescent fibroblasts.
These results demonstrate that senescent skin fibroblasts induced sprouting angiogenesis in the microvessel. It also shows that our model enables to study the interaction between senescent fibroblasts and a blood vessel at the tissue and molecular levels, and raises the possibility of tuning the senescence level of the microenvironment.
3.3. Angiogenesis induced by senescent skin fibroblasts is SASP-dependent
Senescent cells acquire a SASP, which turns them into pro-inflammatory cells. Because some factors of the SASP can be pro-angiogenic, we decided to check whether a SASP occurred in the model. A SASP contained a plethora of factors and a composition that varies by cells and tissues; however, it is established that the most prominent pro-inflammatory cytokine is the interleukin 6 (IL-6).11 We thus used the IL-6 gene expression's increase as a marker of cells that have a SASP. RT-qPCR showed that, while IL-6 expression was not detected in microvessel monocultures and co-cultures with young fibroblasts, it was confirmed in co-cultures with senescent fibroblasts (Fig. 3B, right). The increase in IL-6 expression was lower with 50% senescent cells than when only senescent fibroblasts were embedded in collagen gel, indicating that IL-6 was expressed only by senescent fibroblasts. This result indicates that a SASP occurred in the model and suggests that pro-angiogenic factors were secreted.
To confirm whether the angiogenesis was due to a SASP, we used rapamycin, an mTOR inhibitor. The mTOR protein is a key element of the regulation of a SASP and it has been reported that its inhibition by rapamycin reduces SASPs in senescent human fetal lung myofibroblasts.22 Beginning 24 hours after fabrication, microvessels co-cultured with young and senescent fibroblasts were treated with rapamycin for three days. While control samples containing senescent fibroblasts recapitulated previous results by showing sprouting angiogenesis, samples treated with rapamycin showed no signs of angiogenesis (Fig. 3C). RT-qPCR showed that rapamycin reduced IL-6 expression in samples with senescent fibroblasts, demonstrating that a SASP was inhibited (Fig. 3D, left). Furthermore, DLL4 expression did not increase in samples treated with rapamycin, confirming that sprouting angiogenesis was not activated (Fig. 3D, right).
These results confirmed that a SASP was the main inducer of sprouting angiogenesis in the model. They also demonstrated that the model can be used for studying senotherapeutics.
3.4. Senescent skin fibroblasts rearrange ECM fibers
Because the collagen gel was visibly altered by the presence of senescent fibroblasts, we investigated the collagen fibers’ morphology in the co-cultured microvessel with Masson's trichrome staining. The collagen fibers’ density and size in the vicinity of a fibroblast cell were measured by image processing (ESI Fig. S3A†), which revealed a higher collagen fiber density when senescent fibroblasts were embedded: 10.5 ± 1.9% and 15.7 ± 3.0% in a co-culture with young fibroblasts and senescent fibroblasts, respectively. Furthermore, the cluster sizes was higher around the senescent cells (Fig. 3E); the average size was 2.7 ± 0.9 μm2 and 4.9 ± 1.2 μm2 around young and senescent cells, respectively. To assess whether these effects were due to rearrangement of collagen fibers or biochemical changes caused by a SASP (e.g., digestion and neosynthesis), Masson's trichrome staining was performed on a model treated with rapamycin (ESI Fig. S3B†). The results revealed no difference in collagen fiber density around senescent fibroblasts, whether treated or not, and a tendency for a small reduction in cluster size when rapamycin was used. This suggests that the increase of collagen density around senescent fibroblasts was due mainly to physical rearrangement, and that the SASP played a role in increasing the cluster size.
The directionality of ECM fibers was studied by confocal reflection microscopy (ESI Fig. S4†). While the ECM fibers appeared aligned in the direction of the longer side of a young fibroblast, with a senescent fibroblast, the ECM fibers appeared to be pulled from different directions. This disorganization may be attributable to differences in cell morphology: while young fibroblasts had a typical spindle-shaped structure with two extremities at which ECM fibers converged, senescent fibroblasts exhibited diverse shapes with several points of ECM fibers’ convergence. This is supported by the results of traction force microscopy obtained from a 2D culture that revealed senescent fibroblasts exerted forces in more points compared with young cells (Fig. 2D). In addition, force vectors showed no directional tendency, in contrast with young fibroblasts, which were oriented from the two extremities of a cell toward its center (ESI Fig. S1B†). The results and those of the study of mechanical forces exerted by senescent skin fibroblasts (Fig. 2) suggest that the increase in collagen fiber density around the cells was due to mechanical rearrangement of ECM fibers by senescent skin fibroblasts.
3.5. Factors secreted by senescent skin fibroblasts induce inflammation of endothelium
The above results showed that sprouting angiogenesis of a microvessel was activated by a co-culture with senescent skin fibroblasts and inhibited by a SASP inhibitor. However, senescent fibroblasts also altered physically their surrounding ECM, with increased traction forces possibly involved. This led to question whether senescent fibroblast's secreted factors alone were sufficient to sustain angiogenesis or the physical presence of fibroblasts played a part. To test this hypothesis, a conditioned medium experiment was performed. Briefly, medium containing all factors secreted by young or senescent fibroblasts (EGM2Young, EGM2Senescent) was harvested and used to culture microvessels, i.e., a model without fibroblasts embedded in the collagen gel. After four days, microvessels were observed and a vascular permeability assay was performed by introducing 70 kDa fluorescein isothiocyanate (FITC)-dextran into the lumen of microvessels. Although some endothelial cells were seen migrating and forming bumps on the edges of the microvessel, no clear angiogenic sprouts were observed (Fig. 4A, left panel). RT-qPCR confirmed sprouting angiogenesis was not activated as DLL4 expression was not increased (Fig. 4A, right panel).
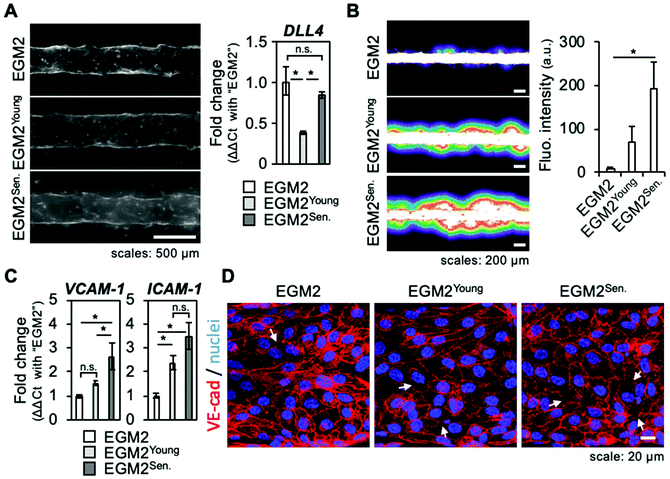 |
| Fig. 4 Effect of soluble factors secreted by senescent fibroblasts on a human microvessel model. (A) Microvessels cultured with conditioned medium: EGM2 is the normal medium for culturing HUVECs, EGM2Young is EGM2 conditioned with young fibroblasts, and EGM2Sen. is EGM2 conditioned with senescent fibroblasts (cf. Materials and Methods section for details). (A, left) MIP views by epifluorescent microscopy of non-fixed microvessels visualized by transient staining with rhodamine-conjugated UEA1; representative samples among six microvessels in two experiments. (A, right) RT-qPCR result of the expression variation of DLL4 gene; three microvessels were pooled for RNA extraction; DLL4 relative expression was measured by comparison with the B2M gene, and the variation of expression (fold change) was calculated by the ΔΔCT method with the microvessels cultured with EGM2 (white bar) as the reference. Error bars: range of fold change; p < 0.05, n.s.: no significance (ANOVA). (B) Permeability assay on microvessels cultured with conditioned medium. (B, left) CLSM views of FITC-dextran (70 kDa) leaking from microvessels into the collagen gel (color-scaled image). (B, right) Quantification of the fluorescence intensity of leakage; the leakages for three microvessels per experimental condition were quantified. Error bars: standard deviations; p < 0.05. (C) Inflammation of the endothelium: RT-qPCR results of expression variation of the inflammatory genes VCAM-1 and ICAM-1; three microvessels were pooled for RNA extraction; gene relative expression was measured by comparison with the B2M gene and the variation of expression (fold change) was calculated by the ΔΔCT method with the microvessels cultured with EGM2 (white bar) as the reference. Error bars: range of fold change; p < 0.05, n.s.: no significance (ANOVA). (D) Tissue cohesion of the endothelium: MIP views by CLSM of the adherens junctions between the endothelial cells of microvessels cultured with conditioned medium; adherens junctions were visualized by IF detection of VE-cadherin. White arrows indicate regions missing VE-cadherin. | |
Vascular permeability assay revealed that microvessels cultured with the conditioned media had an impaired barrier function as the leakage of 70 kDa FITC-dextran was stronger for these culture conditions (Fig. 4B). The barrier function impairment was even stronger for microvessels cultured with EGM2Senescent. RT-qPCR that measured expression of endothelium's inflammatory factors such as VCAM-1 and ICAM-1 showed that microvessels cultured with conditioned media were in an inflamed state (Fig. 4C). An immunofluorescence study of adherens junctions using detection of VE-cadherin (VE-cad) and actin cytoskeletons was performed to visualize the cohesion of endothelial tissue. The cells of microvessels cultured with EGM2Young had a mainly cortical actin cytoskeleton, maintained VE-cad, and showed an elongated shape, hinting at effective tissue cohesion (Fig. 4D and ESI Fig. S5†). However, the cells of microvessels cultured with EGM2Senescent had many actin stress fibers, and were round-shaped with more areas lacking VE-cad. These observations indicated that tissue cohesion was strongly impaired by the culture with EGM2Senescent. When microvessels were cultured for six days with conditioned medium, few angiogenic sprouts were observed in microvessels cultured with EGM2Young, whereas tissue cohesion was completely lost in microvessels cultured with EGM2Senescent (ESI Fig. S6†). These results suggest that factors secreted by skin fibroblasts induced inflammation of the endothelium with two different outcomes: factors secreted by young fibroblasts did not alter tissue cohesion while factors secreted by senescent fibroblasts caused endothelial disruption, hinting at stronger inflammatory effect.
These results show that factors secreted by senescent fibroblasts induced inflammation (as expected from SASP), but were not sufficient to sustain angiogenesis. They also suggest that the physical presence of fibroblasts had a regulatory effect. It is reasonable to infer that the physical changes in the microenvironment induced by senescent fibroblasts played a role in orienting the SASP's ability to sustain angiogenesis rather than disrupt the endothelium. Molecular cross-communication between endothelial cells and fibroblasts may also have played a part in the outcome of co-culturing through a mechanism similar to the auto- and paracrine feedback loops of SASP that have been suggested as regulations of the onset of senescence.23–25
4. Discussion
As the largest of the organs, the skin protects the internal body from environmental harms. Like all organs, it suffers from aging brought on by intrinsic disposition and extrinsic factors. While the outermost layer of the skin, the epidermis, provides a protective barrier that can remove extrinsic damage by continual shedding, the lower layer, the dermis, is more prone to intrinsic and extrinsic aging and accumulative damage. The dermis comprises an ECM secreted by fibroblasts that gives the skin its resistance to tears and elasticity and blood vessels that provides nutrients to the epidermis and immune defense. Consequently, aging of the dermis weakens the barrier function. Skin aging has been extensively studied, and accumulation of CDKN2A/p16INK4A-positive, non-proliferative fibroblasts has been observed in aged human dermis, showing that senescence occurs in vivo.2,26,27 Skin contains between 20% and 60% senescent fibroblasts, which alter young fibroblasts, matrix production and induce age-related skin pigmentation.5,28,29 In the present study, the organ-on-a-chip approach allowed us to study the fibroblast–endothelium interaction independent of other factors. By using young and senescent skin fibroblasts, we investigated how aged fibroblasts affect a healthy endothelium.
We found that senescent fibroblasts tended to cause greater traction stress on their environment than did young cells. For endothelial cells, aging leads to stronger traction forces associated with vascular endothelial dysfunction. From a molecular point of view, the RhoA/Rho-associated protein kinase (ROCK) pathway, a regulator of the contraction/relaxation of cells among other things, plays a major role in this phenomenon and its inhibition has been investigated as a therapeutic strategy.30,31 In fibroblasts, it has been shown that traction forces are mainly regulated by ROCK.12 The increased traction stress that we observed for senescent fibroblasts may therefore be explained by similar age-related increased activity in the RhoA/ROCK pathway. From a mechanical point of view, our results suggest that the anisotropy of a strong traction force and extensive adhesion of senescent fibroblasts induced anisotropic microenvironment changes.
Pioneering research proposed that the primary function of fibroblasts’ traction forces is the morphogenic rearrangement of the ECM.32 In our 3D tissue model, we found that senescent fibroblasts caused severe morphogenic rearrangement of the ECM and contraction of ECM gel. The model suggests that excessive traction stress from aged fibroblasts in the dermis may play a role in the visual aspects of aged skin. Further research on this point may provide opportunities to exploit the mechanical properties of senescent fibroblasts to protect the dermis from the effects of aging.
Regarding the effect of fibroblasts on the endothelium, it is nowadays well established that fibroblasts play a role in angiogenesis but their detailed effect is still investigated. Fibroblasts are quite different cells depending on their tissue of origins.33 Consequently, previous studies with in vitro models similar to ours showed that both lung and dermal fibroblasts supported angiogenesis;34–37 however, in the studies using dermal fibroblasts, angiogenesis required the addition of angiogenic factors in the culture medium, suggesting that dermal fibroblasts were not the inducer of angiogenesis but rather supported the growth of angiogenic sprouts. Evidence suggests that the effect of dermal fibroblast on angiogenesis relates to their ability to stiffen the ECM.38 In our study, we did not observe angiogenesis when young dermal fibroblast were used. However, senescent dermal fibroblasts induced angiogenesis without the addition of soluble factors, and the mechanical stress, which they exerted on the ECM, contributed to sustain the growth of angiogenic sprouts. When a microvessel was co-cultured with fibroblasts including 50% of senescent cells, angiogenesis was limited. With 100% of senescent fibroblasts, angiogenesis was extensive, with long, thin, and sometime branched sprouts. This dependency to the amount of senescent cells confirmed that the angiogenesis was induced by senescent dermal fibroblasts.
Artificial aging induced by ultraviolet (UV) irradiation in a mouse skin model showed a transient and profound increase in angiogenesis that is later repressed by apoptosis.39 In human skin, the density of blood capillaries is reduced in the UV-exposed area, and appears to decrease from the age of 60.40,41 Analysis of skin specimens from a variety of human subjects, from fetuses to 85-year-olds, revealed that, although blood vessel numbers diminished with age, VEGF content increased.42 The same study reported that the number and proliferative pool of fibroblasts also decreased with age. In our model, the co-culture with 100% of senescent fibroblasts may have thus recapitulated the results of UV-induced aging, while the 50% senescent cells co-culture condition was closer to the physiology of an aged skin, which harbors 20% to 60% senescent fibroblasts.29 Our results suggest thus, that senescent fibroblasts may play a role in the increased VEGF levels in aged skin.
Observation of senescent-fibroblast-induced angiogenesis may bring insights into the relationship between blood vessels and the aging of the dermis. Aging skin is characterized by a loss of tissue elasticity. This tissue stiffening has been explained by the fact that the ECM undergoes deep modifications, with fragmented collagen and elastic fibers that lose their original organization, and proteoglycans of a reduced molecular weight.43,44 Metalloproteases (MMPs) present in SASP contribute to ECM deterioration, along with a direct effect of UV radiation and reactive oxygen species.44,45 However, in our 3D tissue model, cells were cultured in a freshly made ECM gel and, although mechanical rearrangement was evident, we found no indication of ECM degradation. Masson's trichrome staining revealed increased density and cluster size of collagen fibers in the vicinity of senescent fibroblasts, and SASP inhibition did not prevent this change, suggesting that ECM remodeling rather than degradation occurred. Our results therefore suggest that senescent fibroblasts play a role in skin stiffening through excessive traction stress. Furthermore, because angiogenic sprouts exhibit a Gaussian-like response to ECM stiffness—ECM that is too soft or too stiff prevents and inhibits sprout expansion, respectively46,47—it is reasonable to hypothesize that senescent fibroblasts can sustain angiogenesis through an appropriate stiffening of ECM due to the morphogenic changes. It would be useful to determine whether senescent fibroblasts cause a vascular endothelial dysfunction, by inducing an excessive angiogenesis and ECM stiffening, that eventually contribute to the reduction in capillaries in aged skin.
Finally, we used rapamycin as a SASP inhibitor to validate whether the angiogenesis was due to the SASP. In recent years, senotherapeutics—treatments that aim at preventing senescence, limiting its effect, or removing senescent cells—have been demonstrated as an effective strategy to reduce aging-associated disorders.48 Our model may therefore prove useful in developing such senotherapeutics.
5. Conclusions
We investigated the changes experienced by blood vessels in aged skin by creating a simple dermis-on-a-chip model. This model comprised an in vitro 3D model of a blood vessel embedded in a collagen-type-I ECM with young and/or senescent skin fibroblasts. This simple setting, free of the influences of other dermal cells such as neurons or immune cells, permitted the monitoring of effects due only to aged fibroblasts, which are a main player in dermis aging. We found that senescent fibroblasts activate angiogenesis through their SASP and tend to show excessive traction stress associated with ECM's morphogenic changes, contributing eventually to angiogenesis. Further exploration of the consequences of this angiogenesis and the fate of blood vessels in aged skin, as well as the underlying molecular and mechanical mechanisms, may lead to the development of new strategies for age-related skin disorders. However, we demonstrated that a 3D model can be used to develop drugs that target senescence. The model's versatility, based on using fibroblasts and endothelial cells originating from other tissues than skin and tuning the number of senescent cells, make it a powerful tool for the comprehensive study of the effects of senescence cells in a tissue.
Funding
This research was partly supported by MEXT/JSPS KAKENHI to Y. T. M. (grant number JP18H01793), to J. P. (JP18K14104), to R. U. (JP18J14488), to T. N. (JP19K16177), to M. N. (JP26250027, JP22118003, JP16K15239), to Y. J. (JP18H05026m, JP16H06148, JP16K15238), and by AMED to Y. T. M. (JP18cm0106239h0001, JP20cm0106272h0001) and M. N. (JP17cm0106122, JP17fk0310111, JP17gm5010001).
Conflicts of interest
There are no conflicts to declare.
Acknowledgements
The authors acknowledge Dr. Haruko Takahashi (The University of Tokyo, current: Hiroshima University) for fruitful discussions, and Eri Otsuka (The University of Tokyo), Keisuke Ito (Tokyo Metropolitan University), and Naoya Takakusaki (Tokyo Metropolitan University) for their technical assistance. The authors are also grateful to Takashi Ando for his help with illustrations, and Kayoko Suenaga (Carl Zeiss Microscopy) for her support with CRM imaging.
References
- M. Xu, T. Pirtskhalava, J. N. Farr, B. M. Weigand, A. K. Palmer and M. M. Weivoda,
et al., Senolytics improve physical function and increase lifespan in old age, Nat. Med., 2018, 24(8), 1246–1256 CrossRef CAS
. Available from: https://www.ncbi.nlm.nih.gov/pubmed/29988130.
- J. C. Jeyapalan, M. Ferreira, J. M. Sedivy and U. Herbig, Accumulation of senescent cells in mitotic tissue of aging primates, Mech. Ageing Dev., 2007, 128(1), 36–44 CrossRef CAS
. Available from: http://internal-pdf://122.109.141.60/(2007,_Jeyapalan)_Accumulation_of_senescent_ce.pdf.
- R. M. Naylor, D. J. Baker and J. M. van Deursen, Senescent Cells: A Novel Therapeutic Target for Aging and Age-Related Diseases, Clin. Pharmacol. Ther., 2013, 93(1), 105–116 CrossRef CAS
. Available from: http://doi.wiley.com/10.1038/clpt.2012.193.
- D. McHugh and J. Gil, Senescence and aging: Causes, consequences, and therapeutic avenues, J. Cell Biol., 2018, 217(1), 65–77 CrossRef CAS
. Available from: http://internal-pdf://196.11.173.252/(2017)_Senescence_and_aging_Causes,_consequenc.pdf.
- J. E. Yoon, Y. Kim, S. Kwon, M. Kim, Y. H. Kim and J. H. Kim,
et al., Senescent fibroblasts drive ageing pigmentation: A potential therapeutic target for senile lentigo, Theranostics, 2018, 8(17), 4620–4632 CrossRef CAS
. Available from: http://internal-pdf://0943947739/(2018,_Jung_Eun_Yoon)_Senescent_fibroblasts_dr.pdf.
- L. Hayflick, The limited in vitro lifetime of human diploid cell strains, Exp. Cell Res., 1965, 37(3), 614–636, DOI:10.1016/0014-4827(65)90211-9
.
- L. Hayflick and P. S. Moorhead, The serial cultivation of human diploid cell strains, Exp. Cell Res., 1961, 25(3), 585–621, DOI:10.1016/0014-4827(61)90192-6
.
- N. Herranz and J. Gil, Mechanisms and functions of cellular senescence, J. Clin. Invest., 2018, 128(4), 1238–1246 CrossRef
. Available from: http://internal-pdf://0719885386/(2018)_Mechanisms_and_functions_of_cellular_se.pdf.
- A. Hernandez-Segura, J. Nehme and M. Demaria, Hallmarks of Cellular Senescence, Trends Cell Biol., 2018, 28(6), 436–453 CrossRef CAS
. Available from: http://internal-pdf://228.60.152.105/(2018)_Hallmarks_of_Cellular_Senescence.pdf.
- S. Lopes-Paciencia, E. Saint-Germain, M. C. Rowell, A. F. Ruiz, P. Kalegari and G. Ferbeyre, The senescence-associated secretory phenotype and its regulation, Cytokine, 2019, 117, 15–22 CrossRef CAS
. Available from: http://internal-pdf://65.167.196.201/(2019,_Cytokine,_Review)_The senescence-associ.pdf.
- J. P. Coppe, P. Y. Desprez, A. Krtolica and J. Campisi, The senescence-associated secretory phenotype: the dark side of tumor suppression, Annu. Rev. Pathol.: Mech. Dis., 2010, 5, 99–118 CrossRef CAS
. Available from: http://internal-pdf://121.226.169.227/(2010, Review)_The_Senescence-Associated_Secre.pdf.
- K. A. Beningo, K. Hamao, M. Dembo, Y. L. Wang and H. Hosoya, Traction forces of fibroblasts are regulated by the Rho-dependent kinase but not by the myosin light chain kinase, Arch. Biochem. Biophys., 2006, 456(2), 224–231 CrossRef CAS
. Available from: http://internal-pdf://6.103.137.119/(2006,_Beningo_KA)_Traction_forces_of_fibrobla.pdf.
- K. Ghosh and B. C. Capell, The Senescence-Associated Secretory Phenotype: Critical Effector in Skin Cancer and Aging, J. Invest. Dermatol., 2016, 136(11), 2133–2139 CrossRef CAS
. Available from: http://internal-pdf://0304089172/(2016,_Review)_The Senescence-Associated_Secre.pdf.
- J. P. Coppe, K. Kauser, J. Campisi and C. M. Beausejour, Secretion of vascular endothelial growth factor by primary human fibroblasts at senescence, J. Biol. Chem., 2006, 281(40), 29568–29574 CrossRef CAS
. Available from: http://internal-pdf://189.27.88.186/(2006)_Secretion_of_VEGF_by_Primary_Human_Fibr.pdf.
- M. Nakanishi, G. R. Adami, R. S. Robetorye, A. Noda, S. F. Venable and D. Dimitrov,
et al., Exit from G0 and entry into the cell cycle of cells expressing p21Sdi1 antisense RNA, Proc. Natl. Acad. Sci. U. S. A., 1995, 92(10), 4352–4356 CrossRef CAS
. Available from: http://internal-pdf://121.161.222.170/(1995,_Nakanishi M)_Exit_from_G0_and_entry_int.pdf.
- B. Sabass, M. L. Gardel, C. M. Waterman and U. S. Schwarz, High resolution traction force microscopy based on experimental and computational advances, Biophys. J., 2008, 94(1), 207–220 CrossRef CAS
. Available from: http://internal-pdf://100.155.183.124/(2008,_Sabass)_High_Resolution_Traction_Force.pdf.
- S. V. Plotnikov, B. Sabass, U. S. Schwarz and C. M. Waterman, High-resolution traction force microscopy, Methods Cell Biol., 2014, 123, 367–394 Search PubMed
. Available from: http://internal-pdf://82.14.237.209/(2014,_Sergey_V)_High-Resolution_Traction_Forc.pdf.
- Q. Tseng, E. Duchemin-Pelletier, A. Deshiere, M. Balland, H. Guillou and O. Filhol,
et al., Spatial organization of the extracellular matrix regulates cell-cell junction positioning, Proc. Natl. Acad. Sci. U. S. A., 2012, 109(5), 1506–1511 CrossRef CAS
. Available from: http://www.ncbi.nlm.nih.gov/pubmed/22307605.
- J. Pauty, R. Usuba, I. G. Cheng, L. Hespel, H. Takahashi and K. Kato,
et al., A Vascular Endothelial Growth Factor-Dependent Sprouting Angiogenesis Assay Based on an In Vitro Human Blood Vessel Model for the Study of Anti-Angiogenic Drugs, EBioMedicine, 2018, 27, 225–236, DOI:10.1016/j.ebiom.2017.12.014
, https://www.thelancet.com/journals/ebiom/article/PIIS2352-3964(17)30497-8/fulltext.
- J. Pauty, R. Usuba, H. Takahashi, J. Suehiro, K. Fujisawa and K. Yano,
et al., A vascular permeability assay using an in vitro human microvessel model mimicking the inflammatory condition, Nanotheranostics, 2017, 1(1), 103–113, DOI:10.7150/ntno.18303
, http://www.ntno.org/v01p0103.htm .
- J. Tigges, J. Krutmann, E. Fritsche, J. Haendeler, H. Schaal and J. W. Fischer,
et al., The hallmarks of fibroblast ageing, Mech. Ageing Dev., 2014, 138, 26–44 CrossRef CAS
. Available from: https://www.ncbi.nlm.nih.gov/pubmed/24686308.
- N. Herranz, S. Gallage, M. Mellone, T. Wuestefeld, S. Klotz and C. J. Hanley,
et al., mTOR regulates MAPKAPK2 translation to control the senescence-associated secretory phenotype, Nat. Cell Biol., 2015, 17(9), 1205–1217, DOI:10.1038/ncb3225
.
- S. Hubackova, K. Krejcikova, J. Bartek and Z. Hodny, IL1- and TGFbeta-Nox4 signaling, oxidative stress and DNA damage response are shared features of replicative, oncogene-induced, and drug-induced paracrine “bystander senescence”, Aging, 2012, 4(12), 932–951 CrossRef CAS
. Available from: http://internal-pdf://149.181.175.92/(2012,Hubackova)_IL1-_and_TGFβ-Nox4_signaling.pdf.
- G. Nelson, J. Wordsworth, C. Wang, D. Jurk, C. Lawless and C. Martin-Ruiz,
et al., A senescent cell bystander effect: senescence-induced senescence, Aging Cell, 2012, 11(2), 345–349 CrossRef CAS
. Available from: http://internal-pdf://152.1.21.190/(2012,_Nelson)_A senescent_cell_bystander_effe.pdf.
- J. C. Acosta, A. O'Loghlen, A. Banito, M. V. Guijarro, A. Augert and S. Raguz,
et al., Chemokine signaling via the CXCR2 receptor reinforces senescence, Cell, 2008, 133(6), 1006–1018 CrossRef CAS
. Available from: http://internal-pdf://191.106.182.15/(2008,Acosta JC)_Chemokine_signaling_via_the_C.pdf.
- S. Ressler, J. Bartkova, H. Niederegger, J. Bartek, K. Scharffetter-Kochanek and P. Jansen-Durr,
et al., p16INK4A is a robust in vivo biomarker of cellular aging in human skin, Aging Cell, 2006, 5(5), 379–389 CrossRef CAS
. Available from: http://internal-pdf://157.78.212.59/(2006,_Ressler)_p16INK4A_is_a_robust_in_vivo_b.pdf.
- M. E. Waaijer, W. E. Parish, B. H. Strongitharm, D. van Heemst, P. E. Slagboom and A. J. de Craen,
et al., The number of p16INK4a positive cells in human skin reflects biological age, Aging Cell, 2012, 11(4), 722–725 CrossRef CAS
. Available from: http://internal-pdf://0945117276/(2012,_Waaijer)_The_number_of_p16INK4a_positiv.pdf.
- T. Ezure, M. Sugahara and S. Amano, Senescent dermal fibroblasts negatively influence fibroblast extracellular matrix-related gene expression partly via secretion of complement factor D, BioFactors, 2019, 45(4), 556–562 CrossRef CAS
. Available from: http://internal-pdf://215.148.120.254/(2019,_Tomonobu_Ezure)_Senescent_dermal_fibrob.pdf.
- I. Lammermann, L. Terlecki-Zaniewicz, R. Weinmullner, M. Schosserer, H. Dellago and A. D. de Matos Branco,
et al., Blocking negative effects of senescence in human skin fibroblasts with a plant extract, npj Aging Mech. Dis., 2018, 4, 4 CrossRef
. Available from: http://internal-pdf://247.36.198.126/(2018)_Blocking_negative_effects_of_senescence.pdf.
- T. M. Cheung, J. B. Yan, J. J. Fu, J. Huang, F. Yuan and G. A. Truskey, Endothelial Cell Senescence Increases Traction Forces due to Age-Associated Changes in the Glycocalyx and SIRT1, Cell. Mol. Bioeng., 2015, 8(1), 63–75 CrossRef CAS
. Available from: http://internal-pdf://67.83.208.205/(2015)_Endothelial_Cell_Senescence_Increases_T.pdf.
- L. Yao, M. J. Romero, H. A. Toque, G. Yang, R. B. Caldwell and R. W. Caldwell, The role of RhoA/Rho kinase pathway in endothelial dysfunction, J. Cardiovasc. Dis. Res., 2010, 1(4), 165–170 CrossRef
. Available from: http://internal-pdf://0628175011/(2010)_The_role_of_RhoA-Rho_kinase_pathway_in.pdf.
- A. K. Harris, D. Stopak and P. Wild, Fibroblast traction as a mechanism for collagen morphogenesis, Nature, 1981, 290(5803), 249–251 CrossRef CAS
. Available from: https://www.ncbi.nlm.nih.gov/pubmed/7207616.
- H. Y. Chang, J. T. Chi, S. Dudoit, C. Bondre, M. Van De Rijn and D. Botstein,
et al., Diversity, topographic differentiation, and positional memory in human fibroblasts, Proc. Natl. Acad. Sci. U. S. A., 2002, 99(20), 12877–12882 CrossRef CAS
. Available from: http://pmc/articles/PMC130553/?report=abstract.
- S. Kim, H. Lee, M. Chung and N. L. Jeon, Engineering of functional, perfusable 3D microvascular networks on a chip, Lab Chip, 2013, 13(8), 1489–1500 RSC
. Available from: http://www.rsc.org/loc.
- D. H. T. Nguyen, S. C. Stapleton, M. T. Yang, S. S. Cha, C. K. Choi and P. A. Galie,
et al., Biomimetic model to reconstitute angiogenic sprouting morphogenesis in vitro, Proc. Natl. Acad. Sci. U. S. A., 2013, 110(17), 6712–6717 CrossRef CAS
. Available from: https://pubmed.ncbi.nlm.nih.gov/23569284/.
- B. Trappmann, B. M. Baker, W. J. Polacheck, C. K. Choi, J. A. Burdick and C. S. Chen, Matrix degradability controls multicellularity of 3D cell migration, Nat. Commun., 2017, 8(1), 371, DOI:10.1038/s41467-017-00418-6
. Available from: http://pmc/articles/PMC5575316/?report=abstract.
- B. M. Baker, B. Trappmann, S. C. Stapleton, E. Toro and C. S. Chen, Microfluidics embedded within extracellular matrix to define vascular architectures and pattern diffusive gradients, Lab Chip, 2013, 13(16), 3246–3252 RSC
. Available from: http://pmc/articles/PMC4082768/?report=abstract.
- A. C. Newman, M. N. Nakatsu, W. Chou, P. D. Gershon and C. C. W. Hughes, The requirement for fibroblasts in angiogenesis: Fibroblast-derived matrix proteins are essential for endothelial cell lumen formation, Mol. Biol. Cell, 2011, 22(20), 3791–3800 CrossRef CAS
. Available from: http://pmc/articles/PMC3192859/?report=abstract.
- K. Yano, K. Kajiya, M. Ishiwata, Y. K. Hong, T. Miyakawa and M. Detmar, Ultraviolet B-Induced Skin Angiogenesis Is Associated with a Switch in the Balance of Vascular Endothelial Growth Factor and Thrombospondin-1 Expression, J. Invest. Dermatol., 2004, 122(1), 201–208, DOI:10.1046/j.0022-202X.2003.22101.x
.
- D. Výbohová, Y. Mellová, K. Adamicová, M. Adamkov and G. Hešková, Quantitative changes of the capillary bed in aging human skin, Histol. Histopathol., 2012, 27(7), 961–967, DOI:10.14670/HH-27.961
.
- K. Kajiya, Y. K. Kim, Y. Kinemura, J. Kishimoto and J. H. Chung, Structural alterations of the cutaneous vasculature in aged and in photoaged human skin in vivo, J. Dermatol. Sci., 2011, 61, 206–208, DOI:10.1016/j.jdermsci.2010.12.005
.
- A. G. Gunin, V. V. Petrov, N. N. Golubtzova, O. V. Vasilieva and N. K. Kornilova, Age-related changes in angiogenesis in human dermis, Exp. Gerontol., 2014, 55, 143–151 CrossRef CAS
. Available from: http://internal-pdf://243.123.141.220/(2014)_Age-related_changes_in_angiogenesis_in.pdf.
- J. K. Kular, S. Basu and R. I. Sharma, The extracellular matrix: Structure, composition, age-related differences, tools for analysis and applications for tissue engineering, J. Tissue Eng., 2014, 5, 2041731414557112 Search PubMed
. Available from: http://internal-pdf://176.6.200.62/(2014)_ECM_review.pdf.
- J. M. Phillip, I. Aifuwa, J. Walston and D. Wirtz, The Mechanobiology of Aging, Annu. Rev. Biomed. Eng., 2015, 17, 113–141 CrossRef CAS
. Available from: http://internal-pdf://241.243.36.84/(2016)_The_Mechanobiology_of_Aging.pdf.
- M. J. Sherratt, Age-Related Tissue Stiffening: Cause and Effect, Adv. Wound Care, 2013, 2(1), 11–17 CrossRef
. Available from: http://internal-pdf://103.99.132.94/(2013)_Age-Related_Tissue_Stiffening_Cause_and.pdf.
- L. T. Edgar, C. J. Underwood, J. E. Guilkey, J. B. Hoying and J. A. Weiss, Extracellular matrix density regulates the rate of neovessel growth and branching in sprouting angiogenesis, PLoS One, 2014, 9(1), e85178 CrossRef
. Available from: http://internal-pdf://147.129.130.58/(2014,_LT Edgar)_Extracellular_matrix_density.pdf.
- C. O. Crosby and J. Zoldan, Mimicking the physical cues of the ECM in angiogenic biomaterials, Regener. Biomater., 2019, 6(2), 61–73 CrossRef CAS
. Available from: http://internal-pdf://81.234.211.107/(2019)_Mimicking_the_physical_cues_of_the_ECM.pdf.
- Y. Ovadya and V. Krizhanovsky, Strategies targeting cellular senescence, J. Clin. Invest., 2018, 128, 1247–1254, DOI:10.1172/JCI95149
.
Footnote |
† Electronic supplementary information (ESI) available. See DOI: 10.1039/d0bm01297a |
|
This journal is © The Royal Society of Chemistry 2021 |