DOI:
10.1039/D0BM01209B
(Review Article)
Biomater. Sci., 2020,
8, 6469-6504
The progress in corneal translational medicine
Received
20th July 2020
, Accepted 12th October 2020
First published on 11th November 2020
Abstract
Cornea tissue is in high demand by tissue donation centres globally, and thus tissue engineering cornea, which is the main topic of corneal translational medicine, can serve as a limitless alternative to a donated human cornea tissue. Tissue engineering aims to produce solutions to the challenges associated with conventional cornea tissue, including transplantation and use of human amniotic membrane (HAM), which have issues with storage and immune rejection in patients. Accordingly, by carefully selecting biomaterials and fabrication methods to produce these therapeutic tissues, the demand for cornea tissue can be met, with an improved healing outcome for recipients with less associated harmful risks. In this review paper, we aim to present the recent advancements in the research and clinical applications of cornea tissue, applications including biomaterial selection, fabrication methods, scaffold structure, cellular response to these scaffolds, and future advancements of these techniques.
1. Introduction
The eye is an important organ in the human body, which provides vision, allowing the environment to be presented as a visual image for humans to respond to. Furthermore, 65% of the nerve pathways to the brain are used by the eye, indicating how important vision is to human life.1 The cornea plays a crucial role in protecting the eye and aids in concentrating human vision by letting light into the eye.2 This protective role for the eye is shared by tears, the sclera, the eyelids and the eye sockets, all simultaneously functioning to prevent the entry of harmful particles. The cornea acts as an outermost layer of the eye, which protects the internal parts of the eye and the lens and retina from ultraviolet light damage.
Tissue engineered cornea is produced by fabricating a scaffold from appropriately selected biomaterials, which is then seeded with cells from different sources. These cells differentiate into specific corneal structures, and once sterilised, this construct can then be implanted to replace a defected cornea. Once implanted, the scaffold acts as a temporary substitute extracellular matrix (ECM) until the seeded cells generate a confluent tissue that can function naturally.
With the increase in the aging population, people are living longer, and thus organs and tissue are expected to last longer and continue functioning throughout their lives. Accordingly, replacing diseased, aged and damaged organs will increase the pressure on tissue banks as the population continues to grow.3 Consequently, engineered tissue can become an untapped supply for surgeons to access whenever necessary, and together with keratoplasty, which is a common surgery, it can vastly reduce the strain on tissue banks and lower immunological rejection rates. Furthermore, the time that a patient will have to wait for transplantation will be significantly reduced since as the element of waiting for a donor organ is removed entirely with multiple synthetic corneas made from one donation of cells, and surgeons will be able to simply retrieve a tissue engineered organ from a vast storage supply.4
2. Cornea anatomy and physiology
The cornea, which is found on the outermost layer of the eye (as shown in Fig. 1), is an avascular connective tissue serving as a transparent protective barrier to the eyeball, while acting as the refractive surface for the eye to enable vision, making up a sixth of the anterior spheroid.5,6 The human cornea can be found anterior to the anterior chamber of the eye in front of the pupil, iris and aqueous humour, lying continuously with the sclera and conjunctiva,7 where the only structure lying superficial to the cornea is the tear film. A reservoir of pluripotent stem cells is located in the limbus, the area where the cornea meets the sclera.7 The cornea is made up of five layers, from the outermost layer to the innermost layer as follows: the epithelium layer, Bowman's layer, the stroma, Descemet's membrane and the endothelium, as shown in Fig. 2. However, the cornea consists of only 3 cellular layers, which are the epithelium, stroma and endothelium layers. Each layer serves a function, collectively working together to allow the refraction of light rays alongside the lens, allowing them to focus on the retina to provide eyesight. Hydration of the eyes is achieved anteriorly through tears and by the aqueous humor in the posterior section of the cornea.8 The acellular non-regenerative layers are made up of collagen layers, which still have some unknown importance to the function of the eye besides maintaining the cornea shape; however, if damage occurs to these layers, opaque scar formation can negatively affect vision.2,8
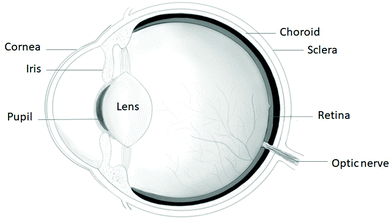 |
| Fig. 1 Anatomy of the cornea in relation to the eye (figure belongs to the authors). | |
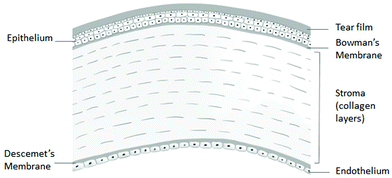 |
| Fig. 2 Layers of the cornea (reproduced from ref. 9 with permission from Elsevier, Copyright 2019).9 | |
2.1 The epithelium layer
The epithelium layer, which has a thickness of roughly 5–7 cells, makes up around 10% of the entire corneal density. The primary role of the epithelium layer is to allow the even spread of moisture to the surface of the cornea via cooperation with the tear film and smoothness of its surface.2 Similar to other epithelial tissue found in the body, it serves to control the entry of solutes and water through diffusion and allows evaporation from its surface to dehydrate the cornea.8 The corneal epithelium layer contains non-keratinized, stratified squamous epithelium, and the top layers of these cells appear as squashed polyhedral cells, which begin to appear rounder and then longer deeper in the epithelium. The first 2–4 layers of the epithelium are known as the surface squamous cells or superficial cell, and beneath these cells are 1–3 layers of wing cells. The next layer is a single layer of basal cells, and the basement membrane lies at the bottom of the epithelium layer, as shown in Fig. 3. The superficial cells, which contain microvilli and microplicae on their surface, are coated by a layer of glycocalyceal bodies, increasing the surface area to increase tear film attachment and oxygen absorption.5,8
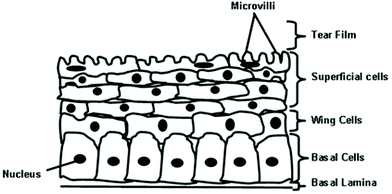 |
| Fig. 3 Epithelial layer of the cornea, including the tear film, surface cells, wing cells, basal cells and basement membrane cells (figure created by the authors). | |
2.2 The stroma layer
The stroma is located posterior to Bowman's layer, which is largely made up of collagen fibers and water, enforcing the corneal strength, while simultaneously maintaining the contour and transparency of the cornea.6 The collagen fibers are equal in size and appear as an organised structure parallel to the cornea surface. These collagen fibers, which are oriented parallel to each other, are known as fibrils and form a lamellae structure, thus giving the cornea its shape and birefractive property.2,10 If the cornea swells significantly, the uniform spacing of the collagen fibers will be disrupted, distorting vision due to light scattering.7 The stroma is the largest layer found within the cornea, making up approximately 90% of the cornea with a thickness of approximately 0.5 mm,2 and thus is responsible for strengthening the cornea. To preserve the shape of the cornea without deformation due to various forces from the eye, the collagen fibrils within the stroma form the load-bearing section of the lamellae. Furthermore, as the main strength of the stroma, these collagen fibrils must appear thin and long to enable the cornea to be transparent without impeding structures.10
2.3 The endothelium layer
The endothelium consists of one layer of hexagonal or polygonal cells, which is only 5 μm thick.2 The endothelium controls the transparency of the cornea by limiting the entry of metabolic factors,11 while maintaining an osmotic gradient to keep the cornea dehydrated adequately.5 Endothelial cells prevent the cornea from becoming unclear and opaque, where the endothelium pumps a surplus of fluid away from the stroma.4 Na+/K+-Adenosine triphosphate (ATP)-ase and other active transport pumps can be used to move solutes into the endothelium from the aqueous humor.8 Anteriorly, the endothelium attaches Descemet's layer, whilst the posterior side possesses microvilli, which protrude into the aqueous humour to increase its surface area.7 When corneal endothelium cells (CEnCs) are destroyed, they do not regenerate in vivo in humans and have a set proliferative ability in vivo,12 which is due to the absence of the G1 phase during cell division.11
2.4 Impact of limbus niche
The activity of human body stem cells in any biological system needs to be strongly regulated to guarantee that the function of a tissue or organ remains stable. Accordingly, the extracellular matrix and cell/tissue niche (microenvironment) in which stem cells reside play an important role in regulating the fate of stem cells.13 Consequently, the stem cell niche is an extremely specialised, anatomically distinct zone of a tissue, which provides suitable micro-environmental signals essential to conserve a sub-population of cells that can participate in the regenerative demands of a tissue at any given time. The precise biological mechanisms such as soluble biochemical factors, mechanical cues and metabolic factors, and cell-contact-dependent signals by which the niche regulates stem cell behaviour have only recently started to be elucidated. Thus, the human limbus ring is an area of significant interest with regards to recognising the niche components that regulate corneal epithelial stem cells. In the human ocular surface, there are some structures of the limbus that separate it from both the conjunctiva and the cornea. The stromal layer in the limbus forms papillae-like invaginations known as the ‘palisades of Vogt’, in between which are limbal epithelial crypts. Within these palisades of Vogt structures, a sub-fraction of basal epithelial cells expresses some stem cell markers, such as canexin-43, Δp63a, Fzd7, N-cadherin, ABCG2, and cytokeratin 19, which is consistent with the notion that the limbus provides a specific stromal micro-environment that is responsible for maintaining and supporting corneal epithelial stem cells.14 Also, the limbus stromal tissue is greatly vascularised and contains distinct extra cellular matrix components such as α1 and α2 collagen IV, β2 laminin and vitronectin compared to the corneal stroma, all of which may play a critical role in maintaining corneal epithelial stem cells. Consequently, there is corresponding evidence that direct biomechanical interactions between the limbus stromal mesenchymal cells and epithelial cells in the palisades of Vogt are essential in maintaining the stemness property of the stem cell population.15 Due to the dome-like topography of the human cornea, the distribution of mechanical force at different corneal locations is variable, which may favour stem cell maintenance at specific locations.16
3. Common diseases associated with the cornea
Conservation of a healthy cornea is critical to maintain the refractive and transparent properties imperative to enabling eyesight. This involves the cooperation of the layers of the cornea, which regulate dehydration and the cells working together, and if these processes are not carried out correctly, it can lead to corneal defects.8 Cataracts is the most common cause of blindness worldwide, followed by cornea-related diseases, where in 2005, it was estimated that 10–15 million people were affected by blindness due to cornea issues.17
3.1 Corneal trauma
Trauma to the cornea can cause a decline in vision due to deep surface injury and resultant dehydration of the local tissue.18 Trauma can be in the form of burns (such as chemical, electrical and thermal) or from abrasion (physical contact or foreign bodies entering the eye).19 Corneal transplantation is often required since despite the swift treatment of burns, scarring can cause blindness. However, corneal transplantation is often ineffective in the case of alkali burns, with transparency being reduced with time because of the reduction in limbal epithelial stem cells.20
3.2 Corneal edema
The endothelium layer controls and maintains the fluid content in the eye, and thus if this cornea membrane is damaged, oedema can form due to excess fluid retention, resulting in a reduction in.2 Similarly, if the epithelium layer is impaired, leakage into the stroma from the tear fluid can occur.7 This can become critical if chronic oedema occurs, whereby the number of CEnC number can become so low that the stroma deturgescence functionality fails and the cornea swells.
3.3 Corneal dystrophies
Corneal dystrophies commonly present as deposits within the cornea, which is usually inherited but can be caused by gene mutation occurring at any point in life, potentially in any of the layers of the cornea. This can usually be diagnosed and analysed using retroillumination, with it presenting as cysts, clouding, scarring or opacities. Symptoms include photophobia, irritation, vision disturbance, reduced sensitivity and pain.19,21 Dystrophies can cause astigmatisms, oedema, or erosion, leading eventually to a reduction in the quality of vision.8
3.4 Dry eyes
Dry eye (also known as hypolacrimation syndrome or keratoconjunctivitis sicca) can be caused by malfunction or deficiency of one or more layers of the tear film. This can lead to pitting on the surface of the corneal epithelium, reducing the smoothness of the surface, resulting in cloudy or hazy vision.7 Dry eye can occur due to multiple conditions, including issues as severe as collagen vascular diseases and conjunctival scarring to Vitamin A deficiency or drug side effects.19
Dry eye can be one of two types, either evaporative dry eye or aqueous tear-deficient dry eye.
3.5 Corneal ulcers
Corneal ulcers are rare but severe cornea sores that can cause blindness, which appear as opaque shapes over the eye surface and occur due to various reasons such as infection, including viral, fungal and parasitic, contact lens use, trauma and dryness. Symptoms can include ocular pain, swelling and increased cornea sensitivity, which can be identified by corneal scraping or tandem scanning confocal microscopy.8
3.6 Corneal development anomalies
Corneal development anomalies are usually congenital and present in paediatric cases, where this type of cornea defect regularly reduces the vision in patients and can sometimes be severe enough to cause blindness. The three most common anomalies are megalocornea (larger than the normal horizontal diameter of the cornea, which can cause nearsightedness), buphthalmos (stretched/oversized cornea causing nearsightedness) and microcornea (smaller than normal cornea causing farsightedness).19
3.7 Corneal degenerations
Corneal degeneration can be age related, hereditary or sporadic, usually occurring later in life, and regardless of in one eye or both, this degeneration rarely presents any symptoms in its patients, but can cause vision disturbances. Degeneration of the cornea can appear as opacities within the cornea layers, deposits, cornea thinning, lesions, astigmatism or nodules.19 Some examples of corneal degenerations include crocodile shagreen, limbal girdle of Vogt, arcus senilis, calcific band keratopathy, Salzmann's nodular degeneration, Terrien's marginal degeneration, polymorphic amyloid degeneration and iridocorneal endothelial syndrome.
3.8 Corneal infections
Microbial keratitis is a common corneal disease resulting from surgery, corneal damage, disease exposure and wearing contact lenses. Keratitis is a category of cornea defects, whereby the cornea becomes inflamed and can cause visual impairment or pain. Issues can arise with the cornea because of contact lens wear for a variety of reasons. Contact lens can prevent proper oxygen circulation and cause drying of the eyes or hypoxia, the solutions used in the lenses may cause an allergic reaction or toxic effect within the eye, and the action of mechanically putting the lens in and taking them out can cause abrasion to the surface. Complications of microbial keratitis can result in epithelial microcysts, stromal oedema and punctate staining.22 Infections can be bacterial, protozoa, fungal or viral and may require a biopsy to diagnose the cause of photophobia, discharge or pain.23
3.9 Corneal inflammatory disorders
Corneal inflammatory disorders are caused by autoimmune conditions, which can be either infectious or non-infectious, caused by trauma or different types of infective agents. These disorders can cause blindness, and in severe cases can be potentially life threatening, with some cases being uncurable, where treatment only reduces the symptoms. Symptoms include inflammation, fluid seepage, styes, debris, eye irritation, pits, excess vascularisation, pain, filament attachment, and photophobia.8,22 Some examples of these inflammatory corneal disorders include chalazion, ocular rosacea, phlyctenulosis, Thygeson's keratitis and blepharitis.
3.10 Metabolic disorders
Patients with metabolic disorders are at higher risk of developing cornea diseases, which can lead to a reduction in corneal transparency. These diseases can be triggered by unhealthy lifestyle, age, heart disease, and hereditary conditions, such as enzyme defects, which can appear over the entirety of the cornea, possibly on multiple layers. The progression of this disorder can lead to fluid build-up and eventually glaucoma, which is an eye condition where the optic nerve is damaged and can worsen if not treated, leading to vision loss. Metabolic disorders affecting the eye present as opacities on the cornea, thinned cornea and discoloration of eye components. Disorders that affect the cornea include but are not limited to Peter's anomaly, lipid metabolism disorders, Axenfeld's anomaly, osteogenesis imperfecta and Wilson's disease.8,19
3.11 Systemic and immunological disorders
Cornea diseases and defects presented due to systemic disorders may be caused by various conditions such as autoimmune diseases or dermatitis, where most patients with these cornea issues are asymptomatic; however, regular eye tests are recommended to detect these problems, which left untreated, can worsen and reduce vision. Diseases that can affect vision by altering the cornea include multiple myeloma, Mooren's ulceration, rheumatoid melt and Stevens-Johnson syndrome. They can cause corneal ulcerations, ocular depositions, abnormal vascularisation, lesions and general corneal discomfort.7,19
3.12 Corneal curvature defects
Cornea curvature defects are one of the most clinically diagnosed issues by opticians, which normally require glasses or contact lens prescription to correct a patient's eyesight. Thy occur when the curvature of the cornea is not globular, and hence long or short sight vision may be disturbed. The cornea may be enlarged, thin, have astigmatisms or have an unusual shape, which can cause pain, vision decline, or oedema. Examples of the effects of corneal curvature include corneal ectasia, keratoconus and keratoglobus.8,19,21
4. Common treatments for corneal defects and diseases
With cornea diseases being the second most common reason for visual impairment, solutions to correct damaged cornea are limited but highly sought after. Superficial damage to the cornea can usually be repaired throughout the epithelium layer; however, injury progression into deeper layers can develop into more permanent deterioration. The lower layers of the cornea, such as Bowman's membrane or the endothelium layer, do not have cell regeneration capability, and therefore disturbance of these layers can cause corneal defects. An example of this is shown in the endothelium layer, where loss of endothelial cells from diseases such as Fuchs’ endothelial dystrophy or endotheliitis can result in oedema and distort vision because of swelling.
4.1 Antibiotics
Antibiotics can be used to treat bacterial keratitis, relieving the patient of some symptoms and reducing corneal inflammation.8 Antibiotics such as tetracycline can be used for severe infections, and if required the dose can be reduced for extended use.19
4.2 Artificial tears
Artificial tears are the primary treatment for dry eye conditions, which enable lubrication and protection of the anterior cornea and tear film. For severe dry eye, high viscosity artificial tears are recommended; however, they can cause blurring, and thus are better to use at night.24
4.3 Steroids
Corticosteroid creams can be used to treat some corneal infections; however, they have been known to reduce the immune responses of patients, and thus make other infections easier to infiltrate the body's defences.8 Steroids can also supress inflammatory reactions and can reduce observable inflammations, making it more difficult to observe more severe problems.22 Cataract formation is a known side effect of corticosteroids, together with increased ocular pressure, and thus despite their effectiveness in treating dry eye, they are not highly recommended in most cases.24
4.4 Amniotic membrane transplantation
Allografts of amniotic membrane (AM) are the gold standard for the treatment of defects of intractable epithelial, thermal and chemical burns, corneal ulcers, limbal cell deficiencies, and Stevens-Johnson syndrome. However, despite the attractive bioactivity and biocompatibility of AM, their mechanical strength is low, which may prevent urologists from using AM for supporting urinary tracts. Augmentation of AM with nanofibers is a hopeful strategy to produce novel bio-composites with attractive specifications that meet the requirements for regenerative urology. This novel bio composite made of AM and PLCL (poly(L-lactide-co-caprolactone)) nanofibers has been applied to replace the bladder wall after regional cystectomy. Gune et al. showed that covering a regenerated section of the urethra after mucosa graft urethroplasty may prevent fistula formation.25 Biotechnology suggests strategies to enrich bio-composites for tissue regeneration in order to manage their bioactivity and biocompatibility. HAM transplantation is used as a carrier for cell expansion, repairing the cornea by encouraging cell renewal; however, because this treatment involves allogenic biological material, it poses transmission risks from the donor to the patient and requires screening.
4.5 Keratoplasty
Keratoplasty, commonly referred to as corneal transplant surgery, involves removing an area of affected cornea from a patient and replacing it with healthy cornea tissue from a donor. This can involve replacing either the entire cornea or specifically damaged layer(s) of the cornea. Corneal endothelial deficiency may be treated with Descemet stripping with automated endothelial keratoplasty (DSAEK). DSAEK involves replacing the endothelial layer of the cornea, rather than the entire cornea (penetrating keratoplasty), which can lead to better results and fewer complications.4 Penetrating keratoplasty is the most invasive surgery, involving full-thickness corneal grafting, whereas lamellar keratoplasty involves partial-thickness transplantation, preserving healthy tissue and replacing defected tissue with corneal tissue from eye banks. There are two types of lamellar keratoplasty, namely anterior lamellar keratoplasty, which is the transplantation of the epithelium layer, Bowman's layer and the stroma, and posterior lamellar keratoplasty, which is the transplantation of Descemet's membrane, endothelium layer and potentially the posterior stroma.8
4.6 Corneal glue
In small defects less than 3 mm in the anterior cornea segment, tissue adhesives can be used to close perforations, defects and other open wound injuries.23 This procedure involves the denuding of tissue around the defect area, drying the area and then applying the adhesive, before covering the eye to keep the cornea clean. This treatment is quicker and less complicated than suturing, while reducing the chance of infection and providing an alternative to transplantation surgical attachment.8
4.7 Excimer laser phototherapeutic keratectomy (PTK)
When the cornea has issues with smoothness or clarity, keratectomy can be performed, which involves the removal of surface imperfections via surgery or use of a laser. After treatment, the patient should be given antibiotics and corticosteroid drops until re-epithelisation is complete and the surface has healed, preventing further infections.8 Excimer lasers (a type of ultraviolet laser) can be used during PTK, which remove opacities or abnormalities within the stroma layer of the cornea without affecting the epithelial layer. This treatment is carried out on defects such as frequent corneal erosion reoccurrence, scarring or nodules located superficially to the eye and anterior (to the stromal layer) corneal dystrophies.19
4.8 Refractive surgery
Interestingly, contact lenses have been adapted to not only correct impaired eyesight, but also to treat ocular surface diseases. These therapeutic contact lenses can be used to deliver drugs or reshape deformities with stiffened lenses.24 Where glasses cannot improve the vision of a patient with refractive error such as near-sightedness (myopia), far-sightedness (hyperopia) or an astigmatism, refractive surgeries are an option.
5. Justification for the need for tissue engineered cornea scaffolds
Human donated cornea tissue is highly sought after to enable surgeons to correct cornea defects, which can resolve vision difficulties, reduce pain and even help patients regain their sight. At the NHS Blood Transplant (NHSBT) eye banks in the UK, in September 2017, 79% of the required cornea donor tissue was available,26 which means that 21% of the patients requiring corneal transplants were unable to have surgery at that time. The demand for human corneal tissue will continuously increase because as the aging population increases, the strain on the tissues in the human body will increase, resulting in problems that require attention. Hence, it is necessary to create alternatives to keratoprosthetics and use of donor tissues. The need for cornea tissue engineering is not limited to the UK, where over 10 million individuals worldwide experience bilateral corneal blindness.27
6. Requirements of tissue engineered cornea
Undoubtedly, tissue engineering has become significantly important in the field of medicine, providing an alternative to prosthetics and donor tissue. To create a highly functional synthetic tissue that closely resembles native healthy tissue, there are some imperative determinants that can dictate the success of the tissue in vivo. Tissue engineered scaffolds serve as a framework for appropriate cells to be seeded to grow throughout the body. Thus, these scaffolds need to be made of appropriate biomaterials, and depending on the tissue desired to be synthetically replicated, their properties will be highly variable. In the case of tissue engineering corneal tissue, together with other factors, the materials chosen will need to possess the correct transparency, suitable strength, and sufficient degradation rates to match cell growth.
Transforming materials into scaffolds requires choosing beneficial methods, where the method is extremely crucial to ensuring the mechanical properties are adequate to prevent collapse of the scaffold, and bestowing a distribution of pores for tolerable porosity. Once a biodegradable scaffold has been produced, appropriate cells should be seeded into the scaffold, and then the entire structure should be placed into a bioreactor to expand the tissue encompassing the scaffold, making it ready for implantation.3
6.1 Biodegradability
The degradation rate of bioengineered scaffolds for the cornea is vital in ensuring that the scaffold degrades at a similar rate to the natural ECM and cell regeneration, where the scaffold mass loss should be proportional to the tissue growth rates.28 Early degradation can cause mechanical weakness of the new tissue and result in its failure, potentially further damaging the cornea. When a bioresorbable material is placed in the body, it begins to degrade, eventually breaking down via hydrolytic and/or enzyme mechanisms to be taken in by the blood stream or exhaled in the form of carbon dioxide depending on the material. Biodegradable scaffolds can be left in the body, not requiring removal after healing, reducing discomfort to the host.
The process of degradation begins once the scaffold is implanted in the body. Water ingresses into the biodegradable scaffold, cleaving the cross-links, side chains and backbone linkages between its units. This results in degradation from the centre of the polymer, degrading outwardly, sometimes causing ‘acid bursts’ of degradation products. Inflammatory responses can result due to acid release from the scaffold, more likely with bulk erosion degradation, depending on whether the scaffold has been neutralised and if the material is hydrophobic or hydrophilic.29 Hydrophilic scaffolds tend to increase the chance of inflammation, with ‘acid bursts’ being frequent, whereas hydrophobic scaffolds cause surface erosion, releasing the acidic by-products slower. Eventually the scaffold will be replaced entirely with new tissue, with the rate depending on various factors.
To control the degradation rate of scaffolds, there are various parameters that can be altered, which include altering bonds or functional groups, changing porosity, varying the crystallinity, copolymerising to adapt the hydrophilicity and changing the morphology of the scaffold.30
6.2 Biocompatibility
For synthetic cornea to be implanted into the body of an animal with no immunological rejection, it must be non-toxic and not cause adverse reactions in anyway, thus the biomaterial must be biocompatible. This should firstly be tested in vitro, screening the construct for cytotoxicity, hemocompatibility and genotoxicity. If in vitro testing is successful, with no incompatibility signs, then the cornea can be tested in vivo, on laboratory bred animals. In vitro tests involve acute systemic toxicity, subchronic toxicity, and implantation testing. This variety of testing ensures that the tissue engineered cornea does not cause damage or any adverse reactions to the body to varying degrees in vivo.31 After vigorous in vitro and in vivo analysis, then human trials can be considered.
Importantly, cellular reactions to foreign material implants can result in undesirable affects, and extrusion from the implant surface and ingestion from macrophages can occur. If the material is not biocompatible and acts as an irritant, inflammation can appear, resulting in reduced healing or cell growth, potentially leading to cell necrosis.32
Biocompatibility can be improved by changing the composition of the scaffold materials, where various components can be added to otherwise incompatible materials, creating biomaterials with a variety of useful properties.
6.3 Mechanical properties
Synthetic cornea must be able to ensure the stresses presented from both eye movement and intraocular pressures can be withstood, where blinking alone puts pressure on the cornea; therefore, resistance needs to be present. Mainly, the mechanical strength of the cornea and the sclera comes from the fibrous collagen arrangements.7 The mechanical properties of synthetic cornea can be altered by changing the material composition and the structure and layout of the material via fabrication methods. In our previous studies, for mechanical testing, PLGA membranes were cut into rectangles and a Bose Electroforce 3100 tensile test machine (Bose, MN, USA) was used to carry out mechanical tests on the membranes. Specifically, the membranes were clamped in place using stainless steel clamps and pulled apart at different rates.33,34
6.4 Cell attachment suitability
For creating a suitable environment for attachment on scaffolds, a few methods are available to encourage cell attachment. Crosslinking is an option to improve cell attachment, where previously collagen was crosslinked with glutaraldehyde (GA)35 or by carrying out dehydrothermal treatment.36 Ideally, the biomaterial should not result in cell death or create a toxic setting that can impact cell growth, which requires its chemical composition to be carefully considered. Also, for keratoplasty, once the type of keratoplasty is known, the next decision is to determine if it is crucial to culture cells in the scaffold prior to transplantation or to design the scaffold to allow the patient's own cells to repopulate it in vivo post-transplantation.37 Additionally, corneal endothelial cells have poor proliferative capacity, and thus implanting a biomaterial alone without cells will probably not be effective.38 On the other hand, for patients with limbal stem cell deficiencies, cells are required to repopulate the limbal region of the eye.39
6.5 Water permeability
To ensure visual clarity, the cornea needs to be suitably wet and permeable to create a balance between adhesion and cohesion of molecules. If the synthetic cornea becomes too wet, the scaffold may become less stiff and limp, but the cornea needs to have strength to prevent breakage from ocular pressures and environments. Excess water intake can also change the shape of the cornea or increase the degradation rate of the scaffold, reducing its refractive properties and resulting in weaker vision acuity; hence, a balance needs to be found. Sufficient hydrophilicity must be present in the scaffold to prevent dry eye and allow clear vision, and therefore treatment can take place after scaffold production to control water permeability, such as cross-linking or inclusions can be introduced to the biomaterials used to make the scaffold.
6.6 Transparency
Transparency is not only important to give clear vision, it also allows easier assessment of healing and examining for infection.11 Thus, it is important to preserve the functionality of the sodium and potassium ATP pumps within the endothelial layer since, as discussed previously, high transparency is the result of these pumps maintaining the equilibrium of the cornea.
Thicker scaffold fibers have been shown to reduce transparency; however, by decreasing the thickness of the fibers, the mechanical strength is compromised and reduced, and therefore strengthening of scaffolds must be carried out via other methods rather than increasing the fiber diameter.40 Additionally, the presence of collagen fibril free regions, which is caused by problems such as corneal oedema, can lead to visual distortions, where the larger these areas, the worse they are.41
Although the stroma may be deemed necessary to have aligned fibers throughout it, Bowman's layer displays irregular alignment, but still allows light transmission, and therefore it is suggested that collagen fibrils need to be evenly spaced and be thinner than the wavelength of light as a priority.41,42
6.7 Porosity
High porosity in scaffolds fabricated for application in cornea tissue engineering is extremely crucial in allowing the movement, attachment, proliferation and differentiation of cells.43 The greater the porosity of a scaffold, the higher the rate of nutrient transfer around the scaffold and cell infiltration into the pores due to the increased area where cells can move into and adhere to.44 The size of the pore can also determine the purpose of the pore, for example, a nano-sized pore will likely be filled when the ECM expands, whereas a macropore will have cells seeded within them and grow throughout.
A higher porosity can increase the surface area, thus increasing the degradation rate and enabling faster cell infiltration. Cell infiltration is an important factor in porous biomaterials, but the pore size is crucial since there is a risk of epithelial cells moving to the neighbouring stroma region, which can cause more complicated problems. A high surface area to volume ratio can enable greater fiber regulation and result in improved mechanical strength.43 The porosity of a scaffold can be determined via microscopy to estimate the pore size and distribution both on the surface and within the scaffold. Alternatively, porosimetry or porometry can be carried out, which involves placing a wetted scaffold in a sealed chamber, then a gas is introduced in the chamber. Bubbles from the fluid and gas form due to the pressure of the gas increasing, showing where the largest pores reside. The gas is continually introduced until the scaffold becomes dry, identifying the percentage of the scaffold that contains pores, and hence allowing its porosity to be determined.3 However, it should be noted that soft materials such as cornea scaffolds may not be able to withstand the pressure applied from the gas and it may not be possible to conduct porometry, giving inaccurate results. Besides, freezing can be carried out, but this can create ice crystals, which destroy the structures within soft scaffolds.
7. Biomaterials for tissue engineered cornea
Biomaterials for tissue engineering can be natural or synthetic, which both have advantages and disadvantages. The tissue engineer has to decide which biomaterial is most suitable, with its advantages outweighing its disadvantages. Natural biomaterials have the advantages of being highly biocompatible, controllable, and can function at the molecular level. However, natural biomaterials can provoke an immune response, increasing the chances of host rejection, and they can degrade quicker than needed, providing insufficient mechanical support, as shown in Table 1. Synthetic biomaterials are useful when it is necessary to control physical and chemical properties closely, allowing the manufacturer to mimic the native tissue closely via porosity and other required structure components. Synthetic biomaterials need to be carefully considered and evaluated before in vivo testing since degradation of some synthetic biomaterials can provoke unwanted adverse reactions that can harm the body, for example upon degradation or contact with a specific environment,45 as shown in Table 2.
Table 1 Natural biomaterials used for tissue engineering of cornea tissue, and their advantages and disadvantages
Biomaterial |
Advantages |
Disadvantages |
Ref. |
Collagen |
(I) Biodegradable |
(I) Unstable |
35, 36, 46–52
|
(II) Biocompatible |
(II) Degrades rapidly |
(III) Encourages cell adhesion |
(III) Expensive |
(IV) Easy to produce |
(IV) Shrinks at cell introduction |
(V) Component of stroma therefore compatible with corneal cells |
(V) Overall has poor mechanical toughness and elasticity (depends on the processing method) |
(VI) Can be crosslinked to improve properties |
|
(VII) Can be organised to match native tissue |
|
|
Gelatin |
(I) Cheaper than collagen |
(I) Degrades faster than collagen due to removed cross-linkages |
47, 49 and 53
|
(II) Derived from collagen |
|
(III) Same amino acid structure as collagen |
|
(IV) Better transparency than collagen |
|
(V) Good elastic modulus |
|
|
Chitosan |
(I) Found naturally in insects, algae, fungi and crustacean shells |
(I) Can swell in aqueous conditions if not crosslinked or combined with a stabiliser |
45, 54–56
|
(II) Non-toxic |
(II) Degrades in less than 8 weeks |
(III) Biodegradable |
(III) Compact internal structure reducing cell proliferation |
(IV) Encourages wound healing |
|
(V) Transparent |
|
(VI) Good mechanical strength |
|
|
Hyaluronic Acid (HA) |
(I) Found naturally in the vitreous humour of the eye |
Rarely, it may cause allergic reactions. Also, can increase eye pressure when injected into the eye. |
32 and 45
|
(II) Has good electrospinning ability |
|
|
Silk Fibroin (SF) |
(I) Very versatile material can be formed into various scaffold types and inexpensive |
(I) Not durable against UV radiation |
11, 47, 57–59
|
(II) Biodegradable and good cell viability |
(II) Opaque material |
(III) Sturdy in water |
(III) Not great biocompatibility |
(IV) Good mechanical properties |
|
(V) Porous scaffolds |
|
(VI) Can be combined with collagen to improve cell attachment and proliferation |
|
|
Chondroitin Sulphate (CS) |
(I) Similar to HA structure |
Increase in corneal thickness during storage has been observed. No side effect or disadvantage reported. |
32, 36 and 60
|
(II) Found naturally in the ECM |
(III) Used to therapeutically treat disorders |
(IV) Transparency increases over time |
|
Decellularized Cornea |
(I) Comparable properties to native cornea |
(I) Limited by human cornea donations |
61–63
|
(II) Low rates of immune-rejection |
(II) Require screening for diseases |
(III) Better transparency |
|
Table 2 Synthetic biomaterials used for tissue engineering of cornea tissue, and their advantages and disadvantages
Biomaterial |
Advantages |
Disadvantages |
Ref. |
Poly lactic acid (PLA) |
(I) Biodegradable |
(I) Takes 10 months to fully degrade |
64 and 65
|
(II) FDA approved |
(II) Poor cell adhesion without surface modification |
(III) Passes naturally from the body when degrading |
(IV) Flexible |
(V) Can be produced from plant extracts |
(V) Can be semi-crystalline or amorphous |
|
Poly glycolic acid (PGA) |
(I) Biodegradable |
(I) Excessively stiff |
45, 54, 64 and 65
|
(II) FDA approved |
(II) Mechanical properties become poor after 6 weeks |
(III) Passes naturally from the body when degrading |
(III) Poor cell adhesion without surface modification |
(IV) Stronger than PLA |
|
(V) Takes 4 months to degrade |
|
(V) Transparent after around 8 weeks |
|
|
Poly lactide-co-glycolide (PLGA) |
(I) Reduced stiffness compared to PGA |
(I) Shrinkage can occur when cells are introduced |
33, 47, 54, 64 and 66
|
(II) Degradation rate can be altered by changing ratio of PLA to PGA |
(II) Poor cell adhesion without surface modification |
(III) Not harmful to the body |
(III) Poor mechanical strength |
|
Poly-ε-caprolactone (PCL) |
(I) Biocompatible |
(I) Extremely slow degradation speed |
44, 45, 47, 67–69
|
(II) Biodegradable |
(II) Low-melting temperature |
(III) Good cell viability |
(III) Highly hydrophobic |
(IV) Approved as cell carrier for the retina and conjunctiva due to non-toxicity |
(IV) Poor transparency |
(V) Flexible |
(V) Poor cell adhesion without surface modification |
(VI) Inexpensive |
|
(VII) Can produce fine fibers when electrospun |
|
|
Poly vinyl acetate (PVA) |
(I) Biocompatible |
(I) Water soluble |
44, 56, 65 and 70
|
(II) Good transparency |
(II) Degrades quickly |
(III) Can produce nanofibers when electrospun |
(III) Poor mechanical strength |
|
(IV) Poor cell adhesion without surface modification |
|
Polyglycerol sebacate (PGS) |
(I) Elastic |
(I) Difficult to obtain |
52, 65 and 71
|
(II) Transparent polymer |
(II) Requires crosslinking |
(III) Biocompatible |
(III) Degrades quickly |
(IV) Biodegradable |
(IV) Poor cell adhesion without surface modification |
(V) Adaptable to control degradation rates and mechanical strength |
|
(VI) Can be used as a shape memory polymer |
|
|
Poly-L-lactic acid (PLLA) |
(I) Isomer form of PLA |
(I) Not as transparent as PLA |
45, 65 and 72
|
(II) Can produce fibrous scaffold for wound healing |
(II) Degrades slower than PLA |
(III) Transparency increasing over time |
(III) Poor cell adhesion without surface modification |
(IV) Good biocompatibility |
|
|
Poly-N-isopropylacrylamide (pNIPAM) |
(I) Encourages cell adhesion and growth |
(I) Monomer is toxic to neural tissue |
54, 73–75
|
(II) Non-cytotoxic and biocompatible |
(II) Expensive |
(III) Easy to manufacture |
(IV) Relatively simple to adapt mechanical properties |
(V) Changing the tissue temperature can alter attachment properties |
(VI) Thermosensitive hydrophobic/hydrophilic changes |
(VII) Can be combined with other materials to improve properties |
Polymers are more suitable than other synthetic and natural biomaterials for application in corneal tissue engineering, where their manufacture is relatively straightforward, and their biodegradability can be controlled.3 Polymers can be dissolved in solvents to create a solution suitable for shaping using different techniques.28 Nonetheless, polymers as scaffolds can prove disadvantageous due to their properties, resulting in toxic leaching or biodegrading too quickly, eliminating the structural support too early. Furthermore, their morphology can be poor due to their molecular weight or poor solution concentration, leading to scaffolds with fibers with excessive diameters or unacceptable layouts. Natural and synthetic materials can be combined to control their properties more closely and include more functional aspects, for example to improve their strength and reduce their degradation rate. Ideally, the biomaterials used for a tissue engineered cornea scaffold should have the ability to work similarly of that to the native cornea ECM, with as few side effects as possible. Table 3 presents the key information on the biomaterials used in cornea tissue engineering experiments in comparison to the native cornea. Overall, polylactide-co-glycolide (PLGA) has been demonstrated to be one of the best biomaterials with promising results for cornea regeneration.
Table 3 Synthetic cornea scaffold properties versus actual cornea results
Material(s) |
Cell source |
Fibre diameter Size Range (nm) |
Pore size (μm) |
Porosity (%) |
Strength (MPa) |
Strain (%) |
Elastic modulus (MPa) |
Water contact angle (°) |
Transmittance at 700 nm |
Transmittance at <400 nm |
Cell Viability |
Thickness (mm) |
Author(s) |
Actual cornea values |
Mixed |
NA |
NA |
NA |
3–5 |
NA |
0.3–7 |
90 |
98% |
85% |
NA |
0.5–0.6 |
NA |
PCL |
Limbal epithelium cells (LEpCs) |
90–174 |
0.2–4 |
85 |
1.56–1.92 |
27.42–32.74 |
0.3–7 |
NA |
28 |
11 |
100% |
NA |
69
|
Alginate and gelatin |
NA |
60.04–74.38 |
NA |
NA |
0.34–0.58 |
NA |
0.82 |
NA |
65% |
32% |
NA |
0.4–0.6 |
76
|
Collagen and PVA |
Human keratocytes (HKs) and Human corneal epithelium cells (HCEpCs) |
59.9–266.3 |
NA |
NA |
3.581 |
29 |
NA |
NA |
50% |
37% |
100% |
NA |
43
|
Collagen |
HKs and cow proteoglycans |
NA |
0.4–12 |
NA |
NA |
NA |
NA |
55.8 |
NA |
NA |
NA |
0.5–1.3 |
77
|
PCL and PGS1 : 1 13 wt% |
NA |
330–550 |
NA |
95.11 |
NA |
NA |
NA |
NA |
NA |
NA |
NA |
NA |
52
|
Collagen with electrospun fibers of CSXLF |
HKs and RPE |
NA |
NA |
69.4 |
NA |
NA |
NA |
NA |
80–84% |
72–79% |
75% |
0.391 |
78
|
Collagen with electrospun Fi–Fo fibers |
HKs and RPE |
NA |
NA |
NA |
NA |
NA |
NA |
NA |
80–84% |
72–79% |
Poor |
0.401 |
78
|
Collagen with copolymer hydrogels |
Corneal epithelium cells (CEpCs) |
NA |
0.14–0.19 |
NA |
NA |
NA |
NA |
NA |
NA |
NA |
NA |
NA |
79
|
Collagen |
Rabbit corneal fibroblasts |
88–186 |
NA |
NA |
NA |
NA |
NA |
NA |
NA |
NA |
NA |
NA |
35
|
Collagen and CS |
Human stroma keratocytes, HEpCs and HEnCs |
NA |
62.1 |
85 |
NA |
NA |
NA |
NA |
NA |
NA |
Good |
0.678 |
36
|
1 : 1 PCL and PGS (13% w/w) |
HCjEpCs and HCEnCs |
178–338 |
NA |
NA |
NA |
NA |
NA |
NA |
99 |
98 |
NA |
NA |
80
|
2 : 1 PGS/PCL (18% w/w) |
Human corneal endothelial cells (HCEnCs) and HCjEpCs |
208–402 |
NA |
NA |
NA |
NA |
NA |
|
99 |
98 |
NA |
NA |
80
|
3 : 1PGS/PCL (18% w/w) |
HCEnCs and HCjEpCs |
179–355 |
NA |
NA |
NA |
NA |
NA |
NA |
99 |
98 |
NA |
NA |
80
|
4 : 1 PGS/PCL (18% w/w) |
HCEnCs and HCjEpCs |
201–481 |
NA |
NA |
NA |
NA |
NA |
NA |
95 |
93 |
Great |
NA |
80
|
Collagen and PCL |
Rabbit corneal cells |
NA |
NA |
NA |
Suitable |
160 |
0.0375 |
95 |
99 |
99 |
NA |
NA |
40
|
Plasma treated PCL |
HEpCs and HLEpCs |
108–154 |
Good |
Good |
1.29–1.79 |
29–31.4 |
NA |
0 |
47 |
36 |
Good |
NA |
68
|
Collagen |
Human cornea stromal cells (HCSCs) |
NA |
NA |
Good |
2.1–5.3 |
28.29–51.21 |
24.17 |
NA |
54 |
33 |
Great |
NA |
57
|
Silk fibroin with lysophosphatidic acid |
Rabbit CEnCs |
NA |
NA |
NA |
NA |
NA |
NA |
35 |
42 |
78 |
Great |
0.006–0.008 |
11
|
Poly(L-lactic-acid-co-ε-caprolactone)(P(LLA-CL)) and SF 75 : 25 |
HCEnCs |
202–250 |
NA |
NA |
8.7–10.08 |
NA |
NA |
71.58 |
90.57 |
75 |
Great |
0.0514–0.0612 |
81
|
Chitosan, HA and gelatin |
Rabbit CEpCs |
NA |
NA |
NA |
NA |
NA |
NA |
NA |
89 |
85 |
Great |
NA |
56
|
PLGA |
Rabbit LCEpCs and corneal stromal cells (CSCs) |
40000–370000 |
NA |
NA |
NA |
NA |
NA |
NA |
NA |
NA |
Good |
NA |
66
|
PLGA 50 : 50 |
Rabbit LEpCs |
2000–3000 |
NA |
NA |
3.5–4.5 |
NA |
45 |
110–0 |
NA |
NA |
Good |
0.05 |
34
|
PVA |
Rabbit limbal stem cells |
NA |
NA |
Poor |
1.2–1.7 |
236.2–333.4 |
7.5 |
NA |
97 |
88 |
NA |
NA |
44
|
PCL (with above experiment) |
Rabbit limbal stem cells |
NA |
10–30 |
High |
1.1–2.5 |
402.6–451.8 |
15.3 |
20 |
NA |
NA |
Good |
NA |
44
|
Collagen |
Cell free |
NA |
NA |
NA |
0.224–0.348 |
NA |
1.749 |
NA |
NA |
NA |
NA |
0.493 |
82
|
8. Synthetic cornea scaffold fabrication methodology
Tissue engineering scaffolds can be produced in a multitude of ways, although only some methods may be suitable for cornea applications. One property all tissue engineering scaffolds need to have is the ability to replicate the native ECM to allow cells to adhere and proliferate appropriately, thus filling and repairing a defected area that would not heal by itself.
8.1 Electrospinning
Electrospinning is a widely used technique utilised for producing tissue engineered scaffolds, as shown in Table 4. The structure of electrospun fibers has been proven to act similarly to that of the natural ECM, creating an environment for cells to adhere and proliferate.43,69
Table 4 Electrospinning parameters used for different materials and their success
Material |
Syringe and needle type |
Voltage (kV) |
Flow rate (mL h−1) |
Collector distance (cm) |
Author |
Result |
PCL dissolved in trifluoroethanol (TFE) |
24 G |
15 |
0.5 |
13 |
69
|
Transparent cornea structure. |
Gelatin |
20 mL, 18 G |
12 |
0.18 |
10 |
76
|
Opaque but strong. |
9% aligned PVA-collagen |
5 mL, 23 G |
15 |
0.2 |
170 |
43
|
Good mechanical strength, low transmittance. |
1 : 1 PGS/PCL 13 wt% |
26 G |
12.5 |
1 |
12 |
52
|
Transparent stroma structure. |
1 : 1 PGS/PCL 13% (w/w) |
10 mL, 26 G |
12.5 |
1 |
12 |
80
|
Nearly fully transparent, slow degradation. |
2 : 1 PGS/PCL 18% (w/w) |
10 mL, 26 G |
18 |
1 |
12 |
80
|
Nearly fully transparent. |
3 : 1 PGS/PCL 18% (w/w) |
10 mL, 26 G |
20 |
1 |
12 |
80
|
Nearly fully transparent, higher cell viability than 1 : 1 and 2 : 1. |
4 : 1 PGS/PCL 18% (w/w) |
10 mL, 26 G |
20 |
1 |
12 |
80
|
Slightly less transparent than 1 : 1–3 : 1, fast degradation, higher cell viability than 1 : 1–3 : 1. |
Collagen crosslinked foams with fibers |
— |
13 |
0.24 |
10 |
78
|
Split-layer cornea structure. |
5 wt% collagen dissolved in acetic acid |
2 mL, 20 G |
4–9 |
0.05–0.3 |
— |
35
|
Fine collagen fiber 2D corneal scaffold. |
70 : 30 gelatin PLLA |
5 mL, 0.4 mm diameter |
15 |
1.8 |
18 |
72
|
Fibrous scaffold non-toxic. |
Plasma treated PCL |
24 G |
15 |
0.5 |
13 |
68
|
Good transparency, mechanical strength and cell proliferation. |
25 : 75 SF/P(LLA-CL) |
2.5 mL |
12 |
1 |
11 |
84
|
Good transparency, cell attachment and cell proliferation. |
PLGA onto microstereo-lithographed PEG diacrylate (PEGDA) |
5 mL |
10–13.5 |
1.8 |
15 |
66
|
Good cell attachment and proliferation, poor transparency. |
PLGA and dichloromethane |
5 mL, 0.8 mm needle |
10–15 |
3 |
20 |
33
|
Good cell attachment. |
PLGA on PEGDA templates |
0.8 mm |
12–15 |
1.8 |
15 |
85
|
Useful for shaping on templates. |
PCL/collagen |
12 mL, 20 G |
15 |
1 |
15 |
40
|
Hemispherical cornea scaffolds, good mechanical strength, transparent and good cell proliferation. |
PCL |
— |
20 |
0.5 |
15 |
44
|
High porosity, with good mechanical properties and transparency. |
PLGA 50 : 50 PLA to PGA |
5 mL, 0.8 mm |
10–15 |
3 |
— |
33
|
Fast degrading, good cell adhesion and proliferation. |
PLGA 50 : 50 PLA to PGA |
5 mL, 0.8 mm |
12.5 |
48 |
30 |
34
|
HAM alternative that can be stored for a long time, and good mechanical properties. |
PCL |
24G |
25 |
3.6 |
9 |
67
|
Biocompatible scaffold; however some cells would not adhere to it. |
PVA cross-linked with GA and HCL |
0.508 mm |
10–22 |
0.5 |
15 |
70
|
Increased viscosity changed fibers from beaded to uniform then to flat. |
Electrospinning usually involves polymer solutions, which are placed in a syringe connected to a needle (also known as a spinneret), and a voltage is supplied.83 The anode is connected to the needle tip, while the cathode is attached to a collector plate, maintaining a fixed voltage between them, applying a voltage between the two components. Pumping of the solution from the needle tip occurs at a specific flow rate, creating fibers from the solution in the syringe.76 The fibers created gather on the collector plate in either an aligned or random arrangement, both with various success in cornea application.28 Then, after leaving the fibers to set for some time, the scaffold becomes handleable and can be further processed by seeding cells or sterilisation since the solvents would have evaporated.72,76
Electrospinning processes can be highly interchangeable, with a variety of parameters that can affect the outcome of the fibers and the scaffold morphology if not selected carefully. Some of these parameters include needle type, applied voltage, flow rate, distance from the spinneret to the collector, temperature and humidity.28Table 5 shows some electrospinning parameters and the results obtained by experiments creating electrospun cornea scaffolds.
Table 5 How changes in parameters during electrospinning alter the outcome of scaffold fibers, using information and features mentioned by Salehi et al. (2013), Doshi and Reneker (1995), Kim, Kim and Park (2018) and Tang et al. (2010)40,52,70,86
Parameter |
Change made |
Effect |
Concentration of solution |
Increased concentration |
Viscosity could be too high, making the jet unstable. |
Reduced concentration |
Solution may not be thick enough and exit the needle as an uncontrollable jet. |
Voltage |
Increasing and decreasing the voltage |
Stream of solution fluctuates depending on the material. A ‘perfect’ voltage being when the jet from the needle changes from spherical to Taylor cone shaped. |
Voltage increased more |
Multiple jets arise from the needle tip making unpredictable fiber patterns. |
Needle diameter |
Decreasing the needle diameter |
Reduction in fiber diameter. |
Temperature and humidity |
Increase temperature and humidity |
The solution dries more rapidly due to the solvent evaporating faster. |
Collector to needle distance |
Needle close to collector plate |
Decreased bead formation, with fibers of smaller diameter. |
Needle further from collector plate |
Increased bead formation, random placement of electrospun fibers. |
Collector type |
Rotating collector |
Creates fibers which are orientated in an aligned manner. |
Smooth conductive surfaces e.g. aluminium foil |
Increased scaffold density, due to fibers being closely arranged. |
Mesh/porous surfaces |
Less crowded fibers, with scaffolds of lower density. |
Charged aluminium bars on plate |
Parallel aligned fibers created between the bars. Only works with conductive solutions. |
Metal pin in the centre of the collector plate covered by a dome-shaped insulating material with a conductive wire surrounding it |
Created circumferential alignment of fibers to mimic the shape of the cornea and to encourage cell growth in a circular direction. |
Feed rate |
Slow feed rate |
Reduced fiber diameter size and less bead formation. |
Electrospinning has been widely investigated with the goal of creating strong but fine fibers to allow maximum cell attachment. Generally, to alter the outcome of an electrospun scaffold, the parameters can be changed, as listed in Table 5, to tweak the fibers with compliant biomaterial solutions.
8.2 Microstereolithography
Microstereolithography can be used together with electrospinning, creating templates that can be used to control the shape of the scaffold during electrospinning.85
Typically, microstereolithography involves mixing a photocurable resin with a photoinitiator, and then the mixture is irradiated using a laser. The laser is then controlled by a computer to create specific shapes, curing the resin layer by layer as the reflected light is collected by a digital multimirror to show a projected image to shape into.66
This technique has been used to form a two-layer structure, containing a ring-shaped layer and a layer with micropockets to collect limbal cells. Ortega et al. used this scaffold as a potential limbal stem cell source for the cornea.66
8.3 Hydrogels
Hydrogels are non-fibrous scaffolds created by dissolving polymers in solvents and then freezing the solution to set into a hydrogel.87 Subsequently, the gels are dehydrated before placing them in various solutions, treating and sterilising before culturing cells into them.88 Hydrogels alone as a scaffold possess poor mechanical strength, which is because they lack fibrous structures to create a synthetic ECM; however their high-water content is useful for replicating the cornea native environment.76
Miyashita et al. used PVA hydrogels to create epithelial sheets for the cornea. These hydrogels were tested on rabbit cornea after culturing with human CEpSCs and rabbit epithelium cells, transplanting them into pockets made in the stroma. The success of this experiment was variable, with 33% of the trials resulting in PVA-collagen detachment from the epithelium, and the rest showing good epithelialisation. Tight junctions were considered present due to testing using horseradish peroxidase, which did not travel to the intracellular spaces, and microvilli were observed on the anterior cells. Overall Miyashita et al. showed that their hydrogels were potentially viable for use with keratoprotheses or epithelium healing; however no basement membrane was present, and the sutures loosened, causing detachment.88
Tonsomboon and Oyen combined alginate hydrogels with electrospun gelatin nanofibers crosslinked with either ethanol or water in attempt to mitigate the mechanical strength issues with hydrogels alone. This method involved electrospinning a gelatin scaffold, desiccating it, and then submerging it in an alginate solution. However, this mixture resulted in opacity compared to the hydrogels alone although the elastic modulus increased, and therefore is an unsuitable solution to replace defected corneas. In comparison to porcine cornea, the ethanol crosslinked gelatin scaffolds were less transparent, but exhibited higher mechanical strength, whereas the water crosslinked scaffolds showed better light transmission; however, this technique still requires development.76
Bakhshandeh et al. also created a corneal scaffold using a plasma-treated PVA hydrogel infiltrated with PCL electrospun nanofibers. PCL was electrospun using the parameters listed in Table 6, and then plasma treated. The PVA hydrogel was created by mixing the PVA with deionized water and dimethyl sulfoxide (DMSO), before heating and freezing it to create the gel. These two materials were combined, having the hydrogel as the centre of the scaffold with the PCL scaffold being cut into a ring and placed at the edge of the hydrogel. The authors found that this construct possessed good elasticity, transparency and cell adhesion. However, despite the high porosity in the PCL fibers, the hydrogel had no porosity, which will slow cell attachment, and thus requires modification.44
Table 6 Fabrication methods to produce synthetic cornea tissue
Cellular layer |
Cell source |
Scaffold material(s) |
Fabrication technique |
Experiment |
Author(s) |
Comments on success |
NA |
Rabbit corneal cells |
PCL/collagen |
Radially aligned electrospinning |
In vitro
|
40
|
Hemispherical cornea scaffolds, good mechanical strength, transparent and encouraged cell proliferation. |
NA |
HEpCs and HLEpCs |
Plasma treated PCL |
Electrospinning |
In vitro
|
68
|
Transparent and biocompatible scaffolds. |
Stroma |
HKs |
Cross-linked collagen – CS Foam |
Freeze Drying |
In vitro
|
78
|
Transparent structure, highly porous with good cell attachment. |
Epithelium (combined with above stroma) |
RPE |
Dehydrothermally cross-linked collagen |
Electrospinning |
In vitro
|
78
|
Transparent structure, poor RPE attachment. |
Stroma |
CEpCs |
Collagen with copolymer TERF |
Hydrogel |
In vivo – micropigs |
79
|
Similar refractive index, transparent, good hydrogel. |
Stroma |
Rabbit Corneal Fibroblasts |
Collagen type I with acetic acid |
Electrospinning |
In vitro
|
35
|
Transparent 2D stromal structure. |
Stroma |
Rabbit stromal cells |
Gelatin and PLLA |
Electrospinning |
In vitro and in vivo – in rabbits |
72
|
Cell ingrowth and tissue regeneration in the cornea, biocompatible. |
Stroma |
HCEnCs and HCjEpCs |
PGS/PCL |
Electrospinning |
In vitro
|
80
|
Allows good cell growth, great wet transparency. 4 : 1 ratio of PGS : PCL had best degradation rate, cell proliferation and viability, with slightly lower transparency than ratios 1 : 1–3 : 1. All scaffolds immune-compatible and elastic. |
Stroma |
HCSSCs |
RGD – treated silk films stacked |
Stacking of silk layers |
In vitro
|
57
|
Transparent, ECM environment produced with good mechanical properties and better cohesion than 2D silk scaffolds. |
Stroma |
HLEpCs |
PCL and TFE |
Electrospinning |
In vitro
|
69
|
Successful scaffold. |
Stroma |
NA |
Gelatin and alginate |
Electrospinning |
In vitro
|
76
|
Strong structure but slight opacity. |
Stroma |
HKs and HCEpCs |
PVA and collagen |
Electrospinning |
In vitro
|
43
|
Good mechanical strength, non-toxic. |
Stroma |
HKs |
Collagen |
Magnetic alignment |
In vitro
|
77
|
Structurally similar to the stroma, but translucent without proteoglycans. |
Stroma |
NA |
PGS/PCL |
Electrospinning with electrostatic field |
In vitro
|
80
|
Transparent structure, with aligned fibers like the native stroma (no mechanical testing done). |
Full |
HCEpCs, stroma keratocytes and HCEnCs |
Collagen/CS |
Lyophilization |
In vitro
|
36
|
Similar thickness, stratified epithelium layer formation with basement membrane forming and endothelial cell layer present. Transparent over time. |
Epithelium |
Rabbit CEpCs |
Carboxymethyl chitosan/gelatin/HA |
Blended membrane |
In vivo – rats and rabbits |
56
|
Fast degrading, high transparency, good CEpC attachment and increased healing speeds. |
Epithelium |
Rabbit LCEpCs and corneal stem cells |
PEG diacrylate with electrospun PLGA fibers on top |
Electrospinning and microfabrication |
In vitro
|
85
|
Good cell attachment, cell proliferation and near transparency. |
Epithelium |
Human and rabbit CEpCs |
PVA/collagen |
Hydrogels |
In vivo – rabbits |
88
|
Successful re-epithelialisation in 66.6% of trials, with tight junctions present but no basement membrane (might be due to ‘air-lift’ culturing). |
Epithelium |
LEpCs |
PLGA |
Electrospinning |
In vitro
|
33
|
Good cell attachment and proliferation. |
Epithelium |
Rabbit limbal explants |
PLGA |
Electrospinning and microfabrication |
Ex vivo – rabbits cornea |
85
|
Allows re-epithelialisation. |
Epithelium |
Rabbit LEpCs |
PLGA |
Electrospinning |
In vitro
|
34
|
Good cell proliferation even after scaffold storage. |
Epithelium |
Rabbit limbal stem cells |
PCL and PVA |
Electrospinning and hydrogel |
In vitro
|
44
|
Porous scaffold, with good elasticity and transparency. Good cell proliferation and adhesion. |
Epithelium |
Rabbit limbal stem cells |
PLGA |
Electrospinning |
Ex vivo – rabbit cornea |
98
|
Fast degradation rates, good cell adherence and regular appearing cells. |
Epithelium |
NA |
Recombinant human collagen |
Hydrogel |
In vivo – humans |
82
|
Stratified epithelium generation, nerve and stroma repopulation, few surface irregularities (due to suturing), slightly too weak. |
Endothelium |
Rabbit CEnCs |
Lysophosphatidic acid/silk fibroin |
Blended membrane |
In vitro
|
11
|
Good cell attachment and good biocompatibility, with better transparency and roughness than SF alone. |
Endothelium |
HCEnC |
SF/P(LLA-CL) |
Electrospinning |
In vitro
|
81
|
25 : 75 SF/P(LLA-CL) best transmittance, cell proliferation and good cell adhesion. |
Li et al. constructed a hydrogel to represent the stroma layer of the cornea using collagen mixed with various copolymers at different ratios to test the property change. The authors used contact lens moulds to set the hydrogels and crosslink the collagen/copolymer mixture. Phosphate buffer saline (PBS) was used to remove any unwanted functional groups, and subsequently sterilise the hydrogels. This resulted in a cornea-shaped tissue construct, which was transparent, encouraged cell growth and adherence, with no inflammatory affects when tested in vivo in micropigs. Additionally, nerve regrowth occurred after 3 weeks, with sensitivity slowly returning. In comparison, the collagen only hydrogels were opaque, thinner and mechanically weak.79
8.4 Self assembly
Self-assembly is a highly adaptable method to produce scaffolds, where the integrity of the structure can be altered by changing the solvent, solvent concentration, pH, temperature and ionic strength. This reversible technique involves various non-covalent bonding methods, which is performed in an environment where no spontaneous changes can occur, with or without dissipating energy to form structures. Self-assembly usually involves amphiphilic molecules and occurs naturally in lipids, bone, teeth and shell formation. When creating a self-assembled structure to form a scaffold, the individual fragments come together to form a whole mass, where a solvent–solute mixture is initially separated until the concentration range is exceeded by the solute concentration, forming micelles of different shapes.89
A group used this method to produce synthetic cornea containing three untransformed cell types, constructing a stroma using fibroblasts to create an ECM environment, seeding endothelial and epithelial cells on either side of the self-assembled stroma to mimic the native cornea. The resulting stratified epithelial cells and the endothelial cells formed a single layer of cells after 10 days and replicated the native cornea cells features closely.90
Self-assembled scaffolds are widely used in drug delivery applications, lessening the side effects of drugs, controlling the timing, solubility and dosage to specific locations by controlling the degradation rate of the drug carrier. However, improvement is still required for self-assembled structures to reduce their cytotoxicity and function more accurately under certain conditions.89
8.5 Lyophilization
Lyophilization is a method used to create foams by freeze drying, using a vacuum to convert a solid directly into a gas.
Acum and Hasirci (2014) used this method to create a split thickness cornea, replicating the epithelium, Bowman's layer and stroma layer. They created a foam with EDC/N-hydroxysuccinimide-cross-linked collagen–CS to represent the stroma with an electrospun collagen mat on top to mimic Bowman's layer, and then electrospun dehydrothermally cross-linked collagen fibers were collected on the top of this construct to act as the epithelium layer. In this experiment, the stroma layer was seeded with HKs and the epithelium layer with retinal pigment epithelium (RPE), with the intermediate layer representing Bowman's layer separating the cellular layers, while still allowing cellular interactions. Briefly, the method used involved impregnating the cross-linked foam with CS, then electrospinning the collagen fibers onto the foam, before dehydrothermally treating the scaffold. Dehydrothermal treatment proved useful in improving the mechanical strength of the scaffold by removing water from collagen and increasing the amount of covalent bonds. Then, both sides of the scaffold were seeded with the appropriately chosen cell type prior to culturing.78
Consequently, Acun and Hasirci produced a tissue engineered scaffold with a similar thickness to the natural cornea, having good porosity, fair transparency, uniform randomly oriented fibers and good cell adhesion to the foam. Degradation was relatively quick, where the weight of the scaffold was reduced by 60% over the course of a month. Also, the transparency improved with time as the collagen scaffold degraded and was replaced by native tissue. Overall, this method shows potential to produce an alternative to HAM for cornea replacement.78
Recently, another group in the United States investigated the therapeutic effects of lyophilized conditioned-medium derived from corneal mesenchymal stromal cells on corneal epithelial wound healing.91 The epithelial wound healing effects of fresh and lyophilized cMSC conditioned-medium were compared with conditioned-medium from non-MSC cells (corneal epithelial cells) using a scratch assay. In this study, to evaluate the anti-inflammatory effects of fresh and lyophilized cMSC conditioned-media, macrophages were stimulated by a toll-like receptor (TLR) ligand followed by treatment with the conditioned media and measuring the expression of inflammatory genes.91 Promising results were obtained; however, further investigation is required in this field.
8.6 Bioprinting
A new approach to producing cornea scaffolds involves the adaptation of the classic 3D printing, where biomaterials are printed to form cornea layers suitable for implantation.92 A 3D bioprinted corneal stroma equivalent was designed as a substitute for native tissue. A reproducible outer and inner organization of the stroma was obtained by optimizing the printing conditions such as the nozzle speed in the x–y direction and the spindle speed.93 Laser-assisted bioprinting with laser-induced forward transfer was utilised with laminin, HA and collagen together with cornea stem cells to produce structures similar to the native cornea, with a controllable cell organisation and unaffected cellular function.94 Sorkio et al. (2018) printed embryonic stem cells (ESCs) on top of a thick layer of a printed stroma scaffold containing adipose-derived stem cells, as demonstrated in Fig. 4. These bioprinted constructs proved to attach better to the experimental host cornea compared to Matriderm® sheets. This printed structure was mostly transparent; however, an opaque sheet of Matriderm® was required under the scaffold when cells were cultured to prevent construct shrinkage, which could be prevented by crosslinking the structures.94
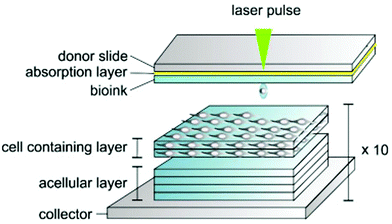 |
| Fig. 4 Method of bioprinting synthetic cornea tissue involving pulsed laser sources to set the bioink droplets, making a bespoke pattern to match the structure of the cornea (reproduced from ref. 94 with permission from Elsevier, Copyright 2018).94 | |
Extrusion-based bioprinting has also been used to create hydrogels with HCECs in collagen, gelatin and alginate.95 This enabled the creation of a highly porous construct, with controllable degradation, fast cell growth and good cell viability.
Bioprinting has not been widely used for cornea tissue engineering; however, it has benefits compared to other tissue engineering methods such as better controllability, improved cellular integration and unique alignment ability.
8.7 Scaffold-free cornea tissue engineering
Recently, Syed-Picard et al. created an alternative to allogenic transplantation using a scaffold-free tissue engineering approach. The authors cultured human corneal stromal stem cells on surfaces made of glass wafers and photoresist with aligned microgrooves to replicate the structure of the cornea. This allowed the peeling of the tissue from the surface, which was ready for transplantation into the cornea of mice because of the collagen fiber alignment and presence of proteoglycan keratocan. The tissue engineered corneal sheets were implanted into mouse corneal stroma pockets, and it was observed that the sheets exhibited increased transparency the longer they were implanted and no reactions occurred from the implant.96
However, although the implantation, transparency and biocompatibility of these sheets were tested, their mechanical strength, wettability and elastic modulus were not assessed in this study. Scaffolds are important in maintaining the structure of tissue engineered cornea, and thus without this mechanical support the construct may fail due to ocular pressures.97
Tissue engineering scaffolds serve as a necessary but temporary support for cell growth into a specific shape, which should degrade with time and are different from prosthetics, allowing tissue to regenerate entirely and perform more naturally.97 A tissue engineered scaffold should be biocompatible, act as an ECM for the cells seeded in it, and be strong enough to support itself in vivo. Accordingly, a variety of methods can be used to create these scaffolds, with various degrees of success achieved. Table 6 shows the various tests carried out to produce synthetic layers of the cornea using the methods discussed, with the resulting outcomes of experiments.
9. Characterisation methods
Characterisation allows the scaffolds produced to be analysed, enabling different material scaffolds to be compared using a variety of techniques.
9.1 Morphology and structure
Morphology can be analysed via microscopy examination using SEM or atomic force microscopy (AFM). Morphological evaluation is highly important to compare native cornea tissue structures to tissue engineered structures to determine their success and close resemblance.
9.2 Wettability
To test the hydrophilicity or wettability of a tissue engineered scaffold, contact angle characterization can be performed using a water contact goniometer.11 Wettability is important to estimate how fast a scaffold becomes wet and when degradation will start. This involves placing a small amount of (about 3 μL) deionised water (dH2O), phosphate buffered saline (PBS) or media (DMEM) on the tested surface and taking a photograph, and this image is then analysed via computer software to calculate the contact angle.69 The angle of a water droplet can be measured at set intervals to determine how the scaffold absorbs the water, and at different positions to determine how they may affect water intake and transparency.
9.3 Transparency
Spectrophotometry can be performed to test the transparency and light transmission of a tissue engineered scaffold, choosing an appropriate wavelength range that the cornea would endure naturally, and adding PBS to mimic the wetness of the cornea in vivo. Alternatively, to test visually, wet scaffolds can be placed over a piece of text and photographs can be taken to show the clarity of the text.69
9.4 Cornea thickness
Slit-lamp beams can be utilised to measure the thickness of a section of the cornea in vivo. This can be done by positioning the beam perpendicular to the cornea when a patient uses a slit-lamp machine and looks directly at the target. This considers the refractive angle and allows analysis of the thickness from the angle of the glass plate.99 A simpler in vitro method involves the use of digital micrometres with high precision, measuring to the nearest micrometre to allow comparison to the native cornea, or using software to measure the thickness from SEM images of the scaffold on its side. Optical coherence tomography (OCT) enables high-resolution imaging of the anterior segment of the human eye. In noncontact examination, it is possible to analyze the layers and shape of the cornea in cross-sectional images. This includes the option to determine the central corneal thickness.100 A research group also used anterior segment optical coherence tomography (AS-OCT) and investigated the epithelial thickness (ET) of the central cornea and limbal regions in patients with limbal stem cell deficiency (LSCD) as a diagnostic and staging parameter.101
9.5 Degradation rate
The degradation rate of scaffolds can be tested by placing the scaffold in PBS solution and analysing its weight loss gradually over months to enable calculation of how long it will take to break-down the entire scaffold. Alternatively, degradation can be determined based on changes in transparency, morphology (such as fibre thickness), porosity and handleability. The extent of breakdown has been assessed differently by various researchers, either with or without cells. Degradation was expressed as a percentage of weight loss of the dried membranes after incubation with cells in media for varying periods. The membranes were weighed at the beginning of the experiment and then each membrane was weighed weekly after removing it from the culture medium and drying it in a vacuum oven.34,98 The weight loss was calculated and expressed as a percentage of the initial weight. The weight loss percentages of the specimens were calculated from the weights obtained before and after degradation using the following formula (1), where W1 and W2 are the sample weights before and after degradation, respectively.
Weight loss (%) (W1 − W2)/W1 × 100. |
9.6 Porosity and fibre diameter
Fibre diameter and porosity are two important characteristics of polymeric fibres, which provide a significant amount of information for degradation and storage. The morphology, microstructure, fibre diameter, fibre integrity and degradation rate of scaffolds were examined previously by many research groups using SEM. The Image J software is a simple software, which was used by our group and other researchers to identify these two parameters.34,98
9.7 Scaffold storage
The storage of scaffolds is crucial since it determines how a long fabricated scaffold can be saved before being placed inside the body. Previously, PLGA membranes, both gamma-irradiated and non-sterile, were stored at a wide temperature range of −80 °C to 50 °C to assess their shelf-life over a 12 month period.33,34,98 Other membranes were also stored at 37 °C, where the membranes were placed in an empty Petri dish in an incubator containing 5% CO2 with high humidity. The water absorption by the membranes was detected using silica orange desiccant. Fibre integrity was assessed using SEM. The results demonstrated that the scaffolds could be stored for up to 24 months without any changes in their morphology and behaviour.34,98
9.8 Sterilisation
There are different options available to sterilise scaffolds such using ethanol and gamma irradiation. However, despite its higher cost and small change in the structure of fibres, gamma irradiation is the most suitable methodology to sterilise scaffolds. Usually membranes (mounted in 12-well plates) are sterilised via γ-irradiation with an external dose range of 25–40 kGy.
9.9 Scaffold handling
One of the characteristics of a suitable scaffold for cornea tissue engineering is that the membrane can be handled easily by surgeons without failing. Brittle scaffolds are not suitable for this purpose, and thus significant efforts have been undertaken to optimise the handling property of scaffolds.34,98 Previously, rabbit limbal epithelial cells (rLEC) were cultured on membranes for 6 weeks at 37 °C in a humidified atmosphere. The physical changes in the membranes were recorded daily by taking optical micrographs, and membrane handling was carried out simply by using a pair of forceps after they were removed from the culture (in wet conditions) and scored as easy to handle, fragile or very brittle.
10. Cell sources
A stem cell niche resides in a unique and protected microenvironment to modulate its role and fate via internal and external factors. Thus, damage or disruption to these surroundings can ultimately lead to opacification and vascularization, which may induce reduced vision, pain, and photophobia.102 The relevant progress in evolving living substitutes has heralded a dominant approach in the clinical interest and application of tissue engineered products.103 In an effort to generate in vivo three-dimensional cornea constructs, the most comprehensively investigated cell sources hinge on two fundamental dichotomies, i.e. autologous versus allogeneic and pluripotent stem cells versus multipotent cells.104 Various experiments have shown that the use of different cell types can result in huge differences in eyesight, alleviating corneal scarring and preventing immunological reactions.105
Comparatively, stem cell sources tend to be more advanced than using donated cornea to heal disorders and diseases, with immunological reactions minimized, storage capabilities upgraded and improved outcomes for patients (Fig. 5 and 6). Mesenchymal stem cells can be obtained from tissues such as adipose tissue, bone marrow, hair follicles, the placenta, and umbilical cord.105 Interestingly, the efficacy of mesenchymal stem cells derived from bone marrow and adipose tissue has been found to be suitable for cornea tissue regeneration,106 with human umbilical mesenchymal stem cells proving to enhance transparency.107,108
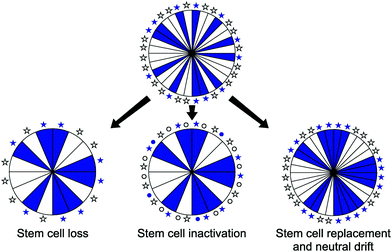 |
| Fig. 5 Decline in corneal epithelial stripe numbers with age may occur by loss, inactivation or replacement of limbal epithelial stem cells (reproduced from ref. 109 with permission from Elsevier, Copyright 2018).109 | |
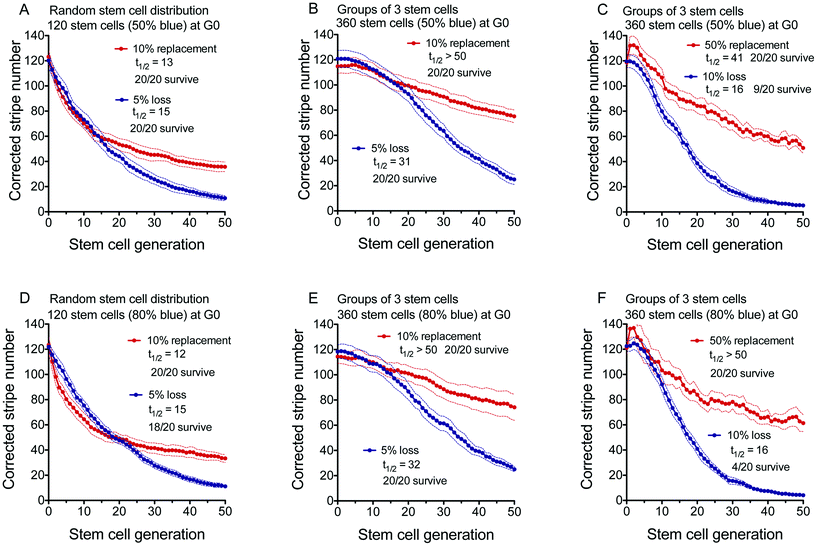 |
| Fig. 6 Decline in corrected stripe number in simulations of limbal epithelial stem cell loss or replacement. (A) Random stem cell distribution 120 stem cells (50% blue), (B and C) Group of 3 stem cells 360 stem cells (50% blue) at G0, (D) Random stem cells 120 stem cells (80% blue) at G0, (E and F) Group of 3 stem cells 360 stem cells (80% blue) at G0, (reproduced from ref. 109 with permission from Elsevier, Copyright 2018).109 | |
10.1 Aberrant corneal stem cell niche function
The lack of proper functioning CESCs through ocular surface disease is perhaps a result of pathological alterations occurring in the limbus stem cell niche. In describing the sentence above, it should be noted that several pathological conditions eventually result in stem cell deficiency or abnormal activity in the immune system (such as lupus, graves, rheumatoid arthritis, Goodpasture syndrome, and type 1 diabetes) or inflammatory responses (uveitis disease).
Abnormal immune responses can induce profound changes in the microenvironment of CESCs, and thus impair their maintenance and/or survival. For example, immune-mediated pathology (such as specific killer T cells, natural killer cells, polymorphonuclear leukocytes, and macrophages, directly attack pathogens) can damage niche components (including antibodies, cytokines, chemokines, and complement, to aid in the war against foreign invaders) and induce conditions such as fibrosis in the sub-epithelial stroma (immune response condition can act as a double-edged sword).110–112
Ocular metabolic alterations such as oxygen tension and nutrient availability in the niche can also be tested, and the up- and down-expression of a range of growth factors and cytokines have been shown to exhibit some effect on stromal stem cells.111
Chemical or thermal injury and genetic disorders such as aniridia (for example Pax6 gene deficiency damages the interaction between CESCs and stromal stem cells in the limbus) may also damage stem cell function through alterations to their niche.113
Chronic inflammation induces metaplasia on the cornea by stimulating abnormal limbus stem cell niche remodelling (mechanotransduction in corneal stem cells). In the normal condition, corneal stem/progenitor cells differentiate into corneal epithelial cells (CK3+ and CK12+), which form a defensive barrier on the ocular surface. Thus, the abnormal differentiation of corneal epithelial stem and/or progenitor cells is an indirect consequence of microenvironmental changes induced by chronic inflammation.
These cells can be introduced into cornea scaffolds by seeding using bioreactors; alternatively, they can be injected into the cornea in vivo and used to treat corneal issues without a scaffold if the damage is minimal.105
Table 7 lists a variety of cell types commonly used in cornea tissue engineering, together with the various successes and pitfalls of using these cells. Also, we discuss the various cell types used in the field of cornea regeneration.
Table 7 Cell sources for tissue engineering of synthetic cornea tissue, and their advantages and disadvantages
Cells |
Advantages |
Disadvantages |
Ref. |
Mesenchymal stem cells (MSCs) – general |
-Can differentiate into required keratocytes |
-Potential immune rejection |
106, 114–118
|
-Flexible to function both in vivo and in vitro |
-Difficult to obtain on a large scale |
-Extensively trialled |
-Later generations of MSCs have limited differentiation capability |
-Allows new collagen to be produced in the stroma layer |
-Reorganises collagen to improve transparency |
-Inhibit inflammation local to the injury |
|
Umbilical cord MSCs |
-Causes no pain to obtain |
-Rarely used autologously due to being obtained after birth |
119–121
|
-Umbilical cord would otherwise be put to waste |
-Despite the risk being low teratomas can occur |
-Ethical to use |
-Storage of cells is difficult |
-Improved self-renewal capabilities |
-Time consuming to prepare for scaffold implantation |
-Lower risk of rejection due to immunosuppressive factors |
-Comparable to human corneal fibroblasts |
|
Bone marrow MSCs |
-Lots of clinical data |
-Painful and difficult to extract |
116 and 121
|
-Good differentiation potential for keratocytes |
-Do not maintain their multipotency for as long as umbilical cord stem cells |
-Recommended to use when limbal stem cells are unobtainable |
-Reduces neovascularization |
-Reduces corneal opacity |
|
Adipose derived MSCs |
-Used to mimic the stroma layer of the cornea |
-Not as effective to counteract cornea hydration |
94, 116 and 122
|
-Ability to organise horizontally and in a lamellae fashion representing the natural cornea stroma structure |
-Good survival in HA to source keratocytes |
|
Autologous corneal limbal stem cells |
-Can be combined with decellularized tissue to mimic the native cornea histologically |
-Limbal stem cells quantities autologously may not be sufficient for extraction and expansion |
116 and 123
|
-Good transparency properties |
-Returns cornea to correct thickness post-injury |
-Studies show this cell type can improve wound healing the best |
-Reduces neovascularization |
|
Autologous extraocular stem cells |
-Procedure to obtain cells is not overly invasive and is efficient |
-If tissue source is not from the cornea (for example from the skin), histological features may vary to the native cornea. |
105 and 124
|
-Can be sourced from adult adipose tissue |
-Good differentiation capability |
|
Corneal stroma stem cells (CSSCs) |
-Derived from the cornea |
-Isolation of cells is difficult due to source size |
105
|
-Requires patient to have no bilateral eye diseases |
|
Embryonic stem cells (ESCs) |
-Limbal epithelial ESCs allow formation of the stratified epithelium layer of the cornea closely mimicking the native cornea |
-Raises various ethical issues |
94 and 125
|
-Can be bioprinted |
-Little clinical data to work with |
|
Induced pluripotent stem cells (iPSCs) |
-Possibly can differentiate into any cell type |
-Fibroblasts require inducing to become iPSCs |
105, 118, 125 and 126
|
-Sourced from differentiated adult stem cells |
-Expensive method to differentiate cells |
-Can be bioprinted effectively |
-Inefficient and slow sourcing method |
-Self-renewing |
-Risk of teratoma occurring if used in vivo |
10.2 Limbal epithelial stem cells or corneal epithelial stem cells (LESCs)
The limbus rim is typically well-known as the niche location for corneal epithelial stem cells. Structurally, LESCs as a sub-population are located within the basal epithelial papillae of the palisades of Vogt.127 The palisades of Vogt are radially orientated fibro-vascular ridges that are tightly positioned at the superior and inferior limbal edges.128 Majo et al. challenged the concept of the exclusiveness of the limbal zone.16 They described that mouse corneal epithelium could be sequential transplanted, self-renewed and consisted of oligopotent stem cells with the capability to create goblet cells if provided with a conjunctival environment.16 Tseng et al. challenged this study since their findings were incompatible with many known cell behaviors such as cell growth, cell fate and differentiation and cell migration properties of the corneal epithelia.129 However, Chang et al. revealed that the corneal epithelial cell proliferation and migration in the center of the human cornea were comparable to that in the periphery even after collapse of the limbus, at least within the first 12 h after wounding.130
10.3 Alternative source of stem cells
Complete bilateral limbal epithelial stem cell deficiency (LSCD) cannot be treated via the common clinical transplantation of autologous limbus (transplant from the patient's own healthy eye) or ex vivo cultivated autologous limbal epithelium (CLET).131 Allogenic limbal epithelium transplantation is possible, but requires the use of immunosuppression and has a transplantation success rate that tends to be reduced progressively over time (success rate of 40% at 24 months and 33% at 48 months).132 The clinical use of in vitro cultured cornea stromal and endothelial stem cells is not applicable because of the intricacy in the separation of stem cells and absence of optimized culture media. Consequently, the discovery of alternative cell sources, both ocular and non-ocular, is essential, which should be easily accessible and from which a large quantity of cells can be obtained.
10.4 Conjunctival epithelial stem cells
Pellegrini et al. in their comprehensive in vitro study revealed the uniform distribution of candidate stem cells in the bulbar and forniceal conjunctiva.132 They presented that conjunctiva epithelial and goblet cells are derived from progenitor cells with high proliferative capability, which produce goblet cells at least twice during their life cycle.133 Qi et al. reported that the molecular pattern expression of markers in the bulbar conjunctival epithelium basal layer cells is similar to that of corneal/limbal epithelium cells. Clinically, ex vivo cultured conjunctival epithelial stem cells have been applied to successfully treatment patients with ocular surface injury. However, the problem is that most LSCD patients do not have a healthy conjunctiva to be used for cell culture and transplantation.134
10.5 Human deciduous dental pulp stem cells
Kerkis et al. separated and identified human deciduous teeth immature dental pulp stem cells, which were so-called human immature dental pulp stem cells (hIDPSC).135 hIDPSC were revealed to express both mesenchymal stem cell markers and human embryonic stem cell markers and to differentiate into derivative cells of the three germinal layers consisting of mesoderm, ectoderm and endoderm. In another study, it was revealed that hIDPSC express limbal stem cells markers and were capable of restoring the cornea of a rabbit after total limbal stem cell deficiency.136 However, there is no indication and confirmation of the effectiveness of this process in human ocular surface subjects.
10.6 Human hair follicle stem cells
Numerous studies have focused on applying hair follicles (HF) as an interesting source of adult SC for cell therapy and tissue engineering. It was displayed that HF contain mesenchymal stem cells in their dermis, epidermis and connective tissue sheath (CTS), which have potential to differentiate into hematopoietic, adipogenic, osteogenic, chondrogenic, myogenic, and neurogenic lineages.137 Meyer-Blazejewska et al. revealed that HFSCs could be reprogrammed into a human corneal epithelial phenotype using conditioned media generated from corneal and limbal stromal fibroblasts.138 In another experiment, it was demonstrated that HFSCs could go beyond their lineage boundaries and terminally differentiate into a different epithelial cell phenotype in vivo when grafted into a specific niche microenvironment in a murine model of limbal stem cell deficiency (LSCD).139
10.7 Oral mucosal epithelium
Oral mucosal epithelial cells (OMECs) are satisfactorily available, can be separated by minimally invasive procedures, and possess high proliferation potential in vitro, which make them ideal seed cells for regenerative medicine. Oral mucosal SCs are also located in the basal layer and express LSC markers and can be reprogrammed into corneal epithelial-like cells.140 Oral mucosal epithelial cells have the capacity to engraft onto the ocular surface and survive after transplantation in patients with LSCD following alkali injury141 and are considered to be safe, despite the risk of contamination. The cultured autologous oral mucosal epithelial cell sheet (CAOMECS) is a transparent, resistant, viable, and rapidly bio-adhesive cell sheet, cultured with UpCell-Insert technology (CellSeed, Inc., Tokyo, Japan), which allows grafting onto the patient's corneal stroma without suturing.142 Therefore, it has been proposed as an alternative treatment for total bilateral LSCD. Burillon et al. performed a clinical trial to confirm the safety and efficacy of CAOMECS with a prospective, noncomparative study in 26 eyes of 25 patients.131 Two patients experienced serious adverse events, one with corneal perforation and the other with massive graft rejection. The treatment was found to be effective in 16 of the 25 patients at 360 days after grafting. Among the 23 patients who completed follow-up at 360 days, 22 had no ulcers, and 19 showed a decrease in the severity of the punctate epithelial keratopathy.141
10.8 Mesenchymal stem cells (MSCs)
MSCs have been identified in the bone marrow, skin, umbilical cord, amniotic membrane, and adipose tissue. They are multi-potent, express mesenchymal and embryonic SC markers and are capable of differentiating into cells of the three embryonic layers.143
10.9 Bone marrow-derived stem cells (BMSC)
BMSCs are multipotent and can differentiate into cells with ectodermal, mesodermal and endodermal origin.144,145 Ma et al. demonstrated that BMSCs can be used to treat corneal disorders.146 Their findings suggested that the transplantation of human BMSCs on human amniotic membrane successfully restored the damaged corneal surface in rats. Remarkably, the healing effect of the transplantation was related to the absence of inflammation and angiogenesis after transplantation of MSCs rather than the epithelial differentiation from BMSCs.146
Ye et al. demonstrated that systemically transplanted BMSCs could engraft to injured cornea to promote wound healing by differentiation, proliferation, and synergizing with haemotopoietic stem cells in a rabbit model of alkaline burn.147,148
Bone marrow-derived progenitor cells could be encouraged by inflammatory mediators and played a critical role in corneal wound healing following alkali burn in rabbits.149 Corneal alkali burn induces a rapid bone marrow reaction to release not only inflammatory cells, but also progenitor cells into circulation. Migrated bone marrow-derived progenitor cells can target local sites to promote wound healing. The effect of corneal injury on the mobilization of endogenous MSCs and targeting the injured cornea was also displayed by Lan et al.149
10.10 Adipose-derived stem cells (ASC)
Adipose-derived stem cells (ASCs) are a source of mesenchymal stem cells with self-renewal property and multipotential differentiation. ASCs have two main benefits, namely, ASCs can be simply accessible from subcutaneous liposuction in large numbers with minimal risk and they have no ethical and political issues compared to embryonic stem cells because they can be derived from autologous fat. These two properties make ASCs a more suitable solution for tissue and organ transplantation in regenerative medicine and clinical training.
Arnalich-Montiel et al. were the first group to investigate the ability of human PLA cells to repair/regenerate the corneal stroma of rabbits.114 Human processed lipoaspirate-derived (PLA) cells differentiated into functional keratocytes when injected into an ablated corneal stroma after 8 and 12 weeks, as assessed by the expression of the cornea-specific proteoglycan, keratocan, and aldehyde dehydrogenase (ALDH). Funderburgh et al. showed that ASCs could differentiate to keratocytes in vitro.150
10.11 Induced pluripotent stem cells (iPS)
Deriving transplantable CECs or LESCs from iPSCs has tremendous potential to be the ideal option to treat CE and ocular surface diseases, but it is still a challenge since the conditions and signals to derive them in human context are inadequately understood.151 Most of the protocols for differentiating ESCs or iPSCs into CECs are based on our understanding of ectoderm development. During embryogenesis, CE originates from the head/ocular surface ectoderm.151
Differentiation of CECs from hPSCs has been proven to be rather challenging, with most of the previously published studies relying on the use of undefined factors, such as conditioned medium, PA6 feeder cells, and Bowman's or amniotic membrane.152–154 Protocols to derive corneal epithelial cells from iPSCs have provided critical insight into the role of each of the exogenous factors incorporated in the culture process.155–158
Most studies aim to use defined in vitro conditions towards generating CECs from iPSCs such that the protocols can be reproducible and lead to the development of clinical grade production of corneal epithelial cells.159 The directed differentiation of iPSCs into CECs depends on the expression of cytokeratins (CK) 12 and 13, while CK3 expression was evident in the cell lines derived from the CE.160,161 To improve the yields of mature CECs and to obtain a stratified cell sheet resembling the native CE, a consistent and efficient stratification method has to be employed. It is not uncommon to detect variation in the differentiation potential among different hiPSC lines, with donor identity and gender being among the potential sources of variation in the case of hiPSC lines.162,163 Therefore, different iPSC lines from multiple sources should be rigorously tested in terms of appropriate cell morphology, gene, and protein expression.
11. Growth factors for the cornea
Growth factors are protein molecules that bind to cell surface receptors, which can be beneficial for tissue engineering, acting as cell signalling molecules to encourage or inhibit cell growth, reduce or increase inflammation, improve cell viability and upregulate or slow healing (Fig. 7).
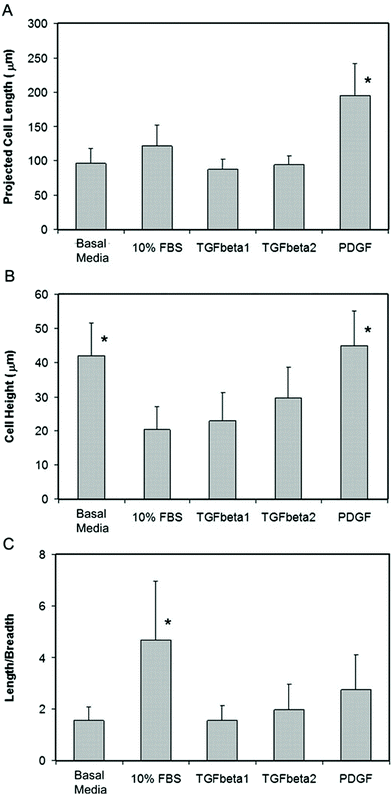 |
| Fig. 7 Difference in keratocyte morphology when exposed to various growth factors in 3D culture. (A) Projected Cell Length, (B) Cell Length, (C) Length/Breadth (reproduced from ref. 164 with permission from ARVO, Copyright 2010, Open Access).164 | |
There are many different types of growth factors commercially available, each playing a unique role in cellular stimulation, requiring a set dosage, concentration and combination to work effectively.165 When stem cells are required in large quantities, it may be useful to implement growth factors to increase the number of stem cells prior to harvesting them. The addition of growth factors to synthetic cornea constructs can lessen the recovery period for the recipient, while reducing the number of undesirable symptoms a patient may encounter from an implant. Table 8 presents some of the growth factors that have reported in cornea tissue engineering (Fig. 8).
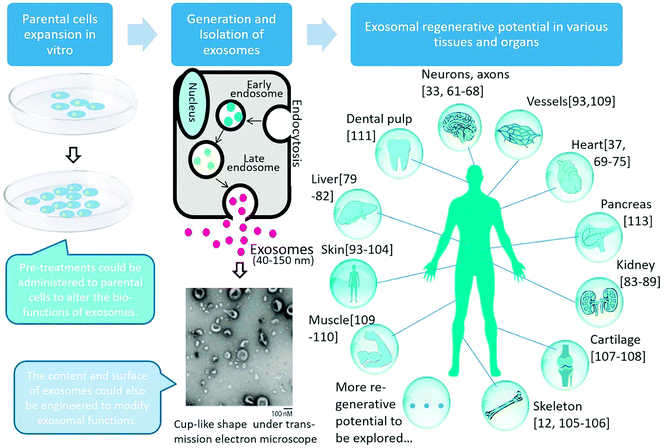 |
| Fig. 8 Exosomal generation and regenerative potential in various tissues and organs. Exosomes originate from the endosomes generated by endocytosis. After further processing, exosomes are released through membrane fusion. The regenerative potential of exosomes has been reported in many tissues and organs, such as the nerve, heart, liver, kidney, skeleton, cartilage, muscle, pancreas, and dental pulp. It is reasonable to believe that more regenerative potential of exosomes will be discovered in the future (reproduced from ref. 166 with permission from Elsevier, Copyright 2018).166 | |
Table 8 Growth factors present in the cornea and their effects on tissue repair
Growth factor |
Function |
Ref. |
Vascular endothelial growth factor (VEGF) |
-Encourages cell migration |
105, 167 and 168
|
-Improves cell viability |
-Improves ECM formation |
-Mediates lymphatic and blood vessel formation |
-Increases corneal transparency |
-Reduces inflammation |
|
Platelet-derived growth factor (PDGF) |
-Encourages cell migration |
105, 164 and 169
|
-Can increase corneal haze |
-Improves cell viability |
-Improves ECM formation |
-Causes lengthening of keratocytes without stress fiber formation |
|
Hepatocyte growth factor (HGF) |
-Encourages improved corneal cell migration and proliferation |
105, 170 and 171
|
-Supresses myofibroblast generation enabling better transparency |
-Improves cell viability |
-Reduces corneal inflammation |
-Reverses inflammatory side effects that prevent proliferation |
-Improves ECM formation |
|
Transforming growth factor (TGF-β) |
-Encourages cell migration and organisation |
105, 164, 170–176
|
-Improves cell viability |
-Increases conjunctival scarring |
-Increases mRNA count |
-Causes corneal transparency to decrease |
-Improves ECM formation |
-Stimulates connective tissue growth factor (CTGF) |
-Increases collagen production in corneal fibroblasts |
-Can be increased in quantity by improving stroma rigidity |
-Encourages formation of endothelial layer similar to native tissue |
|
Mesodermal growth factors |
-Accelerates healing of corneal endothelial cells |
165
|
-Stimulated keratocyte proliferation |
|
Connective tissue growth factor (CTGF) |
-Increases collagen production in corneal fibroblasts |
169, 172 and 177
|
-Controls collagen synthesis effected by TGF-β |
-Promotes scar formation |
-Reduces corneal neovascularization |
|
Fibroblast growth factor (FGF) |
-Stimulates cornea epithelium, endothelium and keratocytes |
165 and 178
|
-Enables complete epithelium healing after PRK |
|
Insulin-like growth factors |
-Can increase keratocyte growth |
165 and 179
|
-Suitable with or without limbal stem cell deficiency |
|
|
Epidermal growth factor (EGF) |
-Stimulates cornea epithelium, endothelium and keratocyte regeneration (especially the endothelium) |
165, 180, 181
|
-Endothelium growth can further be enhanced with insulin-like growth factors alongside EGF |
-Raises the strength of wounds in the stroma |
-Requires long contact to begin mitosis |
12. Cell-free products (exosomes)
The exosomes are a group of extracellular vesicles with different cellular origins and contents. Exosomes can enter the cells to regulate the internal mechanisms of repair and heal the affected area. Exosomes transfer proteins (such as EGF, FGF, brain-derived neurotrophic factor (BDNF), insulin-like growth factor-1 (IGF-1), nerve growth factor (NGF), FGF-1, and glial cell-derived neurotrophic factor (GDNF)) and nucleic acids (including microRNAs (miRNAs), messenger RNAs(mRNAs), and even DNA), which can facilitate their uptake by distant target cells through endocytosis, and thus exosomes can target a specific cell or cells to enhance or interfere with specific biological processes such as intercellular signalling and communication with stromal cells. They are enveloped by a lipid bilayer enriched in cholesterol, sphingomyelin, and ceramide. The membrane of exosomes contains surface markers such as CD9, CD63, and CD81, which can be used to identify exosomes. Han et al. studied the effectiveness and importance of exosomes in ocular surface wound healing, and in 2017, they identified and characterized corneal epithelial-derived exosomes. Their results demonstrated the important role of exosomes in facilitating the intercellular interaction between the epithelium layer and the stroma layer through corneal repair. Thus, exosomes may be employed as a therapeutic approach for corneal repair.182
Exosomes present significant benefits over cells/stem cells with respect to manufacturing, storage, handling, product shelf-life and potential as a ready-to-go biologic product.183,184 Consequently, the application of exosomes derived from stem cells instead of using cells has attracted attention due to their potential advantages in regenerative medicine.182,184–188
Previously, researchers recognized that exosomes are one of the more important secreted constituents in mediating and carrying out the biological functions of MSCs.189–191 Exosomes, which are bilipid membrane-bound nano-vesicles with a size in the range of 40 to 100 nm, are known to transfer bioactive molecules among cells and play critical roles in wound healing and angiogenesis.182,190,192,193
Exosomes released from human-induced pluripotent stem cell-derived MSCs have the ability to repair tissue by promoting collagen synthesis and angiogenesis. Similarly, adipose MSC-derived exosomes promote cutaneous wound healing via optimizing the properties of fibroblasts such as migration, proliferation, and collagen synthesis (Hu et al., 2016). Human umbilical cord MSC-derived exosomes may accelerate wound healing due to their impact on collagen synthesis through the Wnt-4 signaling pathway.183,194
Recently, the use of exosomes in the treatment of ocular diseases has been attracting attention. In a recent study, Bai et al. found that human umbilical cord MSC-derived exosomes greatly reduced the intensity of ongoing experimental autoimmune uveoretinitis by reducing the infiltration of T-cell subsets and other inflammatory cells in the eyes.192 Furthermore, Yu et al. showed that human umbilical cord MSC-derived exosomes ameliorate laser retinal injury via a mechanism involving MCP-1 downregulation.195 In another study, Han et al. showed that mouse corneal epithelial-derived exosomes fused to stromal keratocytes in vitro and induced myofibroblast transformation, suggesting that exosomes may be involved in corneal wound healing.182
Exosomes have several advantages compared to the actual delivery of MSCs to the site of injury. Exosomes can be isolated readily through centrifugation, providing the benefits of MSC-mediated paracrine repair without the risk of immunological rejection, malignant transformation, and obstruction of small vessels associated with cell therapy.184 Furthermore, they can be safely stored since they have excellent stable chemical properties and high biosecurity. The bilipid membrane of exosomes can maintain encapsulated proteins, messenger RNA (mRNA), and microRNA (miRNA) under stable conditions to exert a lasting effect.196,197 Therefore, they can be formulated as a topical gel or drop and locally administered. They can also be reprogrammed to be carriers for therapeutic agents. Finally, due to their smaller size, they are also capable of migrating deep into the corneal stroma compared to cells.198
Future studies are still needed to determine the precise mechanism by which cMSC exosomes induce corneal epithelial wound healing. In particular, further investigations based on their content analysis (proteins, mRNA, and miRNA) are necessary to elucidate the mechanisms of their regenerative effects.183
13. Ethical and sustainability issues associated with engineering cornea tissue
Ethical considerations should balance the attention of the planet, people and profit, ensuring no areas are ignored.
Social and economic status should not mean that a person is more or less likely to receive synthetic cornea. It could be considered that synthetic corneas are more attainable in developing countries than natural tissue transplantation since they may result in less complications in the recipients.
Biomaterials sourced from living animals can raise some ethical and moral issues, especially in the case where the life of an animal is sacrificed to obtain these materials. The recipients will need to be informed of the animal or human compositions included in the synthetic cornea.32 Consideration should be made that the inclusion of human or animal components in the construction of synthetic corneas can reduce the number of eligible recipients wanting the cornea transplant, and they may instead request keratoprostheses, which is a less successful solution.
Cells need to be seeded into the tissue engineered scaffolds, and then need tested in vitro and in vivo before implantation, mostly using rabbits. Culturing cells that are suitable for differentiation into human corneal-like cells can require either scraping of cells from the cornea of the recipient or obtaining corneal cells from the cornea of a cadaveric donor. This is required to culture specific cells to enable cornea structure reformation; however, can be deemed unacceptable to some people, and thus alternatives should be available.
When testing these corneas in vivo, if animals are required, the Animals (Scientific Procedures) Act 1986 should be followed, which is in place to recognise the need for medical and scientific research and testing where there are no other viable alternatives, but ensures that animals have minimal distress, pain and lasting harm.
The improvement of people's lives may outweigh the loss of life of an animal since the use of the animal will help multiple causes, if seen as a utilitarian approach with the benefits outweighing the risk, but this can depend on the individual view since some people anthropomorphise animals more than others.
Donation of human tissue or cells must be voluntary, and consent must be appropriately obtained, and guidelines must be met following the Declaration of Helsinki before procedures take place in removing the tissue or cells. The use of these bodily materials can also be at the discretion of the donor, and their requirements followed. With tissue or cell donation, there is also the issue of genetic identification, since genetic coding embedded within cells creates the risk of donor identification and potential breach of confidentiality or privacy if one were to seek the origin of the cells.
Sustainability is a common issue among engineering challenges, with materials regularly being sourced and obtained, but not being replenished. Production should be optimised to reduce the expenditure of unnecessary resources; however, although there is an emphasis on making a profit, if a more sustainable approach is to be undertaken, then there needs to be a balance between the economics, environmental and society concerns. For something to be sustainable, it must not cause the unnecessary reduction of natural resources, and consequently minimal damage to the environment.
The biomaterials used biodegrade over time since they are required to allow sufficient cell regeneration; however, the production of these polymers may require excessive energy use, which may or may not be environmentally friendly. Scaling up this project will require the use of a vast amount of polymer; however, large batches of solution can be made for electrospinning and the processes monitored to reduce waste from mistakes where possible.
If the production of synthetic corneas becomes unsustainable due to the materials required or the techniques used, it should be considered and calculated how much they will realistically benefit society, and whether these benefits outweigh the risks, responsibly understanding the choice that is being made. Environmental damage can cause a secondary effect, impacting humans negatively, for example droughts can cause crop depletion and storms can result in building damage and even deforestation, leading to the destruction of potential medicinal findings. Every aspect be considered thoroughly based on the environment, other species and the impact on humans.199
14. Corneal translational medicine
14.1 Translational ophthalmology
The main concept of translational science, which aims to facilitate the application of laboratory (in vitro, ex vivo and animal models) scientific findings to the diagnosis and treatment of human diseases in clinical setting, is currently vague.200
Basic science is expanding scientific knowledge at a phenomenal rate through in vitro experiments, investigations of model organisms and use of cross-discipline methodologies.201 However, the development of improved clinical applications utilizing this information is complex and unpredictable. Progress does occur via studies designed to understand a specific disease or treatment developed according to defined strategies. However, novel concepts often arise in ways not readily connected to an obvious clinical problem. The language and ideas, not to mention the methods of investigation, can be foreign and impenetrable to those not yet experienced with the diverse experimental and biological systems currently used. The fact that many breakthroughs occur outside the realms of visual system research presents a severe and mounting challenge to ophthalmologists. This section will meet the needs for the vision community by presenting up to date reviews in translational science. The articles have two goals, first, to review an area of basic research at a level accessible to clinicians, and second, to highlight clinical applications in ophthalmology.201 The ultimate goal is to present concepts and methods that may have applications not yet realized for treating patients. We hope to draw attention to basic areas of science with potential for further development or exploration in eye and ocular diseases. The goal is to stimulate the clinical audience to investigate unexpected avenues for possible applications in their own specialty and to expose basic researchers to opportunities for applying their expertise to clinical problems.202
14.2 Full-thickness human corneas
The main goal in human corneal tissue engineering is to create a full-thickness artificial cornea with the reconstruction of the complex stroma being the most demanding part. To summarize the results presented thus far, none of the bioprinting approaches have been able to satisfy all the demands. Some studies show high survival rates and elongation of human CSKs, but suboptimal mechanical properties.203 Others were able to produce constructs with a high light transmission, but with low biomechanical stability and lack of cell–cell interactions.93 Future developments in 3D bioprinting have to optimize the technology and components to match the complexity of the human corneal stroma. As introduced at the beginning of this article, human corneas are made of five distinct layers. Considering that corneal stromal bioprinting has shown encouraging results to date, it should be expanded to manufacturing the remaining layers of the cornea in vitro, despite their distinct characteristics compared to the stroma. In the study by Zhang et al.,204 human CEpCs were embedded in 15% GelMa hydrogels and printed by extrusion. In another study by Kim et al. (Kim, 2018),205 human CECs were embedded in a gelatin–RGD bioink and printed in an amniotic membrane support. In both studies, the cells remained round inside the matrices after printing and did not form mono/multilayers, challenging the choice of materials and the need for printing these very thin layers with an automated dispensing mechanism. In the human cornea, CEpCs form a non-keratinized stratified squamous epithelium. These cells have a hexagonal shape and form a tight monolayer. Since human CEpCs generally proliferate and migrate from an intact limbus to the cornea, it can be hypothesized that these cells can migrate onto a bioprinted corneal construct without further need to fabricate an additional epithelial layer in vitro. However, engineering the corneal endothelium in vitro may be beneficial considering that human CECs are postmitotic in vivo and do not proliferate. Accordingly, CECs can be added to the solution to form a monolayer next to the corneal stroma 3D construct after bioprinting.
14.3 Corneal replacements and transplantation
The need for alternative options to cadaveric corneas will become increasingly important as a result of the increasing incidence of transmissible diseases (human immunodeficiency virus), aging of the population, and the popularity of refractive surgery, which renders corneas unusable for later transplantation.206 In the context of severe ocular surface pathologies, where the risk of transplant rejection is significantly higher, synthetic homologs, which are known as keratoprostheses, are chosen as an alternative to donor corneal grafts for full-thickness corneal replacement. Keratoprostheses are used to replace corneas at high risk of immunological rejection or endothelial failure after penetrating keratoplasty and in eyes with limbal stem cell deficiency. Several keratoprostheses are in clinical use, but the Boston type I keratoprosthesis is the most common (Fig. 9). It is composed of front and back plates, an optical polymethylmethacrylate section, and a titanium locking ring mounted on a donor cornea, which serves to anchor the device in the host eye. Short-term visual recovery is good, but long-term prognosis is limited by various complications, including glaucoma and endophthalmitis, requiring a high level of medical intervention, thus reducing the long-term use of these devices. Furthermore, osteo-odonto-keratoprosthesis has shown good long-term anatomical survival rate and is currently the most common treatment in the case of end-stage inflammatory corneal disease (Fig. 10), such as Stevens-Johnson syndrome and thermal and chemical burns.206
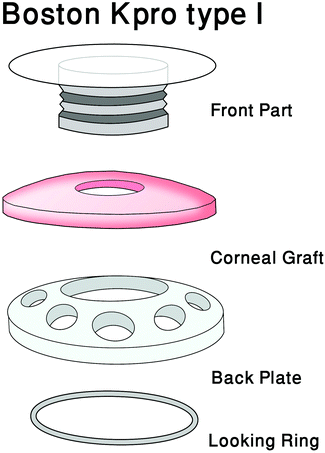 |
| Fig. 9 Boston Kpro type I contains a front plate with an optical cylinder made of poly(methyl methacrylate) and a back plate made of titanium (reproduced from ref. 9 with permission from Elsevier, Copyright 2019).9 | |
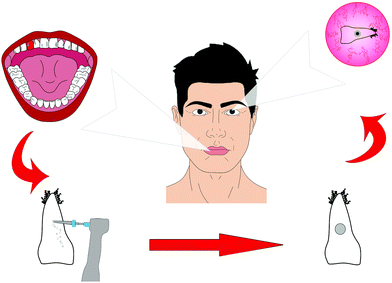 |
| Fig. 10 OOKP (osteo-odonto-keratoprosthesis) type of Kpro originally developed by Strampelli in the 1960s and refined by Falcinelli in the 1970s (reproduced from ref. 9 with permission from Elsevier, Copyright 2019).9 | |
Corneal transplantation remains the preferred treatment option for advanced stages of stromal and endothelial diseases. Even though tremendous advancement in surgical techniques has been made in the last twenty years, many factors remain that limit long-term success, such as global scarcity of donor material, high costs, limited graft survival, allogeneic graft rejection, the need for immunosuppressants, prolonged post-surgery management, and the need for highly trained surgeons to perform the procedures.207,208 Despite the increasing number of donors in the last few years, the growth of the global population and the demographic trends will exacerbate the worldwide issue of donor material shortage. Even in regions with well-developed eye banking systems, e.g., the USA and Western Europe, many potential donor corneas have to be eliminated because of the positive testing for transmissible viruses (such as hepatitis B and C).209 Additional aspects, such as medication history and religious beliefs, further reduce the donor pool. All these factors promote the search for suitable engineered tissue substitutes.
Strategies: Corneal tissue engineering can bypass many of the complications of conventional corneal transplantation, and thus has gained increasing attention recently. The human cornea is avascular and immune privileged. Therefore, it is an ideal organ for tissue engineering since rejection and inflammatory responses are less likely to occur compared to other locations.210 However, the complex nature of corneal tissue to maintain clarity and biomechanical stability and bio-integration of the engineered substitutes remain the main challenges. Prior strategies include the construction of the ECM and cells or a combination of both for the epithelial, stromal and endothelial layers.211 Few research groups have targeted more than one layer. Epithelium: Corneal epithelial cells proliferate continuously from the limbus onto the cornea and also onto centrally implanted tissue-engineered corneal substitutes.212,213 In the case of insufficient migration or adherence, the surface of the construct can be optimized by coating it with collagen, laminin, fibronectin, and fibrin.214 Recently, in corneal epithelial tissue engineering, much effort has been given to searching for biocompatible, mechanically stable, and optically transparent substrates that allow efficient cell adhesion, migration, and proliferation for the ex vivo expansion of LSCs to treat LSCD.214–216 To date, the human amniotic membrane (hAM) has been the most commonly used biological matrix due to its inherent growth factor content, ability to facilitate epithelialization, low immunogenicity, and antifibrotic, antiangiogenic, antimicrobial, and antiviral properties.215–217 However, hAM has several limitations. Due to the possibility of disease transmission, variation in growth factor content depending on the storage method,218 and donor characteristics,82 processing following caesarean section is demanding, particularly to avoid bacterial contamination,50 and, ultimately, the exact mechanism of its action remains unclear.217 Accordingly, based on the variable quality of this material and its reduced transparency,214 new studies have focused on the investigation of biological and synthetic alternatives. For example, collagen constructs promoted CEpC growth in vitro and in animal models.214 Nevertheless, the high water content of collagen hydrogels limits their stability, which can be increased by either mechanical compression or chemical crosslinking.216 Fibrin sealant was used as a substrate for the expansion of LSCs in the treatment of more than 113 patients with LSCD.219,220 Optically robust, highly permeable, and elastic, corneal constructs have been produced from tropoelastin–silk films, supporting CEpCs and CECs in vitro.221 Human anterior lens capsules supported LSCs; however, their availability and fragility limit their clinical application.222 Human hair keratin films showed high light transmission, but suture loosening resulted in their poor anchoring to the ocular surface.222 Human LSCs could successfully be cultivated on glutaraldehyde cross-linked chitosan–gelatin combinations. The scaffolds with a higher gelatin content degraded more rapidly, whereas that with a higher chitosan content was more stable, but also more brittle.223 However, ocular surface reconstruction in vivo with these constructs has not been tested to date. Synthetic polymers offer standardized mass production and lack of biological material, thus obviating concerns of disease transmission. The results for FDA-approved polymers, such as poly(lactide-co-glycolide) (PLGA), hydroxymethylacrylate (HEMA), and polymethacrylate carriers, are limited to in vitro studies thus far.103,224 In rabbit models, implants made from polyethylene glycol (PEG) and polyacrylic acid (PAA) led to corneal inflammation, haze, and ulceration.225,226 Thus, further investigations are needed to evaluate their potential. Stroma: In corneal stromal tissue engineering, different components have been tested similarly to epithelial tissue engineering. Collagenous materials with crosslinking or mechanical compression have shown improved construct stability but limitations in cell viability and matrix remodeling.216,227 Collagen vitrigels contain a high proportion of water, which renders them intrinsically weak, unless blended with other polymers, to create collagen composites or chemical crosslinkers. However, modification can limit the direct seeding of cells within the scaffold. Nevertheless, they do promote dendritic branching, cell elongation, and CSK expression of keratocan and ALDH in vitro.228 Other substances, that have been investigated as alternative stromal biomaterials include PLGA, gelatin, and chondroitin sulfate.229,230 Cell-free corneal implants comprising recombinant human collagen and phosphorylcholine were recently grafted by anterior lamellar keratoplasty into the corneas of unilaterally blind patients diagnosed at high-risk for transplant rejection. Grafting promoted nerve regeneration, as observed by improved touch sensitivity and vision in three out of the six patients.221 Many corneal stromal tissue-engineered constructs have difficulties meeting the demands of tensile strength, natural surface curvature, and stromal architecture of the human cornea, which can result in reduced optical transparency. Nevertheless, promising approaches to address these issues have recently been published. The use of curved templates helped to generate more realistic corneal curvatures and facilitated CSK and collagen alignment, leading to an improved elastic modulus.222 Using peptide amphiphile-coated surfaces with different anisotropies and cultured human CSKs, Gouveia et al. created corneal stromal self-lifting analogous tissue equivalents (SLATEs).231 These SLATEs were comprised of aligned collagen fibrils, and hence imitated the microarchitecture of the human cornea, and were more transparent, thicker, denser, and more resistant to proteolytic degradation compared to the SLATEs formed with randomly oriented constituents. The constructs also integrated well in a rabbit corneal model. In another study, Miotto et al.223 induced self-curving of collagen-based hydrogels via contraction-inhibiting peptide amphiphiles in certain regions of the gels. However, the contraction was facilitated by alpha SMA-expressing corneal stromal cells (i.e., Myo-SFs), which can cause corneal scarring and reduced transparency. Ghezzi et al.103 used patterned, porous, thin, and optically clear silk protein films stacked in an orthogonally, multi-layered architecture, resembling the human cornea, seeded with human CSSCs, to generate 3D functional corneal stroma tissue equivalents. The cells secreted characteristic ECM and remodeled the constructs. Another promising approach is the use of decellularized corneas (DC) from animal and human origins since they retain the prevailing three-dimensional ECM structure, biomechanics, biocompatibility, and transparency.224 The complete removal of all cell remnants is essential to reduce immunogenicity, and the preservation of the ECM ultrastructure enables efficient recellularization and high biocompatibility. To date, different protocols to decellularize entire corneas or thin stromal lenticules have been promoted, but there is no agreement on the best approach.226 The key difference in the protocols is the preservation of the crucial membranes in the tissue, i.e., Bowman's and Descemet's membrane, with respect to decellularization efficiency.225–227 The drawbacks that still need to be addressed are the loss of ECM components, alterations of the stromal structure, biomechanical stability and inability to allow complete recellularization by the host's CSKs post-implantation.225–228 Endothelium: Human CECs are postmitotic in vivo, and thus do not proliferate. However, in vitro, hCECs have been expanded and reinjected into the eyes of patients with endothelial disease.229 Attachment was facilitated by face-down positioning of the patients. Similarly, hCECs can also be seeded on stromal constructs and biological matrices, e.g., gelatin, collagen I gels (vitrigel), gelMA,230 and animal and human DC81,232–235 or synthetic lamellae, e.g., PLGA, poly-L-lactic acid (PLLA), and chitosan.236,237 These tissue engineered carriers were transplanted onto Descemet's membrane-stripped recipient corneal stromal beds in different animal models.81,232,233,235 Synthetic polymers provide high purity with known chemical composition, physical properties, structure, and degradation times. Nevertheless, some components can induce inflammatory reactions.236 Biological carriers, particularly DC lamellae, advantageously represent a natural substrate for hCECs.238 However, grafts can be rejected, especially in the cases of insufficient decellularization, and they may transfer infections. In addition, the use of carriers from human material does not reduce the dependency on donor tissue, even though multiple grafts can be engineered from one donor cornea.
14.4 Stem cells from wisdom teeth
Stem cells from the dental pulp of wisdom teeth have been reported to have potential to turn into cells of the eye's cornea such as stroma cells. Researchers demonstrated that these stem cells have the potential to become a new source of corneal transplant tissue made from the patient's own cells.239 Dental pulp cells (DPCs) contain a population of adult stem cells, and similar to corneal stroma, develop embryonically from the cranial neural crest. DPCs have the potential to differentiate into cells of the corneal stoma (keratocytes). These findings demonstrate a potential for the clinical application of DPCs in cellular or tissue engineering therapies for corneal stromal blindness, which is a big step towards translational medicine in the field of ophthalmology.
15. Clinical trials in corneal tissue engineering
The first treatment developed to reverse LSCD was transplantation.240 For patients with unilateral limbal stem cell deficiency, three different methodologies are available. The first involves transferring a large limbal graft from the healthy eye to the LSCD eye. This methodology decreases the chances of rejection due to an immunoreaction.241 However, there is always the risk of triggering an LSCD phenotype on the healthy eye, which can lead to bilateral LSCD.242 This approach is not applicable for patients with bilateral LSCD.243 Even if the limbal graft is the best method to reverse the LSCD phenotype, patients with unilateral LSCD can also be treated with conjunctival limbal autograft.244 The addition of conjunctival tissue decreases the size of the limbal tissue surgically removed from the healthy eye, thereby decreasing the risk of LSCD formation on the healthy cornea. However, the addition of the conjunctival tissue increases the risk of conjunctival tissue invasion over the cornea because the barrier function of the limbus is not restored. The second methodology involves using organs from deceased people, which are the easiest to harvest for transplantation. The same approach was used for corneal transplantation. Dr Zirm performed the first corneal transplantation with some success in 1905.245 He transplanted corneas from a deceased boy to the cornea of an adult patient. One of the grafts experienced complications, but the graft on the second cornea maintained the transparency of the patient cornea. Allograft treatment is usually performed for bilateral LSCD by transplanting the cornea of deceased people, and autografts are usually performed for unilateral LSCD.208,244,246–248 In 1993, Langer introduced a new scientific term, tissue engineering.249 Tissue engineering combines the fields of biology and engineering to develop artificial tissue that can to restore or improve the tissue function. This technology requires the use of stem cells, which can be isolated from any human organ.
The etiology of LSCD varies. In total, 249 patients were recruited in clinical trials of LSCD caused by Stevens-Johnson syndrome (n = 89), chemical burn (n = 114), ocular cicatricial pemphigoid or pseudo-ocular cicatricial pemphigoid (n = 57), and thermal burn (n = 6). Among them, the factors related to LSCD corneas were variable and played an important role in the outcome of the transplantation grafting (not transplantation). The average age of the patients was 50.6 years old, ranging from eight to 86. The donor's age may play a role in the outcome of the LSCD treatment. No data was reported about the potential relationship between patient age (the age of the oral mucosal epithelial cells) and the outcome of the treatment after grafting. A review reported that in vivo aging mesenchymal stem cells show decreased proliferation potential, differentiation potential, and telomerase length and an increase in genetic instability.250 The number of mesenchymal stem cells isolated from tissues can decrease with age,251 which can also be the case for oral mucosal epithelial cell donors. However, no information has been published about the number of cells isolated per patient or about their potency. A decrease in MSC proliferation and differentiation potential was reported with a reduction in the colony forming assay.251,252 Aged people were reported to have a greater number of larger and flatter cells in the oral mucosal epithelium, which can be related to a decrease in progenitor cells.253 In addition to their decrease in cell proliferation, it was also reported that mesenchymal stem cells are more sensitive to oxidative stress, undergo more apoptosis, and their capacity to differentiate is decreased.251,252,254 Different protocols were suggested as solutions to improve cell viability and resistance to aging, such as the use of xeno-free expansion culture media255 and drugs.256,257 The data obtained from aging MSC can be used to improve the treatment of LSCD with oral mucosa epithelial cells (e.g., composition of the culture media). The definition of a disease is one of the important criteria to understand the outcome of a treatment. We did not review the definition of LSCD since a complete review was published by The International Limbal Stem Cell Deficiency Working Group.258 Only patients with total LSCD and the loss of palisades of Vogt were recruited in these studies. If the central cornea was transparent, patients were not recruited. For most studies, Schirmer's test was not performed on the patient, especially for severely dry eyes. Based on Shimazaki's study, the outcome of transplantation could be improved if the Schirmer's test is above 10 mm,259 but only 14.3% of the studies reported the use of the Schirmer's test. For the inflammation status, the inflammation must be under control or it can jeopardize the success of treatment.260 Usually, inflammation occurs after corneal surgery, which is treated with the injection of steroids.261 Additional surgeries on the eye must be performed due to abnormalities such as symblepharon or fornix.131,141,261 Many patients have these abnormalities, and thus they should be treated before the cell sheet transplantation is carried out to improve the treatment outcome.131 In some studies, symblepharon or abnormalities were reported, and these were related to the patient outcome. All these factors (abnormalities, inflammation, and duration of LSCD) should be recorded and connected per patient to determine a potential relationship between a successful or failed transplantation. Among the studies, 85% used NIH 3T3 fibroblasts as feeder cells and 71.4% used amniotic membranes. Fetal bovine serum (FBS) or autologous serum was used to grow the epithelial cells into cell sheets in 47.2% of the studies. Bovine serum or derived bovine products are not fully rejected by the FDA, but manufacturers must provide the origin of the products.262 In some studies, the authors used autologous serum for the growth of the cell sheets263–266 or reported the use of bovine fetal serum and autologous serum without mentioning how the cell sheets were engineered with the serum.267–270 For FBS, each autologous serum lot was variable between patients, adding a variable that could affect the cell sheet engineering manufacturing process, but allowed the patient to use their own serum with their own cells. However, the FDA recommends the use of chemically well-defined culture media for translational purposes in the absence of animal products271,272 (FDA source, Class II Special Controls Guidance Document: Tissue Culture Media for Human ex vivo Tissue and Cell Culture Processing Applications; Final Guidance for Industry and FDA Reviewers). This was the case in 19% of the reported studies for at least the serum used in cell culture.
Engineering cell sheets with Food and Drug Administration (FDA) approved products is a difficult task, and their harvesting requires specific protocols and growing surfaces. Different methodologies have been developed to facilitate corneal-like cell sheet harvesting, including the use of fibrin glue,273 contact lenses,274 thermo-responsive surfaces,275 and dispase.276,277 The last three methodologies were used for human studies. For the majority of the reported clinical trials, amniotic membranes were used to harvest and graft the cell sheet (71.4% of the clinical trials). Amniotic membranes were initially directly grafted onto the cornea, before being used as a cell sheet carrier.278 Tseng et al.281 showed that the addition of allograft limbal stem cells to the amniotic membrane improved the healing process and the corneal epithelium recovery when both were grafted on the cornea. Similar results were obtained when corneal epithelial cells were cultured on amniotic membranes.279 For total epithelium defects, the combination of epithelial cells with an amniotic membrane produced higher and faster reepithelization of the cornea compared to the graft with only the amniotic membrane.280,281 In both cases, amniotic membranes were used as a carrier for cell sheet grafting and as feeder cells. Amniotic membranes are widely used for cornea cell sheet transplantation;279,282,283 however, their availability is limited, and the membranes require the use of sutures to attach the cell sheet to the cornea. Mouse fibroblast feeder cells are used in co-culture with the amniotic membrane.141,261,263,265–267,270,284–286 An innovative approach involved the use of a thermoresponsive surface that detaches the cell sheet from the culture by decreasing the temperature to below 32 °C. Poly-N-isopropylacrylamide was developed in 1968 by Heskins and Guillet.287 The hydrophobicity of this intelligent polymer varies based on temperature. By becoming hydrophilic below 32 °C, water penetrates under the cell sheet and detaches it without damaging the cell sheet or the extracellular matrix under it. Numerous types of cells were successfully tested using a thermoresponsive surface, including keratinocytes, endothelial cells, hepatocytes, cardiomyocytes, oral mucosa epithelium cells, and corneal epithelial cells.142,275,288–290 Nishida and colleagues pioneered the use of a thermoresponsive surface to manufacture cell sheets for limbal stem cell deficiency. In two studies (9.52% of the studies), stratified cell sheets were engineered on an intelligent surface in the presence of mouse feeder cells and grafted on limbal stem cell deficient eyes.131,275 The polymer did not affect the stratification of the cell sheet, the polarity of the cell sheets, and the stemness of the seeded progenitor stem cells. After harvesting, the cell–cell junctions and the extracellular matrix were maintained. A gentle enzymatic approach was used by Kim in two human studies205,276 (9.52% of the human studies). Engineered cell sheets were detached using dispase treatment, which cleaves collagen and fibronectin from the extracellular matrix.291 In these studies, the cell sheet morphology and phenotype were not studied, but the results after transplantation showed that the cell sheet curative properties were not altered by the dispase treatment. All these approaches do not require sutures to maintain the cell sheet on the cornea, which is an important feature for healing damaged corneas. However, the majority of the clinical trials reported the use of sutures (usually 10–0) to maintain the cell sheet on the top of the cornea, with the use of contact lenses to protect the cell sheet from mechanical damage from the eyelid.
16. Conclusion
Technology involving tissue engineering is continuously advancing with various methods and solutions to produce synthetic cornea tissue. For a successful tissue engineered cornea to be produced, the scaffold, which supports the cells, should be non-cytotoxic, allow cell attachment, provide a suitable environment for cell growth and enable transparency, while maintaining strength to withstand the ocular pressures of the eye. Scientists and engineers are continuously working together to find a universal method to produce synthetic cornea tissue to improve eyesight and heal the cornea, aiming to meet the ever-growing need for human tissue.
Future perspectives
Despite recent great advances in tissue engineering technologies, clinical application of these tissue engineered scaffolds still is not a treatment option for patients. Many combinations of biomaterials have been tested and trialled with no universal answer for which materials or cells are best suited to enable cornea tissue regeneration. Tissue engineered scaffolds can hold the answer to regenerating diseased or damaged cornea, either to repair partial or full-thickness defects, which are more adaptable than other treatment alternatives.
Clinical trials have taken place with some scaffolds; however, their success rate is still low and requires further attention to prevent rejection, enable sufficient remodelling in sync with the scaffold degradation and meet the needs of the in vivo environment. Having a suitable in vitro environment that mimics the natural in vivo environment of the cornea can enable an extensive testing process for patient treatment.
Conflicts of interest
There are no conflicts to declare.
References
-
A. Asadi, R. Andrews, H. Kelly, G. Whitaker, A. Innes and J. Sheppard, How it Works Book of The Human Body, Imagine Publishing Ltd, 2013 Search PubMed.
- Cornea of the Eye https://www.allaboutvision.com/resources/cornea.htm, 2017.
-
E. Schutte, G. Picciolo and D. Kaplan, Tissue engineered medical products (TEMPs), ASTM International, West Conshohocken, 2004 Search PubMed.
- T. Mimura, S. Yamagami and S. Amano, Prog. Retinal Eye Res., 2013, 35, 1–17 CrossRef CAS.
- D. W. DelMonte and T. Kim, J. Cataract Refractive Surg., 2011, 37, 588–598 CrossRef.
-
F. R. S. Gray and H. Carter, Anatomy Descriptive and Surgical, Bounty, London, 2012 Search PubMed.
-
J. Krachmer, M. Mannis and E. Holland, Cornea, Elsevier Mosby, Philadelphia, 2005 Search PubMed.
-
W. Trattler, P. Majmudar, J. Luchs and T. Swartz, Cornea Handbook, SLACK Incorporated, Thorofare, 2010 Search PubMed.
-
S. Yousaf, S. Keshel, G. Farzi, M. Momeni-Moghadam, E. Ahmadi, I. Asencio, M. Mozafari and F. Sefat, Sacffolds for corneal tissue engineering, Woodhead Publishing, 2019 Search PubMed.
- K. M. Meek and C. Knupp, Prog. Retinal Eye Res., 2015, 49, 1–16 CrossRef.
- J. H. Choi, H. Jeon, J. E. Song, J. M. Oliveira, R. L. Reis and G. Khang, Nanomaterials, 2018, 8, 290 CrossRef.
- S. E. Wilson, S. A. Lloyd, Y.-G. He and C. S. McCash, Invest. Ophthalmol. Visual Sci., 1993, 34, 2112–2123 CAS.
- F. M. Watt and B. L. Hogan, Science, 2000, 287, 1427–1430 CrossRef CAS.
- E. Shirzadeh, S. Heidari Keshel, V. Ezzatizadeh, S. Jabbehdari and A. Baradaran-Rafii, J. Cell. Biochem., 2018, 119, 2666–2678 CrossRef CAS.
- M. A. Dziasko and J. T. Daniels, Ocul. Surf., 2016, 14, 322–330 CrossRef.
- F. Majo, A. Rochat, M. Nicolas, G. Abou Jaoudé and Y. Barrandon, Nature, 2008, 456, 250–254 CrossRef CAS.
- O. Ilhan-Sarac and E. K. Akpek, Curr. Opin. Ophthalmol., 2005, 16, 246–250 CrossRef.
- A. Viestenz, B. Seitz, H. G. Struck and A. Viestenz, Clin. Anat., 2018, 31, 39–42 CrossRef.
-
C. Rapuano and W. Heng, Cornea: Color Atlas and Synopsis of Clinical Ophthalmology, Mc Graw Hill, New York, 2003 Search PubMed.
- E. I. Paschalis, C. Zhou, F. Lei, N. Scott, V. Kapoulea, M.-C. Robert, D. Vavvas, R. Dana, J. Chodosh and C. H. Dohlman, Am. J. Pathol., 2017, 187, 1327–1342 CrossRef CAS.
-
M. Wang, Corneal dystrophies and degenerations, American Academy of Opthalmology, San Francisco, 2003 Search PubMed.
-
E. Nathan, The Cornea its Examination in Contact Lens Practice, Butterworth Heinemann, Oxford, 2001 Search PubMed.
-
H. S. Dua and D. G. Said, in The ocular surface: functional anatomy, medical and surgical management, Karger Publishers, edition, 2015, pp. 1–25 Search PubMed.
-
J. B, Advances in Medical and Surgical Cornea, Springer Berlin, Berlin, 2014 Search PubMed.
- M. Gune, M. Umul, A. Güneş and M. Altok, Med. Hypotheses, 2014, 83, 822–824 CrossRef.
- Organ Donation https://www.organdonation.nhs.uk/news-and-campaigns/news/nhs-blo/, 2018.
- A. Shah, J. Brugnano, S. Sun, A. Vase and E. Orwin, Pediatr. Res., 2008, 63, 535–544 CrossRef.
- B. Kong and S. Mi, Materials, 2016, 9, 614 CrossRef.
- M. Brown and D. Farrar, Plast., Rubber Compos., 2008, 37, 46–49 CrossRef CAS.
-
C. Chu, Biodegradable Polymeric Biomaterials: An Updated Overview, CRC Press, Boca Raton, 2003 Search PubMed.
- P. Deshpande, I. Ortega, F. Sefat, V. Sangwan, N. Green, F. Claeyssens and S. MacNeil, Invest. Ophthalmol. Visual Sci., 2015, 56(3), 1553–1561 CrossRef CAS.
-
J. Park and R. Lakes, Biomaterials, Plenum Press, New York, 1992 Search PubMed.
-
P. Deshpande, C. Ramachandran, V. S. Sangwan and S. MacNeil, in Cultivation of limbal epithelial cells on electrospun poly (lactide-co-glycolide) scaffolds for delivery to the cornea.ed. editors, Springer, edition, 2013, pp. 179–185 Search PubMed.
- F. Sefat, R. McKean, P. Deshpande, C. Ramachandran, C. J. Hill, V. S. Sangwan, A. J. Ryan and S. MacNeil, Procedia Eng., 2013, 59, 101–116 CrossRef CAS.
- D. Phu, L. S. Wray, R. V. Warren, R. C. Haskell and E. J. Orwin, Tissue Eng., Part A, 2011, 17, 799–807 CrossRef CAS.
- N. E. Vrana, N. Builles, V. Justin, J. Bednarz, G. Pellegrini, B. Ferrari, O. Damour, D. J. Hulmes and V. Hasirci, Invest. Ophthalmol. Visual Sci., 2008, 49, 5325–5331 CrossRef.
- P. Bhattacharjee, B. L. Cavanagh and M. Ahearne, Sci. Rep., 2020, 10, 1–11 CrossRef.
- T. M. da Mata Martins, P. da Silva Cunha, M. A. Rodrigues, J. L. de Carvalho, J. E. de Souza, J. A. de Carvalho Oliveira, D. A. Gomes and A. M. de Goes, Mater. Sci. Eng., C, 2020, 116, 111215 CrossRef CAS.
- M. Ahearne, J. Fernández-Pérez, S. Masterton, P. W. Madden and P. Bhattacharjee, Adv. Funct. Mater., 2020, 1908996 CrossRef CAS.
- J. I. Kim, J. Y. Kim and C. H. Park, Sci. Rep., 2018, 8, 1–13 CrossRef.
- G. Benedek, Appl. Opt., 1971, 10, 459–473 CrossRef CAS.
- J. W. Ruberti and J. D. Zieske, Prog. Retinal Eye Res., 2008, 27, 549–577 CrossRef CAS.
- Z. Wu, B. Kong, R. Liu, W. Sun and S. Mi, Nanomaterials, 2018, 8, 124 CrossRef.
- H. Bakhshandeh, M. Soleimani, S. S. Hosseini, H. Hashemi, I. Shabani, A. Shafiee, A. H. B. Nejad, M. Erfan, R. Dinarvand and F. Atyabi, Int. J. Nanomed., 2011, 6, 1509 CAS.
-
C. Krishna Murthy and B. Mandel, Biomaterials Based on Natural and Synthetic Polymer Fibers, Pan Stanford Publishing, Danvers, 2016 Search PubMed.
- M. L. Borene, V. H. Barocas and A. Hubel, Ann. Biomed. Eng., 2004, 32, 274–283 CrossRef.
- Z. Chen, J. You, X. Liu, S. Cooper, C. Hodge, G. Sutton, J. M. Crook and G. G. Wallace, Biomed. Mater., 2018, 13, 032002 CrossRef.
- A. Karkhaneh, H. Mirzadeh, A. Ghaffariyeh, A. Ebrahimi, N. Honarpisheh, M. Hosseinzadeh and M. H. Heidari, Br. J. Ophthalmol., 2011, 95, 405–409 CrossRef.
- D. Liu, M. Nikoo, G. Boran, P. Zhou and J. M. Regenstein, Annu. Rev. Food Sci. Technol., 2015, 6, 527–557 CrossRef CAS.
- W. McIntosh Ambrose, A. Salahuddin, S. So, S. Ng, S. Ponce Márquez, T. Takezawa, O. Schein and J. Elisseeff, J. Biomed. Mater. Res., Part B, 2009, 90, 818–831 CrossRef.
- M. Rafat, F. Li, P. Fagerholm, N. S. Lagali, M. A. Watsky, R. Munger, T. Matsuura and M. Griffith, Biomaterials, 2008, 29, 3960–3972 CrossRef CAS.
- S. Salehi, M. Fathi, S. H. Javanmard, T. Bahners, J. S. Gutmann, S. Ergün, K. P. Steuhl and T. A. Fuchsluger, Macromol. Mater. Eng., 2014, 299, 455–469 CrossRef CAS.
- R. Watanabe, R. Hayashi, Y. Kimura, Y. Tanaka, T. Kageyama, S. Hara, Y. Tabata and K. Nishida, Tissue Eng., Part A, 2011, 17, 2213–2219 CrossRef CAS.
- L. Lin and X. Jin, Ann. Eye Sci., 2018, 3, 6 CrossRef.
- Y. Liu, H. Lv, L. Ren, G. Xue and Y. Wang, J. Biomater. Sci., Polym. Ed., 2016, 27, 758–772 CrossRef CAS.
- W. Xu, Z. Wang, Y. Liu, L. Wang, Z. Jiang, T. Li, W. Zhang and Y. Liang, Carbohydr. Polym., 2018, 192, 240–250 CrossRef CAS.
- C. E. Ghezzi, B. Marelli, F. G. Omenetto, J. L. Funderburgh and D. L. Kaplan, PLoS One, 2017, 12, e0169504 CrossRef.
- E. S. Gil, B. B. Mandal, S.-H. Park, J. K. Marchant, F. G. Omenetto and D. L. Kaplan, Biomaterials, 2010, 31, 8953–8963 CrossRef CAS.
- B. D. Lawrence, J. K. Marchant, M. A. Pindrus, F. G. Omenetto and D. L. Kaplan, Biomaterials, 2009, 30, 1299–1308 CrossRef CAS.
- T. Mikami and H. Kitagawa, Biochim. Biophys. Acta, Gen. Subj., 2013, 1830, 4719–4733 CrossRef CAS.
- S. P. Márquez, V. S. Martínez, W. M. Ambrose, J. Wang, N. G. Gantxegui, O. Schein and J. Elisseeff, Acta Biomater., 2009, 5, 1839–1847 CrossRef.
- Y.-g. Xu, Y.-s. Xu, C. Huang, Y. Feng, Y. Li and W. Wang, Mol. Vision, 2008, 14, 2180 CAS.
- C. Zhang, X. Nie, D. Hu, Y. Liu, Z. Deng, R. Dong, Y. Zhang and Y. Jin, Cell Tissue Res., 2007, 329, 249–257 CrossRef.
-
H. Bang Lee, G. Khang and J. Ho Lee, in Polymeric Biomaterials, CRC Press, Boca Raton, 2003, pp. 55–73 Search PubMed.
- S. Rimmer, C. Johnson, B. Zhao, J. Collier, L. Gilmore, S. Sabnis, P. Wyman, C. Sammon, N. J. Fullwood and S. MacNeil, Biomaterials, 2007, 28, 5319–5331 CrossRef CAS.
- Í. Ortega, A. J. Ryan, P. Deshpande, S. MacNeil and F. Claeyssens, Acta Biomater., 2013, 9, 5511–5520 CrossRef.
- G. R. Da Silva, T. H. Lima, R. L. Oréfice, G. M. Fernandes-Cunha, A. Silva-Cunha, M. Zhao and F. Behar-Cohen, Eur. J. Pharm. Sci., 2015, 73, 9–19 CrossRef CAS.
- S. Sharma, D. Gupta, S. Mohanty, M. Jassal, A. K. Agrawal and R. Tandon, Invest. Ophthalmol. Visual Sci., 2014, 55, 899–907 CrossRef CAS.
- S. Sharma, S. Mohanty, D. Gupta, M. Jassal, A. K. Agrawal and R. Tandon, Mol. Vision, 2011, 17, 2898 CAS.
- C. Tang, C. D. Saquing, J. R. Harding and S. A. Khan, Macromolecules, 2010, 43, 630–637 CrossRef CAS.
- R. Rai, M. Tallawi, A. Grigore and A. R. Boccaccini, Prog. Polym. Sci., 2012, 37, 1051–1078 CrossRef CAS.
- C. Zhang, J. Wen, J. Yan, Y. Kao, Z. Ni, X. Cui and H. Wang, RSC Adv., 2015, 5, 12123–12130 RSC.
- S. Lanzalaco and E. Armelin, Gels, 2017, 3, 36 CrossRef.
- L. H. Lima, Y. Morales and T. Cabral, J. Ophthalmol., 2016, 2016, 5356371 Search PubMed.
- B. K. Madathil, P. R. Anil Kumar and T. V. Kumary, BioMed Res. Int., 2014, 2014, 450672 CrossRef.
- K. Tonsomboon and M. L. Oyen, J. Mech. Behav. Biomed. Mater., 2013, 21, 185–194 CrossRef CAS.
- J. Torbet, M. Malbouyres, N. Builles, V. Justin, M. Roulet, O. Damour, Å. Oldberg, F. Ruggiero and D. J. Hulmes, Biomaterials, 2007, 28, 4268–4276 CrossRef CAS.
- A. Acun and V. Hasirci, J. Biomater. Sci., Polym. Ed., 2014, 25, 1110–1132 CrossRef CAS.
- F. Li, D. Carlsson, C. Lohmann, E. Suuronen, S. Vascotto, K. Kobuch, H. Sheardown, R. Munger, M. Nakamura and M. Griffith, Proc. Natl. Acad. Sci. U. S. A., 2003, 100, 15346–15351 CrossRef CAS.
- S. Salehi, M. Czugala, P. Stafiej, M. Fathi, T. Bahners, J. S. Gutmann, B. B. Singer and T. A. Fuchsluger, Acta Biomater., 2017, 50, 370–380 CrossRef CAS.
- T. Fan, X. Ma, J. Zhao, Q. Wen, X. Hu, H. Yu and W. Shi, Mol. Vision, 2013, 19, 400 CAS.
- P. Fagerholm, N. S. Lagali, K. Merrett, W. B. Jackson, R. Munger, Y. Liu, J. W. Polarek, M. Söderqvist and M. Griffith, Sci. Transl. Med., 2010, 2, 46ra61–46ra61 Search PubMed.
- Z. Arabpour, A. Baradaran-Rafii, N. L. Bakhshaiesh, J. Ai, S. Ebrahimi–Barough, H. Esmaeili Malekabadi, N. Nazeri, A. Vaez, M. Salehi and F. Sefat, J. Biomed. Mater. Res.,
Part A, 2019, 107, 2340–2349 CrossRef CAS.
- J. Chen, C. Yan, M. Zhu, Q. Yao, C. Shao, W. Lu, J. Wang, X. Mo, P. Gu and Y. Fu, Int. J. Nanomed., 2015, 10, 3337 CAS.
- Í. Ortega, F. Sefat, P. Deshpande, T. Paterson, C. Ramachandran, A. J. Ryan, S. MacNeil and F. Claeyssens, J. Visualized Exp., 2014, e51826 Search PubMed.
- J. Doshi and D. H. Reneker, J. Electrost., 1995, 35, 151–160 CrossRef CAS.
- Z. Chen, X. Liu, J. You, Y. Song, E. Tomaskovic-Crook, G. Sutton, J. M. Crook and G. G. Wallace, Acta Biomater., 2020, 113, 360–371 CrossRef CAS.
- H. Miyashita, S. Shimmura, H. Kobayashi, T. Taguchi, N. Asano-Kato, Y. Uchino, M. Kato, J. Shimazaki, J. Tanaka and K. Tsubota, J. Biomed. Mater. Res., Part B, 2006, 76, 56–63 CrossRef.
-
G. Verma and P. Hassan, Self-Assembly Approach for Biomaterials Development, Pan Stanford Publishing, Danvers, 2016 Search PubMed.
- S. Proulx, J. d. A. Uwamaliya, P. Carrier, A. Deschambeault, C. Audet, C. J. Giasson, S. L. Guérin, F. A. Auger and L. Germain, Mol. Vision, 2010, 16, 2192 CAS.
- S. Jabbehdari, G. Yazdanpanah, L. N. Kanu, E. Chen, K. Kang, K. N. Anwar, M. Ghassemi, P. Hematti, M. I. Rosenblatt and A. R. Djalilian, Curr. Eye Res., 2020, 1–7 Search PubMed.
- M. Fuest, G. H.-F. Yam, J. S. Mehta and D. F. Duarte Campos, Bioengineering, 2020, 7, 71 CrossRef.
- C. K. Bektas and V. Hasirci, Biomater. Sci., 2020, 8, 438–449 RSC.
- A. Sorkio, L. Koch, L. Koivusalo, A. Deiwick, S. Miettinen, B. Chichkov and H. Skottman, Biomaterials, 2018, 171, 57–71 CrossRef CAS.
- Z. Wu, X. Su, Y. Xu, B. Kong, W. Sun and S. Mi, Sci. Rep., 2016, 6, 1–10 CrossRef.
- F. N. Syed-Picard, Y. Du, A. J. Hertsenberg, R. Palchesko, M. L. Funderburgh, A. W. Feinberg and J. L. Funderburgh, J. Tissue Eng. Regener. Med., 2018, 12, 59–69 CrossRef CAS.
- S. S. Mahdavi, M. J. Abdekhodaie, S. Mashayekhan, A. Baradaran-Rafii and A. R. Djalilian, Tissue Eng. Regener. Med., 2020, 17, 1–27 CrossRef.
- P. Deshpande, C. Ramachandran, F. Sefat, I. Mariappan, C. Johnson, R. McKean, M. Hannah, V. S. Sangwan, F. Claeyssens and A. J. Ryan, Biomaterials, 2013, 34, 5088–5106 CrossRef CAS.
- S. Mishima and B. O. Hedbys, Arch. Ophthalmol., 1968, 80, 710–713 CrossRef CAS.
- Y. Zhao, D. Chen, G. Savini, Q. Wang, H. Zhang, Y. Jin, B. Song, R. Ning, J. Huang and C. Mei, Eye Vision, 2020, 7, 1–12 Search PubMed.
- Q. Liang, Q. Le, D. W. Cordova, C.-H. Tseng and S. X. Deng, Am. J. Ophthalmol., 2020, 216, 132–139 CrossRef.
- M. Sacchetti, P. Rama, A. Bruscolini and A. Lambiase, Stem Cells Int., 2018, 2018, 8086269 Search PubMed.
- C. E. Ghezzi, J. Rnjak-Kovacina and D. L. Kaplan, Tissue Eng., Part B, 2015, 21, 278–287 CrossRef.
- D. J. Szabó, A. Noer, R. Nagymihály, N. Josifovska, S. Andjelic, Z. Veréb, A. Facskó, M. C. Moe and G. Petrovski, PLoS One, 2015, 10, e0143053 CrossRef.
- J. L. A. del Barrio and J. L. Alió, Eye Vision, 2018, 5, 28 CrossRef.
- B. Demirayak, N. Yüksel, O. S. Çelik, C. Subaşı, G. Duruksu, Z. S. Unal, D. K. Yıldız and E. Karaöz, Exp. Eye Res., 2016, 151, 227–235 CrossRef CAS.
- V. J. Coulson-Thomas, B. Caterson and W. W. Y. Kao, Stem Cells, 2013, 31, 2116–2126 CrossRef CAS.
- H. Liu, J. Zhang, C.-Y. Liu, I.-J. Wang, M. Sieber, J. Chang, J. V. Jester and W. W. Kao, PLoS One, 2010, 5, e10707 CrossRef.
- J. D. West, R. L. Mort, R. E. Hill, S. D. Morley and J. M. Collinson, Stem Cell Res., 2018, 30, 1–11 CrossRef.
- S. S. Aceves and S. J. Ackerman, Immunol. Allergy Clin. North Am., 2009, 29, 197–211 CrossRef.
- C. Bonnans, J. Chou and Z. Werb, Nat. Rev. Mol. Cell Biol., 2014, 15, 786–801 CrossRef CAS.
- M. Notara, A. Alatza, J. Gilfillan, A. Harris, H. Levis, S. Schrader, A. Vernon and J. Daniels, Exp. Eye Res., 2010, 90, 188–195 CrossRef CAS.
- T. Ramaesh, K. Ramaesh, J. M. Collinson, S. A. Chanas, B. Dhillon and J. D. West, Exp. Eye Res., 2005, 81, 224–235 CrossRef CAS.
- F. Arnalich-Montiel, S. Pastor, A. Blazquez-Martinez, J. Fernandez-Delgado, M. Nistal, J. L. Alio and M. P. De Miguel, Stem Cells, 2008, 26, 570–579 CrossRef CAS.
- M. P. De Miguel, R. P. Casaroli-Marano, N. Nieto-Nicolau, E. M. Martínez-Conesa, J. L. A. del Barrio, J. L. Alió, S. Fuentes and F. Arnalich-Montiel, Front. Stem Cell Regen. Med. Res., 2015, 1, 92–138 Search PubMed.
- V. Holan, P. Trosan, C. Cejka, E. Javorkova, A. Zajicova, B. Hermankova, M. Chudickova and J. Cejkova, Stem Cells Transl. Med., 2015, 4, 1052–1063 CrossRef CAS.
- S. K. Mittal, M. Omoto, A. Amouzegar, A. Sahu, A. Rezazadeh, K. R. Katikireddy, D. I. Shah, S. K. Sahu and S. K. Chauhan, Stem Cell Rep., 2016, 7, 583–590 CrossRef CAS.
- J. Zhu, M. Slevin, B.-Q. Guo and S.-R. Zhu, Int. J. Ophthalmol., 2018, 11, 2004 Search PubMed.
- D. Karamichos, A. Hutcheon, B. Saitta and J. Zieske, Invest. Ophthalmol. Visual Sci., 2010, 51, 6217 Search PubMed −.
- H.-T. Xie, D. Zhao, Y. Liu and M.-C. Zhang, J. Ophthalmol., 2017, 2017, 2767053 Search PubMed.
- M. Ziaei, J. Zhang, D. V. Patel and C. N. McGhee, Surv. Ophthalmol., 2017, 62, 803–815 CrossRef.
- L. Espandar, B. Bunnell, G. Y. Wang, P. Gregory, C. McBride and M. Moshirfar, Arch. Ophthalmol., 2012, 130, 202–208 CrossRef CAS.
- Z. Wu, Q. Zhou, H. Duan, X. Wang, J. Xiao, H. Duan, N. Li, C. Li, P. Wan and Y. Liu, PLoS One, 2014, 9, e93012 CrossRef.
- P. Carrier, A. Deschambeault, C. Audet, M. v. Talbot, R. Gauvin, C. J. Giasson, F. o. A. Auger, S. L. Guérin and L. Germain, Invest. Ophthalmol. Visual Sci., 2009, 50, 2645–2652 CrossRef.
- A. Faulkner-Jones, C. Fyfe, D.-J. Cornelissen, J. Gardner, J. King, A. Courtney and W. Shu, Biofabrication, 2015, 7, 044102 CrossRef.
- Q. Gu, E. Tomaskovic-Crook, G. G. Wallace and J. M. Crook, Adv. Healthcare Mater., 2017, 6, 1700175 CrossRef.
- P. Ordonez and N. Di Girolamo, Stem Cells, 2012, 30, 100–107 CrossRef CAS.
- M. F. Goldberg and A. J. Bron, Trans. Am. Ophthalmol. Soc., 1982, 80, 155 CAS.
- T.-T. Sun, S. C. Tseng and R. M. Lavker, Nature, 2010, 463, E10–EE1 CrossRef CAS.
- C.-Y. Chang, C. R. Green, C. N. McGhee and T. Sherwin, Invest. Ophthalmol. Visual Sci., 2008, 49, 5279–5286 CrossRef.
- C. Burillon, L. Huot, V. Justin, S. Nataf, F. Chapuis, E. Decullier and O. Damour, Invest. Ophthalmol. Visual Sci., 2012, 53, 1325–1331 CrossRef.
- G. Pellegrini, O. Golisano, P. Paterna, A. Lambiase, S. Bonini, P. Rama and M. De Luca, J. Cell Biol., 1999, 145, 769–782 CrossRef CAS.
- H. Qi, X. Zheng, X. Yuan, S. C. Pflugfelder and D. Q. Li, J. Cell. Physiol., 2010, 225, 180–185 CrossRef CAS.
- V. S. Sangwan, G. K. Vemuganti, S. Singh and D. Balasubramanian, Biosci. Rep., 2003, 23, 169–174 CrossRef CAS.
- I. Kerkis, A. Kerkis, D. Dozortsev, G. C. Stukart-Parsons, S. M. G. Massironi, L. V. Pereira, A. I. Caplan and H. F. Cerruti, Cells Tissues Organs, 2006, 184, 105–116 CrossRef CAS.
- B. Monteiro, R. Serafim, G. Melo, M. Silva, N. Lizier, C. Maranduba, R. Smith, A. Kerkis, H. Cerruti and J. Gomes, Cell Proliferation, 2009, 42, 587–594 CrossRef CAS.
- C. A. Jahoda, C. J. Whitehouse, A. J. Reynolds and N. Hole, Exp. Dermatol., 2003, 12, 849–859 CrossRef.
- E. A. Blazejewska, U. Schlötzer-Schrehardt, M. Zenkel, B. Bachmann, E. Chankiewitz, C. Jacobi and F. E. Kruse, Stem Cells, 2009, 27, 642–652 CrossRef CAS.
- E. A. Meyer-Blazejewska, M. K. Call, O. Yamanaka, H. Liu, U. Schlötzer-Schrehardt, F. E. Kruse and W. W. Kao, Stem Cells, 2011, 29, 57–66 CrossRef CAS.
- T. Nakamura, K. i. Endo and S. Kinoshita, Stem Cells, 2007, 25, 628–638 CrossRef CAS.
- T. Inatomi, T. Nakamura, M. Kojyo, N. Koizumi, C. Sotozono and S. Kinoshita, Am. J. Ophthalmol., 2006, 142, 757–764 CrossRef.
- M. Yamato, M. Utsumi, A. Kushida, C. Konno, A. Kikuchi and T. Okano, Tissue Eng., 2001, 7, 473–480 CrossRef CAS.
- M. F. Pittenger, A. M. Mackay, S. C. Beck, R. K. Jaiswal, R. Douglas, J. D. Mosca, M. A. Moorman, D. W. Simonetti, S. Craig and D. R. Marshak, Science, 1999, 284, 143–147 CrossRef CAS.
- Y. Jiang, B. N. Jahagirdar, R. L. Reinhardt, R. E. Schwartz, C. D. Keene, X. R. Ortiz-Gonzalez, M. Reyes, T. Lenvik, T. Lund and M. Blackstad, Nature, 2002, 418, 41–49 CrossRef CAS.
- D. S. Krause, N. D. Theise, M. I. Collector, O. Henegariu, S. Hwang, R. Gardner, S. Neutzel and S. J. Sharkis, Cell, 2001, 105, 369–377 CrossRef CAS.
- Y. Ma, Y. Xu, Z. Xiao, W. Yang, C. Zhang, E. Song, Y. Du and L. Li, Stem Cells, 2006, 24, 315–321 CrossRef.
- J. Ye, S. Y. Lee, K. H. KooK and K. Yao, Graefe's Arch. Clin. Exp. Ophthalmol., 2008, 246, 217–222 CrossRef.
- J. Ye, K. Yao and J. Kim, Eye, 2006, 20, 482–490 CrossRef CAS.
- Y. Lan, S. Kodati, H. S. Lee, M. Omoto, Y. Jin and S. K. Chauhan, Invest. Ophthalmol. Visual Sci., 2012, 53, 3638–3644 CrossRef CAS.
- Y. Du, D. S. Roh, M. L. Funderburgh, M. M. Mann, K. G. Marra, J. P. Rubin, X. Li and J. L. Funderburgh, Mol. Vision, 2010, 16, 2680 CAS.
- E. Collomb, Y. Yang, S. Foriel, S. Cadau, D. J. Pearton and D. Dhouailly, Dev. Dyn., 2013, 242, 401–413 CrossRef CAS.
- S. Ahmad, R. Stewart, S. Yung, S. Kolli, L. Armstrong, M. Stojkovic, F. Figueiredo and M. Lako, Stem Cells, 2007, 25, 1145–1155 CrossRef CAS.
- J. Brzeszczynska, K. Samuel, S. Greenhough, K. Ramaesh, B. Dhillon, D. Hay and J. Ross, Int. J. Mol. Med., 2014, 33, 1597–1606 CrossRef CAS.
- R. Hayashi, Y. Ishikawa, M. Ito, T. Kageyama, K. Takashiba, T. Fujioka, M. Tsujikawa, H. Miyoshi, M. Yamato and Y. Nakamura, PLoS One, 2012, 7, e45435 CrossRef CAS.
- A. Cieślar-Pobuda, M. Rafat, V. Knoflach, M. Skonieczna, A. Hudecki, A. Małecki, E. Urasińska, S. Ghavami and M. J. Łos, Oncotarget, 2016, 7, 42314 CrossRef.
- H. Hongisto, T. Ilmarinen, M. Vattulainen, A. Mikhailova and H. Skottman, Stem Cell Res. Ther., 2017, 8, 291 CrossRef.
- T. A. Kamarudin, S. Bojic, J. Collin, M. Yu, S. Alharthi, H. Buck, A. Shortt, L. Armstrong, F. C. Figueiredo and M. Lako, Stem Cells, 2018, 36, 337–348 CrossRef CAS.
- A. Mikhailova, T. Ilmarinen, H. Uusitalo and H. Skottman, Stem Cell Rep., 2014, 2, 219–231 CrossRef CAS.
- H. Vaajasaari, T. Ilmarinen, K. Juuti-Uusitalo, K. Rajala, N. Onnela, S. Narkilahti, R. Suuronen, J. Hyttinen, H. Uusitalo and H. Skottman, Mol. Vision, 2011, 17, 558 CAS.
- K. Araki-Sasaki, Y. Ohashi, T. Sasabe, K. Hayashi, H. Watanabe, Y. Tano and H. Handa, Invest. Ophthalmol. Visual Sci., 1995, 36, 614–621 CAS.
- M. Poli, C. Burillon, C. Auxenfans, M.-R. Rovere and O. Damour, Cornea, 2015, 34, 817–823 CrossRef.
- M. Nishizawa, K. Chonabayashi, M. Nomura, A. Tanaka, M. Nakamura, A. Inagaki, M. Nishikawa, I. Takei, A. Oishi and K. Tanabe, Cell Stem Cell, 2016, 19, 341–354 CrossRef CAS.
- J. Schuster, J. Halvardson, L. Pilar Lorenzo, A. Ameur, M. Sobol, D. Raykova, G. Annerén, L. Feuk and N. Dahl, Cell. Reprogram., 2015, 17, 327–337 CrossRef CAS.
- A. Kim, N. Lakshman, D. Karamichos and W. M. Petroll, Invest. Ophthalmol. Visual Sci., 2010, 51, 864–875 CrossRef.
- B. J. Tripathi, P. S. Kwait and R. C. Tripathi, Cornea, 1990, 9, 2–9 CrossRef CAS.
- H. Jing, X. He and J. Zheng, Transl. Res., 2018, 196, 1–16 CrossRef CAS.
- Z. Jiang, G. Liu, F. Meng, W. Wang, P. Hao, Y. Xiang, Y. Wang, R. Han, F. Li and L. Wang, Br. J. Ophthalmol., 2017, 101, 1583–1590 CrossRef.
- N. Singh, M. Tiem, R. Watkins, Y. K. Cho, Y. Wang, T. Olsen, H. Uehara, C. Mamalis, L. Luo and Z. Oakey, Blood, 2013, 121, 4242–4249 CrossRef CAS.
- X. Feng, L. Pi, S. Sriram, G. S. Schultz and D. J. Gibson, PLoS One, 2017, 12, e0172304 CrossRef.
- H. Miyagi, S. M. Thomasy, P. Russell and C. J. Murphy, Exp. Eye Res., 2018, 166, 49–55 CrossRef CAS.
- M. Omoto, K. Suri, A. Amouzegar, M. Li, K. R. Katikireddy, S. K. Mittal and S. K. Chauhan, Mol. Ther., 2017, 25, 1881–1888 CrossRef CAS.
- T. D. Blalock, M. R. Duncan, J. C. Varela, M. H. Goldstein, S. S. Tuli, G. R. Grotendorst and G. S. Schultz, Invest. Ophthalmol. Visual Sci., 2003, 44, 1879–1887 CrossRef.
- C. Chen, B. Michelini-Norris, S. Stevens, J. Rowsey, X.-o. Ren, M. Goldstein and G. Schultz, Invest. Ophthalmol. Visual Sci., 2000, 41, 4108–4116 CAS.
- M. F. Cordeiro, M. B. Reichel, J. A. Gay, F. D'Esposita, R. A. Alexander and P. T. Khaw, Invest. Ophthalmol. Visual Sci., 1999, 40, 1975–1982 CAS.
- V. B. Leclerc, O. Roy, K. Santerre and S. Proulx, Sci. Rep., 2018, 8, 1–15 CrossRef.
- Y. H. Yang, T. L. Hsieh, A. T. Q. Ji, W. T. Hsu, C. Y. Liu, O. K. S. Lee and J. H. C. Ho, Stem Cells, 2016, 34, 2525–2535 CrossRef CAS.
- L. Pi, A. K. Shenoy, J. Liu, S. Kim, N. Nelson, H. Xia, W. W. Hauswirth, B. E. Petersen, G. S. Schultz and E. W. Scott, FASEB J., 2012, 26, 3365–3379 CrossRef CAS.
- A. Meduri, P. Aragona, P. L. Grenga and A. M. Roszkowska, J. Refractive Surg., 2012, 28, 220–223 CrossRef.
- N. Yamada, R. Matsuda, N. Morishige, R. Yanai, T. Chikama, T. Nishida, T. Ishimitsu and A. Kamiya, Br. J. Ophthalmol., 2008, 92, 896–900 CrossRef CAS.
- J. C. Pastor and M. Calonge, Cornea, 1992, 11, 311–314 CrossRef CAS.
- G. S. Schultz, J. B. Davis and R. A. Eiferman, Cornea, 1988, 7, 96–101 CrossRef CAS.
- K.-Y. Han, J. A. Tran, J.-H. Chang, D. T. Azar and J. D. Zieske, Sci. Rep., 2017, 7, 40548 CrossRef CAS.
- R. Samaeekia, B. Rabiee, I. Putra, X. Shen, Y. J. Park, P. Hematti, M. Eslani and A. R. Djalilian, Invest. Ophthalmol. Visual Sci., 2018, 59, 5194–5200 CrossRef CAS.
- F. J. Vizoso, N. Eiro, S. Cid, J. Schneider and R. Perez-Fernandez, Int. J. Mol. Sci., 2017, 18, 1852 CrossRef.
- S. R. Baglio, D. M. Pegtel and N. Baldini, Front. Physiol., 2012, 3, 359 Search PubMed.
- M. Madrigal, K. S. Rao and N. H. Riordan, J. Transl. Med., 2014, 12, 1–14 CrossRef.
- G. Maguire, Commun. Integr. Biol., 2013, 6, e26631 CrossRef.
- N. Sevivas, F. G. Teixeira, R. Portugal, L. Araújo, L. F. Carriço, N. Ferreira, M. Vieira da Silva, J. Espregueira-Mendes, S. Anjo and B. Manadas, Am. J. Sports Med., 2017, 45, 179–188 CrossRef.
- G. Lou, Z. Chen, M. Zheng and Y. Liu, Exp. Mol. Med., 2017, 49, e346 CrossRef.
- S. Rani, A. E. Ryan, M. D. Griffin and T. Ritter, Mol. Ther., 2015, 23, 812–823 CrossRef CAS.
- B. Yu, X. Zhang and X. Li, Int. J. Mol. Sci., 2014, 15, 4142–4157 CrossRef CAS.
- L. Bai, H. Shao, H. Wang, Z. Zhang, C. Su, L. Dong, B. Yu, X. Chen, X. Li and X. Zhang, Sci. Rep., 2017, 7, 1–11 CrossRef.
-
R. C. Lai, R. W. Y. Yeo and S. K. Lim, Mesenchymal stem cell exosomes. Seminars in cell & developmental biology, 2015, Elsevier Search PubMed.
- J. Zhang, J. Guan, X. Niu, G. Hu, S. Guo, Q. Li, Z. Xie, C. Zhang and Y. Wang, J. Transl. Med., 2015, 13, 49 CrossRef.
- B. Yu, H. Shao, C. Su, Y. Jiang, X. Chen, L. Bai, Y. Zhang, Q. Li, X. Zhang and X. Li, Sci. Rep., 2016, 6, 34562 CrossRef CAS.
- T. Shen, Q.-Q. Zheng, J. Shen, Q.-S. Li, X.-H. Song, H.-B. Luo, C.-Y. Hong and K. Yao, Chin. Med. J., 2018, 131, 704 CrossRef.
-
J. Wahlgren, L. Statello, G. Skogberg, E. Telemo and H. Valadi, Delivery of small interfering RNAs to cells via exosomes, Springer, 2016 Search PubMed.
- Measuring the Size & Surface Charge of Exosomes, https://www.brookhaveninstruments.com/library/micelles-liposomes-exosomes/measuring-the-size-and-surface-charge-of-exosomes-microvesicles-and-liposomes, 2018.
-
C. Whitbeck, Ethics in engineering practice and research, Cambridge University Press, Cambridge, 1998 Search PubMed.
- S. H. Woolf, J. Am. Med. Assoc., 2008, 299, 211–213 CAS.
- F. C. Fang and A. Casadevall, Infect. Immun., 2010, 78, 563–566 CrossRef CAS.
- B. E. Knox, J. Ophthalmic Vision Res., 2012, 7, 1 Search PubMed.
- D. F. Duarte Campos, M. Rohde, M. Ross, P. Anvari, A. Blaeser, M. Vogt, C. Panfil, G. H. F. Yam, J. S. Mehta and H. Fischer, J. Biomed. Mater. Res., Part A, 2019, 107, 1945–1953 CrossRef CAS.
- B. Zhang, Q. Xue, H.-y. Hu, M.-f. Yu, L. Gao, Y.-c. Luo, Y. Li, J.-t. Li, L. Ma and Y.-f. Yao, J. Zhejiang Univ., Sci., B, 2019, 20, 945–959 CrossRef CAS.
- K. W. Kim, S. J. Lee, S. H. Park and J. C. Kim, Adv. Healthcare Mater., 2018, 7, 1800398 CrossRef.
- A. Tan, D. T. Tan, X.-W. Tan and J. S. Mehta, Ocul. Surf., 2012, 10, 15–25 CrossRef.
- P. Rama, S. Bonini, A. Lambiase, O. Golisano, P. Paterna, M. De Luca and G. Pellegrini, Transplantation, 2001, 72, 1478–1485 CrossRef CAS.
- P. Rama, S. Matuska, G. Paganoni, A. Spinelli, M. De Luca and G. Pellegrini, N. Engl. J. Med., 2010, 363, 147–155 CrossRef CAS.
- B. Aghaei-Ghareh-Bolagh, J. Guan, Y. Wang, A. D. Martin, R. Dawson, S. M. Mithieux and A. S. Weiss, Biomaterials, 2019, 188, 50–62 CrossRef CAS.
- A. Galal, J. J. Perez-Santonja, J. L. Rodriguez-Prats, M. Abad and J. Alio, Cornea, 2007, 26, 473–478 CrossRef.
- M. Borrelli, S. Reichl, Y. Feng, M. Schargus, S. Schrader and G. Geerling, J. Mater. Sci.: Mater. Med., 2013, 24, 221–230 CrossRef CAS.
-
T. de By, Shortage in the face of plenty: improving the allocation of corneas for transplantation, Karger Publishers, 2003 Search PubMed.
- M. Van Meter, W. Spears and P. H. Sheth, Int. J. Eye Bank., 2013, 1, 1–9 Search PubMed.
- A. de la Mata, T. Nieto-Miguel, M. López-Paniagua, S. Galindo, M. R. Aguilar, L. García-Fernández, S. Gonzalo, B. Vázquez, J. San Román and R. M. Corrales, J. Mater. Sci.: Mater. Med., 2013, 24, 2819–2829 CrossRef CAS.
- E. Bentley, C. J. Murphy, F. Li, D. J. Carlsson and M. Griffith, Cornea, 2010, 29 DOI:10.1097/ICO.0b013e3181c846aa.
- A. M. Oelker and M. W. Grinstaff, IEEE Trans. Nanobiosci., 2011, 11, 37–45 Search PubMed.
- X. W. Tan, L. Hartman, K. P. Tan, R. Poh, D. Myung, L. L. Zheng, D. Waters, J. Noolandi, R. W. Beuerman and C. W. Frank, J. Mater. Sci.: Mater. Med., 2013, 24, 967–977 CrossRef CAS.
- L. Hartmann, K. Watanabe, L. L. Zheng, C. Y. Kim, S. E. Beck, P. Huie, J. Noolandi, J. R. Cochran, C. N. Ta and C. W. Frank, J. Biomed. Mater. Res., Part B, 2011, 98, 8–17 CrossRef.
- J.-Y. Lai, Y.-T. Li, C.-H. Cho and T.-C. Yu, Int. J. Nanomed., 2012, 7, 1101 CrossRef CAS.
- X.-Y. Ma, H.-J. Bao, L. Cui and J. Zou, PLoS One, 2013, 8, e76103 CrossRef CAS.
- M. M. Islam, O. Buznyk, J. C. Reddy, N. Pasyechnikova, E. I. Alarcon, S. Hayes, P. Lewis, P. Fagerholm, C. He and S. Iakymenko, npj Regener. Med., 2018, 3, 1–10 CrossRef.
- R. M. Gouveia, E. Koudouna, J. Jester, F. Figueiredo and C. J. Connon, Adv. Biosyst., 2017, 1, 1700135 CrossRef.
- M. Miotto, R. M. Gouveia, A. M. Ionescu, F. Figueiredo, I. W. Hamley and C. J. Connon, Adv. Funct. Mater., 2019, 29, 1807334 CrossRef.
- S. L. Wilson, L. E. Sidney, S. E. Dunphy, H. S. Dua and A. Hopkinson, Curr. Eye Res., 2016, 41, 769–782 CrossRef CAS.
- M. González-Andrades, V. Carriel, M. Rivera-Izquierdo, I. Garzón, E. González-Andrades, S. Medialdea, M. Alaminos and A. Campos, Transl. Vision Sci. Technol., 2015, 4, 13 CrossRef.
- A. Porzionato, E. Stocco, S. Barbon, F. Grandi, V. Macchi and R. De Caro, Int. J. Mol. Sci., 2018, 19, 4117 CrossRef.
- S. L. Wilson, L. E. Sidney, S. E. Dunphy, J. B. Rose and A. Hopkinson, J. Funct. Biomater., 2013, 4, 114–161 CrossRef.
- J. Fernández-Pérez and M. Ahearne, Methods, 2020, 171, 86–96 CrossRef.
- S. Kinoshita, N. Koizumi, M. Ueno, N. Okumura, K. Imai, H. Tanaka, Y. Yamamoto, T. Nakamura, T. Inatomi and J. Bush, N. Engl. J. Med., 2018, 378, 995–1003 CrossRef CAS.
- M. Rizwan, G. S. Peh, H.-P. Ang, N. C. Lwin, K. Adnan, J. S. Mehta, W. S. Tan and E. K. Yim, Biomaterials, 2017, 120, 139–154 CrossRef CAS.
- R. M. Gouveia, E. González-Andrades, J. C. Cardona, C. González-Gallardo, A. M. Ionescu, I. Garzon, M. Alaminos, M. González-Andrades and C. J. Connon, Biomaterials, 2017, 121, 205–219 CrossRef CAS.
- T. Bayyoud, S. Thaler, J. Hofmann, C. Maurus, M. S. Spitzer, K.-U. Bartz-Schmidt, P. Szurman and E. Yoeruek, Curr. Eye Res., 2012, 37, 179–186 CrossRef CAS.
- N. Koizumi, Y. Sakamoto, N. Okumura, H. Tsuchiya, R. Torii, L. J. Cooper, Y. Ban, H. Tanioka and S. Kinoshita, Cornea, 2008, 27, S48–S55 CrossRef.
- E. Yoeruek, T. Bayyoud, C. Maurus, J. Hofmann, M. S. Spitzer, K. U. Bartz-Schmidt and P. Szurman, Acta Ophthalmol., 2012, 90, e125–e131 CrossRef.
- J. Yoshida, A. Oshikata-Miyazaki, S. Yokoo, S. Yamagami, T. Takezawa and S. Amano, Invest. Ophthalmol. Visual Sci., 2014, 55, 4975–4981 CrossRef.
- X. Gao, W. Liu, B. Han, X. Wei and C. Yang, J. Mater. Sci.: Mater. Med., 2008, 19, 3611–3619 CrossRef CAS.
- B. Ozcelik, K. D. Brown, A. Blencowe, K. Ladewig, G. W. Stevens, J. P. Y. Scheerlinck, K. Abberton, M. Daniell and G. G. Qiao, Adv. Healthcare Mater., 2014, 3, 1496–1507 CrossRef CAS.
- F. Arnalich-Montiel, A. Moratilla, S. Fuentes-Julián, V. Aparicio, M. Cadenas Martin, G. Peh, J. S. Mehta, K. Adnan, L. Porrua and A. Pérez-Sarriegui, PLoS One, 2019, 14, e0225480 CrossRef CAS.
- F. N. Syed-Picard, Y. Du, K. L. Lathrop, M. M. Mann, M. L. Funderburgh and J. L. Funderburgh, Stem Cells Transl. Med., 2015, 4, 276–285 CrossRef CAS.
- J. Oliva, F. Bardag-Gorce and Y. Niihara, Int. J. Mol. Sci., 2020, 21, 411 CrossRef.
- K. R. Keivyon and S. C. Tseng, Ophthalmology, 1989, 96, 709–723 CrossRef.
- J. Chen and S. Tseng, Invest. Ophthalmol. Visual Sci., 1990, 31, 1301–1314 CAS.
- E. M. Espana, M. Grueterich, A. C. Romano, A. Touhami and S. C. Tseng, Ophthalmology, 2002, 109, 2004–2010 CrossRef.
- S. M. Daya, Curr. Opin. Ophthalmol., 2017, 28, 370–376 CrossRef.
- W. Armitage, A. Tullo and D. Larkin, Br. J. Ophthalmol., 2006, 90, 1222–1223 CrossRef CAS.
- J. T. Theng and D. T. Tan, Ophthalmic Surg., Lasers Imaging Retina, 1997, 28, 765–768 CAS.
- R. J.-F. Tsai, L.-M. Li and J.-K. Chen, N. Engl. J. Med., 2000, 343, 86–93 CrossRef CAS.
- R. J.-F. Tsai and S. C. Tseng, Cornea, 1994, 13, 389–400 CrossRef CAS.
- R. Langer, Tissue Eng. Sci., 1993, 260, 920–926 CAS.
- Y.-H. K. Yang, Regener. Ther., 2018, 9, 120–122 CrossRef.
- A. Stolzing, E. Jones, D. Mcgonagle and A. Scutt, Mech. Ageing Dev., 2008, 129, 163–173 CrossRef CAS.
- S. Zhou, J. S. Greenberger, M. W. Epperly, J. P. Goff, C. Adler, M. S. LeBoff and J. Glowacki, Aging Cell, 2008, 7, 335–343 CrossRef CAS.
- R. A. Eid, F. Sawair, G. Landini and T. Saku, Age, 2012, 34, 651–658 CrossRef.
- M. S. Choudhery, M. Badowski, A. Muise, J. Pierce and D. T. Harris, J. Transl. Med., 2014, 12, 8 CrossRef.
- A. Blázquez-Prunera, J. M. Díez, R. Gajardo and S. Grancha, Stem Cell Res. Ther., 2017, 8, 1–11 CrossRef.
- C. Grezella, E. Fernandez-Rebollo, J. Franzen, M. S. V. Ferreira, F. Beier and W. Wagner, Stem Cell Res. Ther., 2018, 9, 1–6 CrossRef.
- Q. Zhang, H. Wang, Y.-J. Yang, Q.-T. Dong, T.-J. Wang, H.-Y. Qian, N. Li, X.-M. Wang and C. Jin, Int. J. Cardiol., 2014, 176, 670–679 CrossRef.
- S. X. Deng, V. Borderie, C. C. Chan, R. Dana, F. C. Figueiredo, J. A. Gomes, G. Pellegrini, S. Shimmura and F. E. Kruse, Cornea, 2019, 38, 364 CrossRef.
- J. Shimazaki, S. Shimmura, H. Fujishima and K. Tsubota, Ophthalmology, 2000, 107, 1518–1523 CrossRef CAS.
- D. Hos, D. R. Saban, F. Bock, B. Regenfuss, J. Onderka, S. Masli and C. Cursiefen, Arch. Ophthalmol., 2011, 129, 445–452 CrossRef CAS.
- T. Nakamura, T. Inatomi, C. Sotozono, T. Amemiya, N. Kanamura and S. Kinoshita, Br. J. Ophthalmol., 2004, 88, 1280–1284 CrossRef CAS.
- Bovine Derived Materials Used in Vaccine Manufacturing Questions and Answers, https://www.fda.gov/vaccines-blood-biologics/questions-about-vaccines/bovine-derived-materials-used-vaccine-manufacturing-questions-and-answers, 2018.
- M. Hirayama, Y. Satake, K. Higa, T. Yamaguchi and J. Shimazaki, Invest. Ophthalmol. Visual Sci., 2012, 53, 1602–1609 CrossRef.
- S. Kolli, S. Ahmad, H. S. Mudhar, A. Meeny, M. Lako and F. C. Figueiredo, Stem Cells, 2014, 32, 2135–2146 CrossRef CAS.
- C. Priya, P. Arpitha, S. Vaishali, N. Prajna, K. Usha, K. Sheetal and V. Muthukkaruppan, Eye, 2011, 25, 1641–1649 CrossRef CAS.
- Y. Satake, K. Higa, K. Tsubota and J. Shimazaki, Ophthalmology, 2011, 118, 1524–1530 CrossRef.
- L. P. Ang, T. Nakamura, T. Inatomi, C. Sotozono, N. Koizumi, N. Yokoi and S. Kinoshita, Arch. Ophthalmol., 2006, 124, 1543–1551 CrossRef.
- T. Inatomi, T. Nakamura, N. Koizumi, C. Sotozono, N. Yokoi and S. Kinoshita, Am. J. Ophthalmol., 2006, 141, 267–275 CrossRef.
- C. Sotozono, T. Inatomi, T. Nakamura, N. Koizumi, N. Yokoi, M. Ueta, K. Matsuyama, H. Kaneda, M. Fukushima and S. Kinoshita, Acta Ophthalmol., 2014, 92, e447–e453 Search PubMed.
- C. Sotozono, T. Inatomi, T. Nakamura, N. Koizumi, N. Yokoi, M. Ueta, K. Matsuyama, K. Miyakoda, H. Kaneda and M. Fukushima, Ophthalmology, 2013, 120, 193–200 CrossRef.
- D. W. Jayme and S. R. Smith, Cytotechnology, 2000, 33, 27–36 CrossRef CAS.
- A. Stein, BioTechniques, 2007, 43, 228–229 CrossRef.
- S. Canonico, Acta Bio-Med. Ateneo Parmense, 2003, 74, 21–25 Search PubMed.
- N. Di Girolamo, J. Chui, D. Wakefield and M. T. Coroneo, Br. J. Ophthalmol., 2007, 91, 459–464 CrossRef.
- K. Nishida, M. Yamato, Y. Hayashida, K. Watanabe, K. Yamamoto, E. Adachi, S. Nagai, A. Kikuchi, N. Maeda and H. Watanabe, N. Engl. J. Med., 2004, 351, 1187–1196 CrossRef CAS.
- Y. H. Kim, D. H. Kim, E. J. Shin, H. J. Lee, W. R. Wee, S. Jeon and M. K. Kim, PLoS One, 2016, 11, e0147548 CrossRef.
- Y. J. Kim, H. J. Lee, J. S. Ryu, Y. H. Kim, S. Jeon, J. Y. Oh, H. K. Choung, S. I. Khwarg, W. R. Wee and M. K. Kim, Cornea, 2018, 37, 76–83 CrossRef.
- I. Shukla, Indian J. Ophthalmol., 1962, 10, 55 CAS.
- N. Koizumi, T. Inatomi, A. J. Quantock, N. J. Fullwood, A. Dota and S. Kinoshita, Cornea, 2000, 19, 65–71 CrossRef CAS.
- N. Koizumi, N. J. Fullwood, G. Bairaktaris, T. Inatomi, S. Kinoshita and A. J. Quantock, Invest. Ophthalmol. Visual Sci., 2000, 41, 2506–2513 CAS.
- S. C. Tseng, P. Prabhasawat, K. Barton, T. Gray and D. Meller, Arch. Ophthalmol., 1998, 116, 431–441 CrossRef CAS.
- M. Avila, M. España, C. Moreno and C. Peña, Cornea, 2001, 20, 414–420 CrossRef CAS.
- P. Prabhasawat, P. Ekpo, M. Uiprasertkul, S. Chotikavanich, N. Tesavibul, K. Pornpanich and P. Luemsamran, Cell Tissue Banking, 2016, 17, 491–503 CrossRef CAS.
- A. Baradaran-Rafii, S. Delfazayebaher, N. Aghdami, E. Taghiabadi, S. Bamdad and D. Roshandel, Ocul. Surf., 2017, 15, 789–794 CrossRef.
- Y. Satake, M. Dogru, G.-Y. Yamane, S. Kinoshita, K. Tsubota and J. Shimazaki, Arch. Ophthalmol., 2008, 126, 23–28 CrossRef.
- K. Takeda, T. Nakamura, T. Inatomi, C. Sotozono, A. Watanabe and S. Kinoshita, Am. J. Ophthalmol., 2011, 152, 195–201 CrossRef.
- M. Heskins and J. E. Guillet, J. Macromol. Sci., Chem., 1968, 2, 1441–1455 CrossRef CAS.
- K. Ohashi, T. Yokoyama, M. Yamato, H. Kuge, H. Kanehiro, M. Tsutsumi, T. Amanuma, H. Iwata, J. Yang and T. Okano, Nat. Med., 2007, 13, 880–885 CrossRef CAS.
- T. Shimizu, M. Yamato, T. Akutsu, T. Shibata, Y. Isoi, A. Kikuchi, M. Umezu and T. Okano, J. Biomed. Mater. Res., 2002, 60, 110–117 CrossRef CAS.
- M. Yamato, M. Okuhara, F. Karikusa, A. Kikuchi, Y. Sakurai and T. Okano, J. Biomed. Mater. Res., 1999, 44, 44–52 CrossRef CAS.
- P. Singh, C. Carraher and J. E. Schwarzbauer, Annu. Rev. Cell Dev. Biol., 2010, 26, 397–419 CrossRef CAS.
Footnote |
† Equal contribution. |
|
This journal is © The Royal Society of Chemistry 2020 |