DOI:
10.1039/D0BM01154A
(Minireview)
Biomater. Sci., 2020,
8, 5846-5858
Biomaterial-assisted photoimmunotherapy for cancer
Received
13th July 2020
, Accepted 6th September 2020
First published on 5th October 2020
Abstract
With the development of phototherapy, which is a type of light-induced cancer treatment, various biomaterials have been well designed as photoabsorbing/sensitizing agents or effective carriers to enhance the therapeutic efficacy and evade the side effects of phototherapy. In recent years, the immunological responses induced by phototherapy have been widely explored, which are mainly triggered by the tumor associated antigens (TAAs) released from the dying cancer cells after phototherapy, together with the secretion of damage associated molecular patterns (DAMPs) and various pro-inflammatory cytokines/factors. To amplify these immunological responses induced by phototherapy, various adjuvant nano/micromaterials are introduced to boost the immune system to recognize and kill cancer cells. Moreover, such immune responses are further demonstrated to work in synergy with other immunotherapies such as immune checkpoint blockade (ICB), chimeric antigen receptor (CAR)-T cell and cytokine therapy, achieving significantly increased immune response rates and successful therapeutic outcomes. Here, this minireview will focus on the recent progress in engineering biomaterials for enhanced photoimmunotherapy and discuss the challenges, opportunities and future prospects in this field.
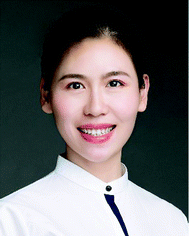 Qian Chen | Dr Qian Chen received her BS degree and PhD degree in 2013 and 2017, respectively, both from Soochow University. She then worked at the University of North Carolina at Chapel Hill as a postdoctoral scholar from 2017 to 2018. After that, she worked at the University of California, Los Angeles (UCLA) as a post-doc from 2018 to 2019. In 2019, Dr Chen joined the Institute of Functional Nano & Soft Materials (FUNSOM) at Soochow University as a principal investigator. Dr Chen's research focuses on leveraging biomaterials and biomedical engineering strategies for applications in multimodal biomedical imaging, cancer immunotherapy and tissue engineering. She has published over 60 peer-reviewed papers in leading journals including Nat. Nanotechnol., Nat. Commun., Proc. Natl. Acad. Sci. U. S. A., Adv. Mater., etc. |
1. Introduction
Phototherapy, a class of non-invasive therapeutic techniques, usually utilizes phototherapeutic agents to selectively kill cancer cells under appropriate light irradiation. Generally, it falls into two categories, namely, photothermal therapy (PTT) and photodynamic therapy (PDT).1 PTT usually employs light-absorbing agents, such as gold nanostructures with various morphologies, carbon nanomaterials, transition metal oxides and sulfides, as well as various other inorganic and organic nanoparticles with strong absorbance in the near infrared (NIR) region, effectively converting the photoenergy into heat to kill cancer cells.2–4 In comparison with PTT, PDT is a more mature strategy of phototherapy which has been used in the clinic.5 During PDT, photosensitizers could generate singlet oxygen (1O2) (a kind of reactive oxygen species (ROS)) to destroy cancer cells under suitable light irradiation.6 Various photosensitizers including Photofrin®, Chlorin e6 (Ce6), and methylene blue have been widely used in both fundamental research and the clinic.7–9 However, most of these photosensitizers exhibit poor therapeutic results and serious phototoxicity due to their limited tumor homing ability.10 Various micro- or nanoparticles including micelles, liposomes, proteins, dendrimers, and so on have been extensively used to deliver photosensitizers to improve the efficacy of PDT as well as reduce their phototoxicity.11–14
In addition, to directly destroy tumors with high selectivity and low systemic toxicity, phototherapy is able to trigger strong immunological responses.15 It has been demonstrated that dying tumor cells after phototherapy are able to release tumor-associated antigens (TAAs) and self-antigens, which could be captured by antigen-presenting cells (APCs) particularly dendritic cells (DCs) and then presented to adaptive immune cells.16 Meanwhile, various immunogenic factors, such as the secretion of damage associated molecular patterns (DAMPs) including heat-shock proteins (HSPs), high-mobility group box 1 (HMGB1), and adenosine triphosphate (ATP), the exposure of calreticulin (CRT) on the surface of cancer cells, and the release of pro-inflammatory cytokines/factors (TNF-α, IL-1β, IL-6, IL-8 and IL-10), have been identified during the dying of cancer cells after phototherapy.17–19 However, the immune responses induced by phototherapy itself are usually limited in killing cancer cells. Various adjuvants including cytosine guanine (CpG) oligodeoxynucleotides, lipopolysaccharides (LPS), monophosphoryl lipid A (MPLA), imiquimod (R837), resiquimod (R848) and polyinosinic
:
polycytidylic acid (poly I
:
C) are introduced to amplify the immune responses induced by phototherapy.20–22 As demonstrated by our group, the TAAs released after phototherapy, working together with the adjuvant, could act as “tumor vaccines” to stimulate the immune system.23 Furthermore, such immune responses induced by phototherapy are able to work in synergy with other immunotherapies including immune checkpoint blockade (ICB), chimeric antigen receptor (CAR)-T cell and cytokine therapy, achieving significantly increased immune response rates and successful therapeutic outcomes.24–26
For photoimmunotherapy, multifunctional biomaterials not only could act as phototherapeutic agents or carriers to promote the efficacy of phototherapy itself, but also can deliver immunostimulatory cargoes such as adjuvants to amplify the anti-tumor immune responses induced by phototherapy.27 More importantly, the engineered biomaterials also have the ability to deliver and release various immunotherapeutic agents, including antibodies, cytokines and even immune cells, remodeling the microenvironment where immune cells meet cancer cells, antigens, inhibitors or stimulatory signals, and other immune cells.28 In this review article, we will first discuss the design and development of biomaterials that are used to maximize the efficiency of phototherapy and then provide an overview of the immune responses induced by phototherapy together with combining phototherapy with other immunotherapeutic strategies to achieve synergistic therapeutic efficacy (Fig. 1). Finally, future perspectives and clinical challenges in this field of biomaterial-assisted photoimmunotherapy are also discussed.
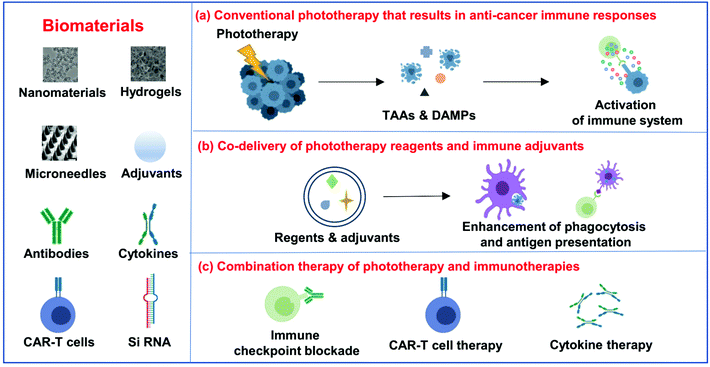 |
| Fig. 1 Scheme of biomaterial-assisted photoimmunotherapy for cancer. In this review article, the following three points are mainly discussed: (a) conventional phototherapy that results in anti-cancer immune responses; (b) co-delivery of phototherapy reagents and immune adjuvants; and (c) combination therapy of phototherapy and immunotherapies. | |
2. Biomaterial-assisted phototherapy
Phototherapy, including PTT and PDT, is a type of light-triggering local treatment, exhibiting many unique advantages including improved selectivity and controllability, as well as low systemic toxicity.29 PTT depends on light absorbing agents to effectively convert the energy of light into heat, increasing the local temperature of tumors to kill cancer cells.30 On the other hand, PDT relies on the photosensitizers, which are able to transfer the energy of light to the surrounding oxygen molecules, generating cytotoxic 1O2 to damage cancer cells.31 Recently, various biomaterials have been investigated to enhance the therapeutic efficacy of the two types of light-triggered therapies.
To realize safe and effective PTT, ideal photothermal agents should exhibit strong absorbance in the NIR region and convert the absorbed optical energy into heat effectively. In the past few years, various biomaterials with high NIR absorbance have been investigated as PTT agents. Commonly exploited photothermal agents are inorganic nanoparticles including different noble metal nanostructures (nanoparticles, nanorods, nanoshells, nanocages, nanostars, nanoprisms, nanocomposites and alloys), transition metal sulfides and oxides, carbon nanomaterials (carbon nanotubes and graphene) and so on.4,32,33 After appropriate surface coating, these inorganic photothermal agents exhibit high efficiency in ablating tumors in animal experiments. However, the non-biodegradable ability of most inorganic PTT agents limited their future clinical application owing to the concerns about their long-term toxicity.34 In addition to inorganic photothermal agents, some organic conjugated polymers such as polypyrrole and polyaniline also have been explored as efficient PTT agents.35,36 Despite the satisfactory therapeutic results achieved by these organic conjugated polymers, their biodegradation behaviors still remain unclear. Recently, various biomaterials, including liposomes, micelles, porphysomes and protein, encapsulating NIR dyes (e.g. indocyanine green (ICG)), have been investigated as safe and effective PTT agents.37–40 Thanks to the biodegradability and biocompatibility of the components in these biomaterials, they may have less safety concerns and encounter less challenges for clinical translation in the future.
PDT, another type of light-triggering therapeutic strategy, relies on three important components: photosensitizers, light and oxygen (O2).41 During PDT, photosensitizers are able to transfer the absorbed optical energy to the surrounding O2 to generate cytotoxic 1O2 to kill tumor cells. Various photosensitizers including Ce6, Photofrin®, methylene blue and 5-aminolaevulinic have been used in both fundamental research and the clinic.7–9 However, most of these photosensitizers do not show ideal therapeutic results owing to their limited tumor accumulation ability. In the past several decades, various biomaterials, such as micelles, liposomes, proteins, polymer microcapsules, dendrimers and cerasomes, have been extensively used to deliver photosensitizers to improve the therapeutic efficacy of PDT and reduce their phototoxicity.11–14 Furthermore, some inorganic nanomaterials with optical properties, such as metallic nanoparticles, carbon nanomaterials and quantum dots, also have been explored to deliver photosensitizers, or even used as PDT agents themselves.42 Considering that most of these photosensitizers are excited by visible light, which usually shows limited tissue penetration depth, upconversion nanoparticles (UCNPs), a kind of unique optical nanomaterial, which could emit visible light under NIR light irradiation, have been developed as a novel kind of carrier for PDT with significantly improved tissue penetration depth.43,44 On the other hand, considering the oxygen-dependent nature of PDT, the hypoxic tumor microenvironment (TME) usually results in ineffective therapeutic efficacy. To overcome this limitation, various biomaterials have been developed to replenish O2 and/or diminish the dependence on O2 during PDT.45 Some biomaterials with excellent catalytic performance, such as catalases, manganese dioxide nanoparticles, and Pt nanozymes, have been explored to generate O2 by decomposing hydrogen peroxide (H2O2) in the TME.46–48 More directly, some unique biomaterials with O2 transport capacity, including perfluorocarbons, hemoglobin and artificial red cells, can be engineered as effective O2 carriers to transport O2 into the tumor.49–51 Moreover, some unique photosensitizers based on boron dipyrromethene and ruthenium complexes have been developed to exhibit high ROS production ability even in the hypoxic TME, achieving excellent therapeutic efficacy of PDT.52,53
3. Biomaterial-assisted photothermal immunotherapy
(a) Conventional phototherapy that results in anticancer immune responses
In the past few decades, a large number of biomaterials with high NIR absorbance have been explored for PTT, which is effective for local tumor treatment owing to the sensitivity of cancer cells to high temperatures. However, the recurrence and metastasis of tumors is the biggest challenge for PTT.54 The ideal PTT should not only eliminate the primary treated tumors but also should be able to control tumor metastasis and recurrence. The principle of in situ photoimmunotherapy (ISPI), which combines PTT and immunological stimulation with an immune adjuvant, was first proposed in 1997.55 It was found that dying cancer cells after PTT could release TAAs, thermally induced HSPs, together with self-antigens. APCs, particularly DCs, would capture these antigens and present them to T cells, to activate the adaptive immune system.
Although PTT itself could activate the immune system and recruit different immune cells, it might be insufficient to trigger effective anti-tumor immune responses. Therefore, a strategy that combines immune adjuvants and PTT-induced immunological responses has been widely investigated.28,56 As a successful example in a preliminary clinical study, R837, a Food and Drug Administration (FDA) approved toll-like receptor 7 (TLR7) agonist, was introduced to further stimulate the immune responses induced by PTT on patients with late-age melanoma.57 Excitingly, eleven patients with multiple cutaneous metastases received ISPI in one or multiple 6-week treatment cycles by a local injection of ICG and R837 and local irradiation with NIR laser, and exhibited strong anti-tumor immune responses to inhibit tumor growth and metastasis. Five patients after ISPI treatment were still alive at the time of their last follow-up, indicating the unique advantages of ISPI with imiquimod for patients with advanced and late-stage melanoma.
(b) Co-delivery of phototherapy reagents and immune adjuvants
With the development of biotechnology, emerging interest is focused on leveraging biomaterials, which not only could act as effective PTT agents, but also are able to deliver immunostimulatory cargoes to promote the anti-tumor immune responses induced by PTT.27 In 2014, Lu and co-workers developed chitosan-coated hollow CuS nanoparticles (HCuSNPs-CpG) encapsulating CpG oligodeoxynucleotides as PTT agents and immune adjuvants (Fig. 2). Under NIR laser irradiation, the temperature of tumors could obviously increase to “burn” cancer cells and release the TAAs into the surrounding milieu. Meanwhile, as the temperature increased, the structure of CuS could break down, reassemble, and transform into polymer complexes to release CpG and promote the uptake of antigens and CpG by plasmacytoid DCs within the tumor. Their results indicated that such combined photothermal and immunotherapy was effective to activate the immune system and successfully inhibit the growth of both primary and distant tumors (Fig. 2b and c). Iron oxide nanoparticles, a clinically approved transition metal oxide, were developed as a kind of magnetic-targeting immunostimulatory nanoagent to load with CpG for photothermally triggered immunotherapy by Ran and co-workers. Under external magnetic fields, these nanoparticles could accumulate in the tumor region more effectively and therefore stir a more powerful photoimmune response.59 Zhou et al. synthesized bovine serum albumin-bioinspired gold nanorods (GNRs) loaded with R837 for the combined PTT-induced immunotherapy for the treatment of melanoma.60 Moreover, considering the excellent photothermal properties of graphene, Qu and co-workers used graphene oxide as the carrier for the controllable delivery of CpG and PTT.61 Under NIR light irradiation, locally generated heating by graphene could accelerate the intracellular trafficking of nanoparticles, significantly improving the immunostimulation responses and production of proinflammatory cytokines induced by CpG. In animal experiments, excellent photothermal and immunological therapeutic efficacy was achieved. In another work, Liu and co-workers developed a type of versatile nanoparticle (PC@GCpD(Gd)) based on polydopamine stabilized graphene quantum dots (GQD), CpG oligodeoxynucleotides and gadolinium ion (Gd3+) for fluorescence/magnetic resonance imaging-guided photoimmunotherapy (Fig. 2d).62 After intravenous injection, these versatile nanoparticles could effectively accumulate into the tumor to kill cancer cells by increasing the local temperature under laser irradiation. More importantly, the contemporaneously delivered CpG was able to further stimulate the secretion of pro-inflammatory cytokines and promote the maturation of DCs, effectively activating tumor specific T lymphocytes (Fig. 2e). As shown in the therapeutic results, such photothermal immunotherapy induced strong anti-tumor immune responses in inhibiting the metastasis of EMT6 murine mammary tumors (Fig. 2f). In addition to inorganic photothermal agents, in 2018, Gong and co-workers encapsulated small molecule dyes with high NIR absorbance, IR-7, and the immunoadjuvant hyaluronic acid linked CpG (HA-CpG) into liposomes for effective photothermal and immunotherapy.63 It was found that the photothermal effect induced by IR-7 could induce the necrosis of cancer cells and promote the release of TAAs. Interestingly, the presence of the immunoadjuvant CpG was able to promote the maturation of DCs, realizing effective antigen presentation and activation of T cells to recognize and kill cancer cells. In addition to nanomaterials, some unique biomaterials also have been investigated to co-encapsulate NIR absorbing agents and immunostimulators for enhanced photoimmunotherapy. In a recent work, Gu and co-workers developed a melanin-mediated photoimmunotherapy based on the transdermal microneedle (MN) patch delivery system (Fig. 3a).64 In this work, B16F10 tumor lysate containing melanin was loaded into the MN, which allowed sustained release of the tumor-associated lysate after insertion into the skin. Meanwhile, with NIR light irradiation, the photothermal effect of melanin in the patch caused the local acute inflammatory and released some signal molecules to activate the immune system, leading to enhanced antitumor vaccination. It was found that photothermal immunotherapy induced by the polymeric MN loaded B16F10 tumor lysate could increase the infiltration of T cells and the secretion of cytokines, obviously prolonging the survival of mice after tumor challenge and eliciting established primary and metastatic tumors. Hydrogels, a kind of commonly used biomaterial in the clinic, are also developed as the platform to enhance photoimmunotherapy.15 In one of their works, Wei's group encapsulated the self-assembled nanoparticles (composed of R848, ICG and CpG) into the thermosensitive PDLLA–PEG–PDLLA (PLEL) hydrogel, achieving NIR-stimulated release of immune components (Fig. 3b).65 Working together with the TAAs released after PTT, it could effectively activate the immune system to prevent cancer metastasis and recurrence (Fig. 3c and d). In 2017, Nishikawa and co-workers synthesized an immunostimulatory DNA hydrogel by assembling designed hexapod–DNA containing CpG sequences with gold nanoparticles.66 As expected, with NIR laser irradiation, the hydrogel could disassemble and release the hexapod–DNA containing CpG sequences, achieving excellent anti-tumor efficacy.
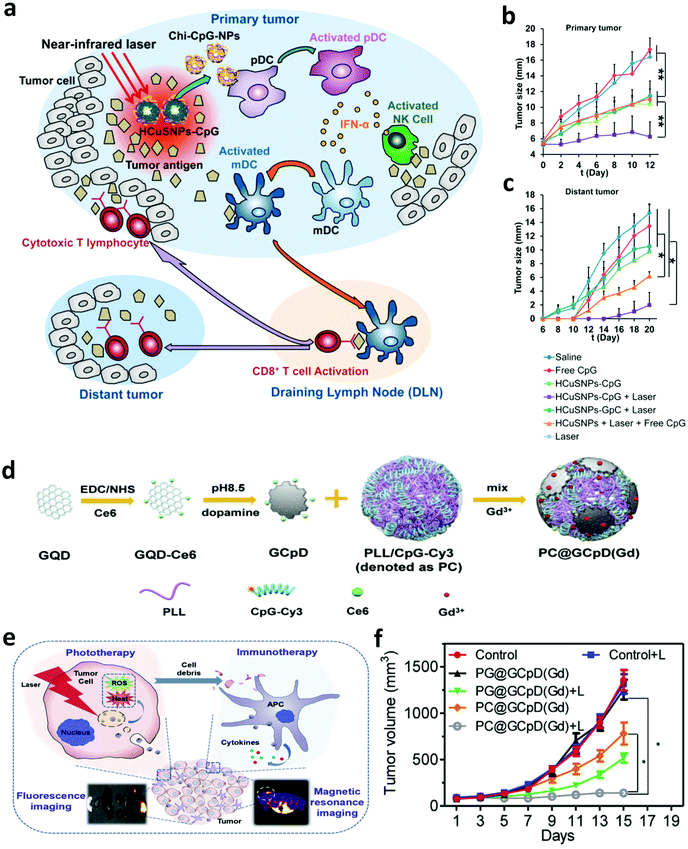 |
| Fig. 2 (a) Mechanisms of the anti-tumor immune responses induced by the chitosan-coated hollow CuS nanoparticles containing CpG oligodeoxynucleotides for PTT. (b and c) Tumor-growth curves of primary and distant tumors in mice after different treatments. (d) Synthesis of versatile nanoparticles based on polydopamine stabilized graphene quantum dots, CpG oligodeoxynucleotides and gadolinium ion. (e) Mechanisms of the anti-tumor immune responses induced by PC@GCpD(Gd) for PTT. (f) Tumor-growth curves of EMT6 murine mammary tumors in mice after different treatments. Reproduced from ref. 58 with permission from the [ACS], copyright [2014], ref. 62 with permission from [Elsevier], copyright [2019]. | |
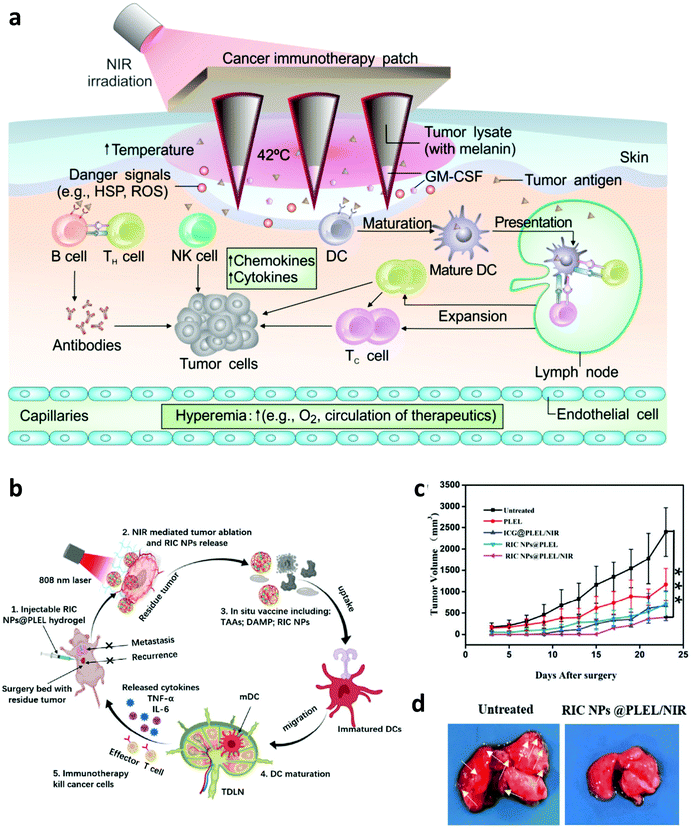 |
| Fig. 3 (a) Mechanisms of anti-tumor immune responses induced by melanin-mediated photoimmunotherapy based on the transdermal microneedle patch. (b) Mechanisms of anti-tumor immune responses induced by the thermosensitive NPs@PLEL hydrogel for PTT. (c) Tumor-growth curves of mice showed inhibition to cancer recurrence. (d) Photograph and tissue slices of the lung in mice showed inhibition to cancer metastasis. Reproduced from ref. 64 with permission from the [American Association for the Advancement of Science], copyright [2017], ref. 65 with permission from [Wiley], copyright [2020]. | |
(c) Combination therapy of phototherapy and immunotherapy
Although combing PTT with immune adjuvants could activate the immune system, it might not be enough to induce efficient anti-tumor immune responses due to the presence of immunosuppressive cells or factors.67 For example, the existence of immune checkpoints, such as cytotoxic T-lymphocyte-associated antigen 4 (CTLA-4) and programmed cell death protein 1 and its ligand (PD-1/PD-L1), could negatively regulate the tumor specific T cells and lead to the failure of immunotherapy.68 Thus, many efforts have been devoted to investigate the synergistic anti-tumor effects induced by PTT and ICB. In 2014, our group investigated the immune responses induced by single-walled carbon nanotube (SWNT) based PTT and CTLA-4 blockade.69 Interestingly, we found that in combination with anti-CTLA-4 treatment, more CD8+ T cells were activated and the regulatory T cells (Tregs) were inhibited after SWNT-based PTT of the primary tumor, showing greatly enhanced therapeutic efficacy on both subcutaneous tumor and lung metastatic tumor models (Fig. 4a). In a later study, our group further investigated the immune responses induced by combining photothermal ablation of the primary tumor with adjuvant nanoparticles and ICB. In this work, the photothermal and immune adjuvant nanoparticles were synthesized using three FDA-approved agents, including poly(lactic-co-glycolic) acid (PLGA), ICG and R837 (Fig. 4b).23 It was found that photothermal ablation of the primary tumor could release TAAs, which working together with immune adjuvant nanoparticles containing R837 exhibit vaccine-like functions. Excitingly, in combination with CTLA-4 blockade, the generated anti-tumor immune responses are enhanced to attack the remaining cancer cells, which are useful in inhibiting cancer metastasis and recurrence (Fig. 4c–e). In 2019, Sun's group presented a combined all-in-one and all-in-control strategy based on thermally reversible lipid gels that were loaded with the photothermal agent IR820 and anti-PD-L1 antibody, which could sensitize the tumor to ICB for synergistic therapy.70 Both 4T1 carcinoma and B16F10 melanoma tumor models showed amplified ICB immune therapy, significantly inhibiting cancer metastasis.
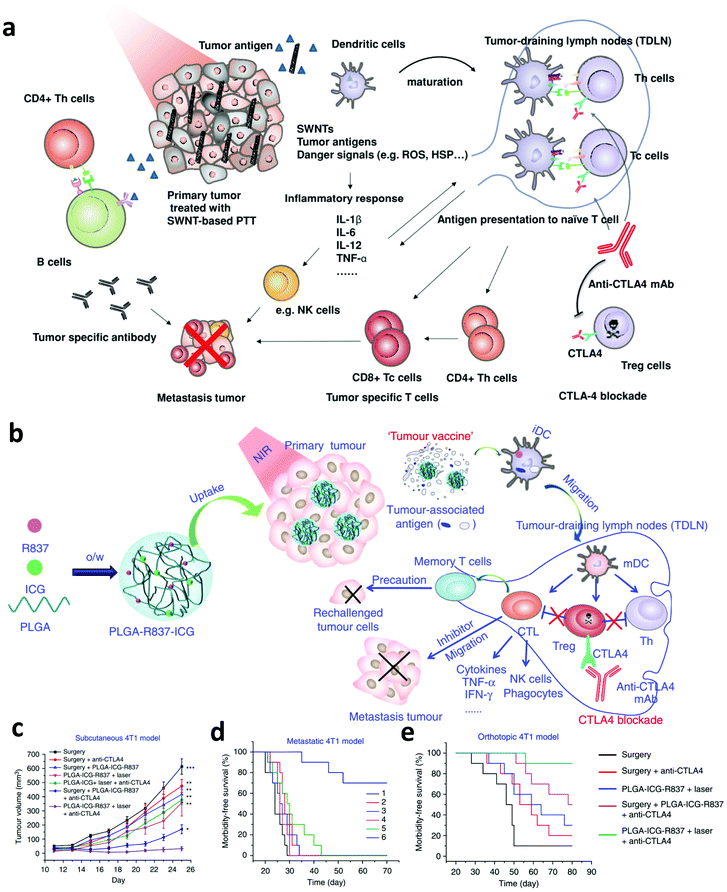 |
| Fig. 4 (a) Mechanisms of synergistic anti-tumor effects induced by PTT based on SWNTS and ICB. (b) Mechanisms of the “tumor vaccine” based on PLGA-R837-ICG nanoparticles for PTT. (c, d and e) Tumor-growth curves of mice bearing subcutaneous 4T1 tumors and survival curves of mice bearing the metastatic and orthotopic 4T1 tumor model. Reproduced from ref. 69 with permission from [Wiley], copyright [2014], ref. 23 with permission from [Springer Nature], copyright [2017]. | |
In addition to in combination with ICB, photothermal ablation of solid tumors has also been investigated to enhance the efficacy of other immunotherapies such as CAR-T cell therapy, which exhibits modest therapeutic efficiency in treating solid tumors due to the inefficient infiltration of CAR-T cells into the tumor and the presence of immunosuppressive TME.71 In a recent work, Chen et al. explored the synergistic therapeutic effect induced by PTT and CAR-T cell therapy.72 It was found that mild hyperthermia of the solid tumor could reduce the compact structure and interstitial fluid pressure, increase the blood perfusion, and recruit different endogenous immune cells in the solid tumor, which working together could obviously promote the accumulation and activation of CAR-T cells into the solid tumor (Fig. 5a and b). Thus, photothermal ablation of the tumor may be a promising strategy to increase the therapeutic index of CAR-T cells against solid tumors (Fig. 5c). In addition to CAR-T cell therapy, combining PTT with cytokine therapy also attracted wide attention. In 2018, Zhang and co-workers designed a thermally sensitive programmable bacteria (TBP), E. coli MG1655, that transformed with plasmids expressing cytotoxic tumor necrosis factor α (TNF-α) and decorated with gold nanoparticles (Fig. 5d and e).73 After the oral administration of TBP, these bacteria could be transported to internal microcirculation and accumulated into the tumor region due to their anaerobic feature. The generation of heat in the tumor under NIR laser irradiation could control the expression of TNF-α to induce apoptotic cancer cell death, exhibiting excellent antitumor efficacy (Fig. 5f).
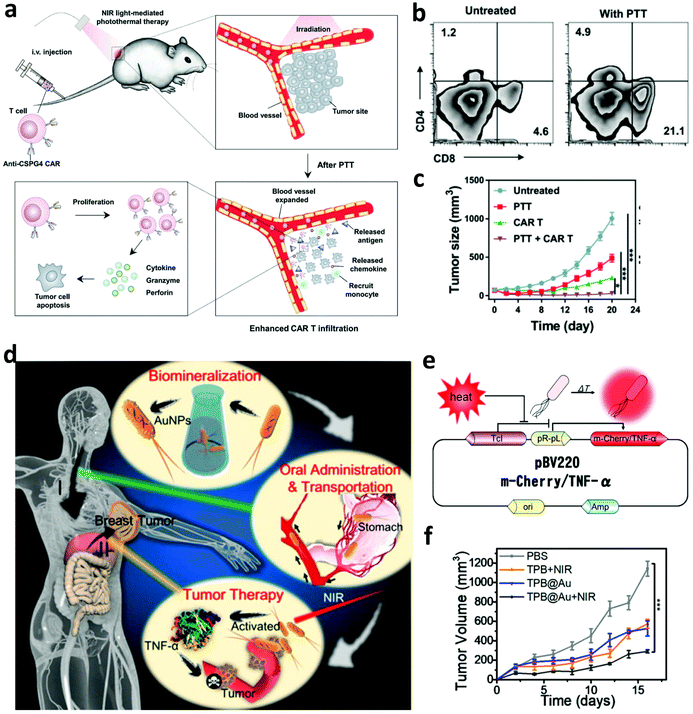 |
| Fig. 5 (a) Mechanisms of synergistic therapeutic effects induced by PTT and CAR-T cell therapy. (b) Infiltration of CAR-T cells into the solid tumor after mild PTT. (c) Tumor-growth curves of solid tumors after different treatments. (d) Mechanisms of anti-tumor immune responses induced by the thermally sensitive programmable bacteria. (e) Mechanism of m-Cherry/TNF-α expression based on plasmid pBV220. (f) Tumor-growth curves of mice after different treatments. Reproduced from ref. 72 with permission from [Wiley], copyright [2019], ref. 73 with permission from the [ACS], copyright [2018]. | |
4. Biomaterial-assisted photodynamic immunotherapy
In addition to PTT, PDT, another type of phototherapy, is able to generate a large amount of cytotoxic 1O2 to induce the necrosis and/or apoptosis of cancer cells. Interestingly, the apoptosis and necrosis of cancer cells after PDT, also called immunogenic cell death (ICD), could stimulate the immune system by inducing the exposure of CRT, increasing the expression of HSPs and releasing TAAs.74,75 Furthermore, the generated 1O2 during PDT could also induce acute local inflammation, which is able to recruit various immune cells into the tumor and secrete various pro-inflammatory cytokines including interleukin (IL)-1β, IL-6, IL-8 and TNF-α.76 These cytokines could further stimulate the innate and adaptive immune system to attack the remaining cancer cells. In one of their works, Korbelik and co-workers studied the immune responses induced by PDT of the tumor with traditional photosensitizers including Photofrin® porfimer sodium (Photofrin) or tetra(m-tetrahydroxy-phenyl)chlorin (mTHPC).77 Their results demonstrated that a large amount of neutrophils were mobilized and attracted into the destructed tumor site after PDT. Besides, research by Berns's group indicated that the cytotoxic activity of macrophages could be stimulated by generating superoxide anions during PDT.78 Another work by Evans et al. further demonstrated that the PDT-stimulated macrophages could produce TNF-α to mediate the necrosis of cancer cells.79
Although PDT-induced inflammation could stimulate the immune system, it may not be enough to trigger the effective anti-tumor immune responses.76 The dying cancer cells after PDT usually could release TAAs, which could initiate the immune system, but immunosuppressive signals also exist to suppress the anti-tumor immune responses. Thus, many efforts have been devoted to combining PDT with various immunological stimulators including adjuvants, small molecular inhibitors and checkpoint blockade antibodies to potentiate the immune responses.80 Lin and co-workers developed a novel nanoscale metal–organic framework (MOF) (TBC-Hf) based on chlorin derivatives (TBC) and loaded the small molecular indoleamine-2,3-dioxygenase (IDO) inhibitor for combined PDT and immunotherapy (Fig. 6a).81 Interestingly, more T cells, B cells and NK cells were detected in the tumor after the activation of the immune system by combing ICD induced by PDT and IDO inhibition with the small-molecule agents. Moreover, the synergistic immune responses induced by PDT and IDO inhibitors could effectively inhibit the growth of both primary and distant tumors. In a following work, Ni et al. designed a nanoscale cationic MOF based on the dinuclear WVI secondary building units and the photosensitizer 5,10,15,20-tetra(p-benzoato)porphyrin (TBP) to facilitate the antigen presentation after PDT and promote the maturation of DCs with the help of anionic CpG.82 In another work, Xu et al. synthesized multitasking UCNP nanoparticles by simultaneously loading Ce6 and R837 (UCNP-Ce6-R837) for effective PDT immunotherapy.83 Under NIR laser irradiation, UCNP-Ce6-R837 nanoparticles could enable the effective photodynamic destruction of the tumor and release a large amount of TAAs, which working together with the immune adjuvant R837 could trigger strong anti-tumor immune responses. More interestingly, such anti-tumor immune responses could be further amplified by CTLA-4 blockade to obviously inhibit tumor metastasis and recurrence. In another work, Ni et al. developed a Cu–porphyrin (TBP)-based MOF to achieve combined PDT and immunotherapy. With the degradation of the Cu-TBP MOF, on the one hand, the released Cu2+ could catalyze estradiol-dependent ROS generation, and, on the other hand, TBP effectively mediates PDT, which could activate the immune system and elicit the systemic antitumor immunity with the help of anti-PD-L1 (Fig. 6b).84 In addition to combining PDT with monoclonal antibodies for checkpoint blockade, Li and co-workers investigated PDT-mediated cancer immunotherapy in combination with PD-L1 knockdown with small interfering RNAs (siRNAs).85 Specifically, they designed an acid-responsive micelleplex by integrating photosensitizers and PD-L1 specific siRNA (Fig. 6c). Their results indicated that the combination of PDT and PD-L1 knockdown could effectively inhibit tumor growth and metastasis.
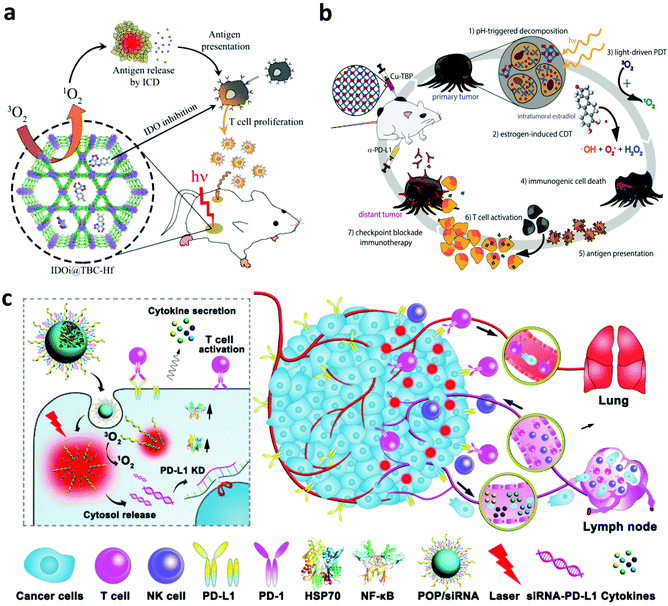 |
| Fig. 6 (a) Mechanisms of anti-tumor immune responses induced by IDOi@TBC-Hf. (b) Mechanisms of anti-tumor immune responses induced by the Cu–porphyrin-based MOF for PDT and chemodynamic therapy. (c) Mechanisms of anti-tumor immune responses induced by PDT and PD-L1 specific siRNA. Reproduced from ref. 81 with permission from the [ACS], copyright [2016] ref. 84 with permission from [Cell Press], copyright [2019], ref. 85 with permission from the [ACS], copyright [2016]. | |
Hypoxia in the TME not only limits the therapeutic efficacy of PDT, but also affects the recruitment, functions, and proliferation of various immune cells within tumors, leading to the failure of immunotherapy.86 To overcome this barrier, our group designed biodegradable hollow manganese dioxide (H-MnO2) nanoparticles loaded with Ce6 and the chemotherapeutic drug doxorubicin (DOX) (Fig. 7a).87 Taking advantage of the catalysis of H-MnO2, endogenous H2O2 was decomposed to generate O2, relieving hypoxia in the TME to further promote the cell killing efficiency of PDT. More significantly, the relieved hypoxic TME could effectively re-build the immunosuppressive microenvironment to promote anti-tumor immune responses such as the polarization of macrophage to M1-phenotype. Moreover, the enhanced PDT and chemotherapy of the primary tumor was able to release TAAs, which could be engulfed by APCs to activate the immune system. Taken together, the above-mentioned effects significantly promote the therapeutic efficacy of combined PDT, chemotherapy and ICB, obviously inhibiting the growth of both primary and distant tumors (Fig. 7b and c). Peng and co-workers developed a liposome-based nanoplatform that encapsulated catalases, NIR photosensitizers and DOX for enhanced chemo-PDT by improving the level of O2 in tumors via catalyzing intratumoral H2O2. The generated 1O2 could further modulate immune cytokines to activate the immune system, enhancing tumor inhibition in vivo.88 In another work, Wang et al. utilized a biocompatible polymer, dextran (DEX), to modify hyaluronidase via the pH-responsive linkers, formulating DEX–HAase nanoparticles (Fig. 7d).89 In the acidic TME, the released hyaluronidase could break hyaluronic acid in the condensed tumor extracellular matrix (ECM) and loosen the ECM structure, resulting in the enhanced perfusion of oxygen in the tumor. Excitingly, the improved oxygen level in the tumor not only improves the therapeutic efficacy of PDT, but also reverses the immunosuppressive TME to amplify the anti-tumor immune responses after PDT, achieving significantly enhanced synergistic effects between PDT and PD-L1 checkpoint blockade (Fig. 7e and f).
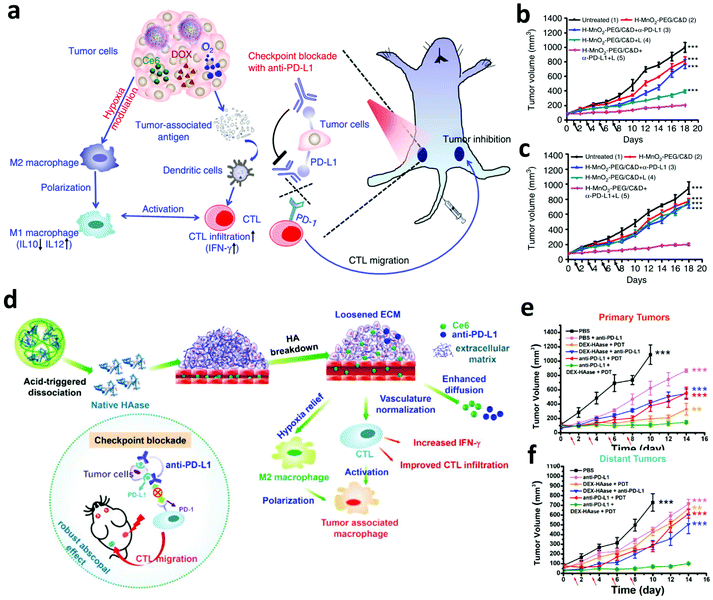 |
| Fig. 7 (a) Mechanisms of relieving hypoxic TME and synergistic therapeutic effects induced by H-MnO2 nanoparticles and anti-PD-L1. (b and c) Tumor-growth curves of primary and distant tumors in mice after different treatments. (d) Mechanisms of loosening the ECM structure and synergistic therapeutic effects induced by DEX–HAase nanoparticles and anti-PD-L1. (e and f) Tumor-growth curves of primary and distant tumors in mice after different treatments. Reproduced from ref. 87 with permission from [Springer Nature], copyright [2017], ref. 89 with permission from [Wiley], copyright [2019]. | |
5. Conclusion and future perspectives
Photoimmunotherapy has exhibited promising pre-clinical responses on different tumor models due to its unique superiorities including specific antitumor immunity and long-term immunological memory responses.90 As shown in this review, phototherapy could induce apoptotic and necrotic cancer cell death, which is different from most traditional cytotoxic agents that usually induce apoptotic tumor cell death. In the case of necrosis, also called immunogenic cell death, cytoplasmic components spill over into the extracellular space via the damaged plasma membrane and induce strong inflammatory responses, and the debris of tumor cells after phototherapy could act as tumor-associated antigens. Acute inflammation caused by phototherapy could further potentiate immune responses by attracting various immune cells and promoting the tumor-associated antigen presentation to activate the cellular immune system.
Although phototherapy is able to activate the immune system, it may not be enough to trigger effective anti-tumor immune responses due to the presence of immunosuppressive factors or cells. Various multifunctional biomaterials including nano/macroparticles, hydrogels or microneedles have been explored not only as phototherapeutic agents or carriers to promote the efficacy of phototherapy itself, but also as immune adjuvants by loading with various immunostimulants such as agonists for toll-like receptor to amplify the anti-tumor immune responses induced by phototherapy. More interestingly, such engineered biomaterials also have the ability to deliver and release various immunotherapeutic agents, including antibodies, siRNAs, cytokines and even immune cells, to remodel the immune microenvironment where immune cells meet cancer cells, antigens, inhibitory or stimulatory signals.
Despite the progress achieved, more work is still needed to investigate the dynamic immune response and understand how phototherapy impacts the specific cellular aspects of antitumor immunity, with the aim of providing basic principles or guidance for the combination of phototherapy and immunotherapy for an individual patient. Specifically, one point to be considered is to optimize phototherapy for inducing local tumor cures and producing inflammation to stimulate the immune system. Furthermore, it is also important to design suitable biomaterial-mediated phototherapy that is suitable to in combination with immunotherapeutics such as immune adjuvants or ICB agents. The timing and dosing frequency of immunotherapy are also very important to the therapeutic efficacy and should be explored in more detail. Besides, other therapeutic strategies, such as chemotherapy,67 have been reported that could trigger the similar immune response to that of phototherapy and show effective combinative anti-tumor immune responses when in combination with immune stimulates. Last but not least, the potential risk, overstimulation of the immune system with self-antigens after phototherapy, should be noted, which may lead to autoimmune toxicities. Thus, more attention should be paid to the balance between efficacy and safety rather than pursuing more powerful anti-tumor immune responses during the design of biomaterials for photoimmunotherapy. Meanwhile, biomaterials used here should be absolutely safe to avoid the possible side effects, and their formulation should allow large-scale production with low cost, which directly affects the potential of clinical translation.
Conflicts of interest
There are no conflicts to declare.
Acknowledgements
This article was partially supported by the National Research Programs of China (2016YFA0201200), the National Natural Science Foundation of China (91959104, 21927803, 51903182, 51525203), the Natural Science Foundation of Jiangsu Province (BK20190826), Collaborative Innovation Center of Suzhou Nano Science and Technology, and the 111 Program from the Ministry of Education of China.
References
- S. G. Bown, World J. Surg., 1983, 7, 700–709 CrossRef CAS.
- X. Huang, I. H. El-Sayed, W. Qian and M. A. El-Sayed, J. Am. Chem. Soc., 2006, 128, 2115–2120 CrossRef CAS.
- K. Yang, L. Feng, X. Shi and Z. Liu, Chem. Soc. Rev., 2013, 42, 530–547 RSC.
- M. Zhou, R. Zhang, M. Huang, W. Lu, S. Song, M. P. Melancon, M. Tian, D. Liang and C. Li, J. Am. Chem. Soc., 2010, 132, 15351–15358 CrossRef CAS.
- A. Braun, C. Dehn, F. Krause and S. Jepsen, J. Clin. Periodontol., 2008, 35, 877–884 CrossRef CAS.
- T. J. Dougherty, C. J. Gomer, B. W. Henderson, G. Jori, D. Kessel, M. Korbelik, J. Moan and Q. Peng, JNCI, J. Natl. Cancer Inst., 1998, 90, 889–905 CrossRef CAS.
- V. X. D. Yang, P. J. Muller, P. Herman and B. C. Wilson, Lasers Surg. Med., 2003, 32, 224–232 CrossRef.
- H. Kato, K. Furukawa, M. Sato, T. Okunaka, Y. Kusunoki, M. Kawahara, M. Fukuoka, T. Miyazawa, T. Yana, K. Matsui, T. Shiraishi and H. Horinouchi, Lung Cancer, 2003, 42, 103–111 CrossRef.
- J. P. Tardivo, A. Del Giglio, C. S. de Oliveira, D. S. Gabrielli, H. C. Junqueira, D. B. Tada, D. Severino, R. de Fátima Turchiello and M. S. Baptista, Photodiagn. Photodyn. Ther., 2005, 2, 175–191 CrossRef CAS.
- R. Weijer, M. Broekgaarden, M. Kos, R. van Vught, E. A. J. Rauws, E. Breukink, T. M. van Gulik, G. Storm and M. Heger, J. Photochem. Photobiol., C, 2015, 23, 103–131 CrossRef CAS.
- C. Vannostrum, Adv. Drug Delivery Rev., 2004, 56, 9–16 CrossRef CAS.
- L. Feng, D. Tao, Z. Dong, Q. Chen, Y. Chao, Z. Liu and M. Chen, Biomaterials, 2017, 127, 13–24 CrossRef CAS.
- Q. Chen, C. Wang, L. Cheng, W. He, Z. Cheng and Z. Liu, Biomaterials, 2014, 35, 2915–2923 CrossRef CAS.
- N. Nishiyama, Y. Morimoto, W.-D. Jang and K. Kataoka, Adv. Drug Delivery Rev., 2009, 61, 327–338 CrossRef CAS.
- X. Duan, C. Chan and W. Lin, Angew. Chem., Int. Ed., 2019, 58, 670–680 CrossRef CAS.
- J. Constantino, C. Gomes, A. Falcão, B. M. Neves and M. T. Cruz, Immunol. Res., 2017, 65, 798–810 CrossRef CAS.
- M. Korbelik, J. Banáth, J. Sun, D. Canals, Y. A. Hannun and D. Separovic, Int. Immunopharmacol., 2014, 20, 359–365 CrossRef CAS.
- M. Korbelik, W. Zhang and S. Merchant, Cancer Immunol. Immunother., 2011, 60, 1431–1437 CrossRef CAS.
- L. R. Sousa, B. N. Cavalcanti and M. M. Marques, Photomed. Laser Surg., 2009, 27, 37–42 CrossRef CAS.
- L. Chen, L. Zhou, C. Wang, Y. Han, Y. Lu, J. Liu, X. Hu, T. Yao, Y. Lin, S. Liang, S. Shi and C. Dong, Adv. Mater., 2019, 31, 1904997 CrossRef CAS.
- A. L. Chin, Y. Zhong and R. Tong, Biomater. Sci., 2017, 5, 1491–1499 RSC.
- M.-J. Choi, J.-S. Park, J.-E. Park, H. S. Kim and H.-S. Kim, Biomol. Ther., 2017, 25, 641–647 CrossRef CAS.
- Q. Chen, L. Xu, C. Liang, C. Wang, R. Peng and Z. Liu, Nat. Commun., 2016, 7, 13193 CrossRef CAS.
- C. W. Ng, J. Li and K. Pu, Adv. Funct. Mater., 2018, 28, 1804688 CrossRef.
- Q. Chen, Q. Hu, E. Dukhovlinova, G. Chen, S. Ahn, C. Wang, E. A. Ogunnaike, F. S. Ligler, G. Dotti and Z. Gu, Adv. Mater., 2019, 31, 1970166 CrossRef.
- Y. Li, X. Li, F. Zhou, A. Doughty, A. R. Hoover, R. E. Nordquist and W. R. Chen, Cancer Lett., 2019, 442, 429–438 CrossRef CAS.
- Q. Chen, M. Chen and Z. Liu, Chem. Soc. Rev., 2019, 48, 5506–5526 RSC.
- I. Adjei and S. Blanka, J. Funct. Biomater., 2015, 6, 81–103 CrossRef CAS.
- L. Cheng, C. Wang, L. Feng, K. Yang and Z. Liu, Chem. Rev., 2014, 114, 10869–10939 CrossRef CAS.
- Q. Chen, J. Chen, Z. Yang, J. Xu, L. Xu, C. Liang, X. Han and Z. Liu, Adv. Mater., 2019, 31, 1802228 CrossRef.
- S. Nie, Nanomedicine, 2010, 5, 523–528 CrossRef.
- L. Cheng, K. Yang, Q. Chen and Z. Liu, ACS Nano, 2012, 6, 5605–5613 CrossRef CAS.
- Q. Tian, M. Tang, Y. Sun, R. Zou, Z. Chen, M. Zhu, S. Yang, J. Wang, J. Wang and J. Hu, Adv. Mater., 2011, 23, 3542–3547 CrossRef CAS.
- H.-C. Huang, S. Barua, G. Sharma, S. K. Dey and K. Rege, J. Controlled Release, 2011, 155, 344–357 CrossRef CAS.
- Z. Zha, J. Wang, E. Qu, S. Zhang, Y. Jin, S. Wang and Z. Dai, Sci. Rep., 2013, 3, 2360 CrossRef.
- J. Zhou, Z. Lu, X. Zhu, X. Wang, Y. Liao, Z. Ma and F. Li, Biomaterials, 2013, 34, 9584–9592 CrossRef CAS.
- A. Yuan, X. Tang, X. Qiu, K. Jiang, J. Wu and Y. Hu, Chem. Commun., 2015, 51, 3340–3342 RSC.
- V. Mundra, Y. Peng, S. Rana, A. Natarajan and R. I. Mahato, J. Controlled Release, 2015, 220, 130–140 CrossRef CAS.
- P. Xue, M. Hou, L. Sun, Q. Li, L. Zhang, Z. Xu and Y. Kang, Acta Biomater., 2018, 81, 242–255 CrossRef CAS.
- L. Han, Y. Zhang, X.-W. Chen, Y. Shu and J.-H. Wang, J. Mater. Chem. B, 2016, 4, 105–112 RSC.
- I. Yoon, J. Z. Li and Y. K. Shim, Clin. Endosc., 2013, 46, 7 CrossRef.
- S. Wang, R. Gao, F. Zhou and M. Selke, J. Mater. Chem., 2004, 14, 487 RSC.
- C. Wang, H. Tao, L. Cheng and Z. Liu, Biomaterials, 2011, 32, 6145–6154 CrossRef CAS.
- P. Zhang, W. Steelant, M. Kumar and M. Scholfield, J. Am. Chem. Soc., 2007, 129, 4526–4527 CrossRef CAS.
- J. Dang, H. He, D. Chen and L. Yin, Biomater. Sci., 2017, 5, 1500–1511 RSC.
- C.-P. Liu, T.-H. Wu, C.-Y. Liu, K.-C. Chen, Y.-X. Chen, G.-S. Chen and S.-Y. Lin, Small, 2017, 13, 1700278 CrossRef.
- W. Zhu, Z. Dong, T. Fu, J. Liu, Q. Chen, Y. Li, R. Zhu, L. Xu and Z. Liu, Adv. Funct. Mater., 2016, 26, 5490–5498 CrossRef CAS.
- S. Xu, X. Zhu, C. Zhang, W. Huang, Y. Zhou and D. Yan, Nat. Commun., 2018, 9, 2053 CrossRef.
- Y. Cheng, H. Cheng, C. Jiang, X. Qiu, K. Wang, W. Huan, A. Yuan, J. Wu and Y. Hu, Nat. Commun., 2015, 6, 8785 CrossRef CAS.
- X. Li, N. Kwon, T. Guo, Z. Liu and J. Yoon, Angew. Chem., Int. Ed., 2018, 57, 11522–11531 CrossRef CAS.
- W. Liu, T. Liu, M. Zou, W. Yu, C. Li, Z. He, M. Zhang, M. Liu, Z. Li, J. Feng and X. Zhang, Adv. Mater., 2018, 30, 1802006 CrossRef.
- S. G. Awuah and Y. You, RSC Adv., 2012, 2, 11169 RSC.
- E. Wachter, D. K. Heidary, B. S. Howerton, S. Parkin and E. C. Glazer, Chem. Commun., 2012, 48, 9649 RSC.
- W. R. Chen, R. L. Adams, A. K. Higgins, K. E. Bartels and R. E. Nordquist, Cancer Lett., 1996, 98, 169–173 CrossRef CAS.
- W. R. Chen, R. L. Adams, R. Carubelli and R. E. Nordquist, Cancer Lett., 1997, 115, 25–30 CrossRef CAS.
- L. Zou, H. Wang, B. He, L. Zeng, T. Tan, H. Cao, X. He, Z. Zhang, S. Guo and Y. Li, Theranostics, 2016, 6, 762–772 CrossRef CAS.
- X. Li, M. F. Naylor, H. Le, R. E. Nordquist, T. K. Teague, C. A. Howard, C. Murray and W. R. Chen, Cancer Biol. Ther., 2010, 10, 1081–1087 CrossRef.
- L. Guo, D. D. Yan, D. Yang, Y. Li, X. Wang, O. Zalewski, B. Yan and W. Lu, ACS Nano, 2014, 8, 5670–5681 CrossRef CAS.
- L. Wang, M. Wang, B. Zhou, F. Zhou, C. Murray, R. A. Towner, N. Smith, D. Saunders, G. Xie and W. R. Chen, J. Mater. Chem. B, 2019, 7, 7406–7414 CAS.
- B. Zhou, J. Song, M. Wang, X. Wang, J. Wang, E. W. Howard, F. Zhou, J. Qu and W. R. Chen, Nanoscale, 2018, 10, 21640–21647 RSC.
- Y. Tao, E. Ju, J. Ren and X. Qu, Biomaterials, 2014, 35, 9963–9971 CrossRef CAS.
- C. Wu, X. Guan, J. Xu, Y. Zhang, Q. Liu, Y. Tian, S. Li, X. Qin, H. Yang and Y. Liu, Biomaterials, 2019, 205, 106–119 CrossRef CAS.
- L. Li, S. Yang, L. Song, Y. Zeng, T. He, N. Wang, C. Yu, T. Yin, L. Liu, X. Wei, Q. Wu, Y. Wei, L. Yang and C. Gong, Theranostics, 2018, 8, 860–873 CrossRef CAS.
- Y. Ye, C. Wang, X. Zhang, Q. Hu, Y. Zhang, Q. Liu, D. Wen, J. Milligan, A. Bellotti, L. Huang, G. Dotti and Z. Gu, Sci. Immunol., 2017, 2, eaan5692 CrossRef.
- Y. P. Jia, K. Shi, F. Yang, J. F. Liao, R. X. Han, L. P. Yuan, Y. Hao, M. Pan, Y. Xiao, Z. Y. Qian and X. W. Wei, Adv. Funct. Mater., 2020, 30, 2001059 CrossRef CAS.
- T. Yata, Y. Takahashi, M. Tan, H. Nakatsuji, S. Ohtsuki, T. Murakami, H. Imahori, Y. Umeki, T. Shiomi, Y. Takakura and M. Nishikawa, Biomaterials, 2017, 146, 136–145 CrossRef CAS.
- J. Nam, S. Son, L. J. Ochyl, R. Kuai, A. Schwendeman and J. J. Moon, Nat. Commun., 2018, 9, 1074 CrossRef.
- P. A. Ott, F. S. Hodi and C. Robert, Clin. Cancer Res., 2013, 19, 5300–5309 CrossRef CAS.
- C. Wang, L. Xu, C. Liang, J. Xiang, R. Peng and Z. Liu, Adv. Mater., 2014, 26, 8154–8162 CrossRef CAS.
- L. Huang, Y. Li, Y. Du, Y. Zhang, X. Wang, Y. Ding, X. Yang, F. Meng, J. Tu, L. Luo and C. Sun, Nat. Commun., 2019, 10, 4871 CrossRef.
- S. Kakarla and S. Gottschalk, Cancer J., 2014, 20, 151–155 CrossRef CAS.
- Q. Chen, Q. Hu, E. Dukhovlinova, G. Chen, S. Ahn, C. Wang, E. A. Ogunnaike, F. S. Ligler, G. Dotti and Z. Gu, Adv. Mater., 2019, 31, 1900192 CrossRef.
- J.-X. Fan, Z.-H. Li, X.-H. Liu, D.-W. Zheng, Y. Chen and X.-Z. Zhang, Nano Lett., 2018, 18, 2373–2380 CrossRef CAS.
- L. Galluzzi, O. Kepp and G. Kroemer, EMBO J., 2012, 31, 1055–1057 CrossRef CAS.
- D. V. Krysko, A. D. Garg, A. Kaczmarek, O. Krysko, P. Agostinis and P. Vandenabeele, Nat. Rev. Cancer, 2012, 12, 860–875 CrossRef CAS.
- A. P. Castano, P. Mroz and M. R. Hamblin, Nat. Rev. Cancer, 2006, 6, 535–545 CrossRef CAS.
- I. Cecic, C. S. Parkins and M. Korbelik, Photochem. Photobiol., 2007, 74, 712–720 CrossRef.
- R. W. Steubing, S. Yeturu, A. Tuccillo, C.-H. Sun and M. W. Berns, J. Photochem. Photobiol., B, 1991, 10, 133–145 CrossRef CAS.
- S. Evans, W. Matthews, R. Perry, D. Fraker, J. Norton and H. I. Pass, JNCI, J. Natl. Cancer Inst., 1990, 82, 34–39 CrossRef CAS.
- T. G. St. Denis, K. Aziz, A. A. Waheed, Y.-Y. Huang, S. K. Sharma, P. Mroz and M. R. Hamblin, Photochem. Photobiol. Sci., 2011, 10, 792 RSC.
- K. Lu, C. He, N. Guo, C. Chan, K. Ni, R. R. Weichselbaum and W. Lin, J. Am. Chem. Soc., 2016, 138, 12502–12510 CrossRef CAS.
- K. Ni, T. Luo, G. Lan, A. Culbert, Y. Song, T. Wu, X. Jiang and W. Lin, Angew. Chem., 2020, 132, 1124–1128 CrossRef.
- J. Xu, L. Xu, C. Wang, R. Yang, Q. Zhuang, X. Han, Z. Dong, W. Zhu, R. Peng and Z. Liu, ACS Nano, 2017, 11, 4463–4474 CrossRef CAS.
- K. Ni, T. Aung, S. Li, N. Fatuzzo, X. Liang and W. Lin, Chem, 2019, 5, 1892–1913 CAS.
- D. Wang, T. Wang, J. Liu, H. Yu, S. Jiao, B. Feng, F. Zhou, Y. Fu, Q. Yin, P. Zhang, Z. Zhang, Z. Zhou and Y. Li, Nano Lett., 2016, 16, 5503–5513 CrossRef CAS.
- A. Pezzolo, D. Marimpietri, L. Raffaghello, C. Cocco, A. Pistorio, C. Gambini, M. Cilli, A. Horenstein, F. Malavasi and V. Pistoia, Oncotarget, 2014, 5, 10368–10381 CrossRef.
- G. Yang, L. Xu, Y. Chao, J. Xu, X. Sun, Y. Wu, R. Peng and Z. Liu, Nat. Commun., 2017, 8, 902 CrossRef.
- C. Shi, M. Li, Z. Zhang, Q. Yao, K. Shao, F. Xu, N. Xu, H. Li, J. Fan, W. Sun, J. Du, S. Long, J. Wang and X. Peng, Biomaterials, 2020, 233, 119755 CrossRef.
- H. Wang, X. Han, Z. Dong, J. Xu, J. Wang and Z. Liu, Adv. Funct. Mater., 2019, 29, 1902440 CrossRef.
- W. R. Chen, W.-G. Zhu, J. R. Dynlacht, H. Liu and R. E. Nordquist, Int. J. Cancer, 1999, 81, 808–812 CrossRef CAS.
|
This journal is © The Royal Society of Chemistry 2020 |