DOI:
10.1039/D0BM00709A
(Paper)
Biomater. Sci., 2020,
8, 3926-3934
Development of dual anti-biofilm and anti-bacterial medical devices†
Received
1st May 2020
, Accepted 10th June 2020
First published on 10th June 2020
Abstract
The rising occurrence of antimicrobial resistance demands new strategies for delivering antibiotics to ensure their effective use. In this study, a multi-functional strategy to address medical device associated infections is explored whereby an anti-attachment and an antibacterial mechanism have been combined. Silicone catheters impregnated with multiple antibiotics are coated with polyacrylate coatings previously shown to reduce bacterial attachment and biofilm formation. Antibiotics are delivered through the applied coating and the delivery rate depends on the coating thickness and the calculated log P. Coated devices achieve a zone of inhibition and TK100 to Gram-negative Escherichia coli and Gram-positive Staphylococcus aureus similar to those of uncoated devices, whilst maintaining anti-attachment properties. No adverse immunological responses of the coatings were observed. The multi-functional nature of the device developed in the study represents an important approach to combatting medical device associated infections.
Introduction
Antibiotic resistance is rising to dangerous levels in all areas of the world, decreasing the effectiveness of disease treatments and placing increasing pressure on the global health system.1 One issue reducing the efficacy of antibiotic treatments is biofilm formation; after adhering to a surface, planktonic bacteria form biofilms, which are up to 1000 times less susceptible to antimicrobial treatments and host defences.2 Around 80% of healthcare-acquired infections are believed to be associated with biofilm formation, with catheter-associated urinary tract infections (CAUTIs) one of the most common infections found in patients.2,3 The duration of catheterisation has a direct influence on the likelihood and type of infection, with treatment considerations often varying between long-term (>28 days) and short-term catheter infections.3b Long-term, indwelling catheterisation can be required in cases of chronic debilitating illness with loss of mobility, such as multiple sclerosis, or in patients with a condition affecting nerves that control the bladder, such as spinal injury. The daily risk of bacterial colonisation when an indwelling catheter is in place is 3–7%; patients requiring extended catheter use are therefore at high risk of bacterial infection and those with chronic indwelling catheters are assumed to be bacteriuric.3b
Development and implementation of novel biomaterials able to resist biofilm formation is an attractive target for use in a variety of biomedical applications including urinary catheters. A range of synthetic polymeric materials able to resist bacterial attachment have been reported including zwitterionic polymers and polymeric ammonium salts,4 poly(ethylene glycol)5 and polyglycerols,6 and polyacrylates and polymethacrylates.7 In particular, polyacrylates with molecular stiff hydrophobic pendant groups offer broad spectrum resistance to biofilm formation,8 which has also been demonstrated in vivo.7b The mechanism by which these materials prevent bacterial biofilm has not yet been established, however, the polymers do not kill bacteria and the importance of a bulky hydrophobic moiety suggests possible interactions with the lipophilic cell wall or preferential adsorption of hydrophobic biomolecules that mediate the biological response.7,8 These materials can be engineered as flexible coatings adhered to silicone such that they are suitable for use with urinary catheters.9 However, these materials have yet to be shown to resist biofilm formation for long-term periods of 28 days or more.
An alternative approach to developing anti-biofilm coatings involves the release of antimicrobials from impregnated materials. Antiseptics such as chlorhexidine10 and silver containing compounds have been employed;11 however, toxicity issues and limited evidence of efficacy question the viability of these approaches.12 McCoy et al. developed a pH-responsive antimicrobial release system using polymerised drug conjugates in an effort to extend the therapeutic release time beyond that often observed with diffusion-controlled release.13 However, such an approach restricts use to a narrow range of infections, and efficacy may be decreased in complex cases. Bayston et al. developed a method to impregnate silicone catheters with multiple antimicrobials. This methodology successfully prevented colonisation by a wide range of pathogens for over 12 weeks.3a,14
We hypothesised that this approach, used in conjunction with polyacrylate coatings resistant to biofilm formation, would allow for the development of infection-resistant devices with reduced dependence on antimicrobials and optimised antimicrobial release profiles suitable for long-term use. Hence, the coating would both prevent biofilm formation and control the release of antimicrobials. Here, the coating would be intended to prevent formation of biofilm on a urinary catheter rather than for the treatment of established urinary tract infections.
Results and discussion
Validation of anti-biofilm performance
Four monomers of varying hydrophobicity were polymerised using thermally-initiated free-radical polymerisation to provide a homopolymer library of coatings (Fig. 1); two of these polymers, poly-tert-butyl cyclohexyl acrylate (p-tBCHA, Fig. 1a) and poly-ethylene glycol dicyclopentyl ether acrylate (p-EGDPEA, Fig. 1b), have been previously shown to resist biofilm formation, whilst poly-di(ethylene glycol) methyl ether methacrylate (p-DEGMA, Fig. 1c) and poly-2-hydroxy-3-phenoxypropyl acrylate (p-HPhOPA, Fig. 1d) both supported biofilm formation.7b The four polymers were selected due to their range in hydrophobicity, with calculated log
P (cLog
P) values varying from 0.89 to 4.7 (Fig. 1). Successful polymerisation of the monomers was confirmed by NMR (Fig. SI1–3†).
 |
| Fig. 1 (a–d) Monomers used in the study (cLog P calculated using ChemDraw 16.0 software). | |
Initially the anti-biofilm nature of the silicone sections coated with 1 wt% solutions of each polymer was assessed. Coating thickness was measured by SEM to be 0.5–3 μm (Fig. 2). Variations in coating thickness were likely due to viscosity differences between the different polymer solutions.15 Samples were incubated with S. aureus for 72 h before staining with SYTO64 and imaging by confocal microscopy. Samples were assessed with S. aureus as this species produced higher surface coverages of bacterial biofilm on uncoated plain catheters (45.9 ± 22.5%) compared with E. coli (3.6 ± 3.1%). The bacterial biofilm coverages measured on each coating are shown in Fig. 3. A statistically significant (p < 0.0001) reduction in bacterial coverage of >97% was observed on the coatings of p-tBCHA and p-EGDPEA compared with the uncoated catheters. This was consistent with previous observations for these coatings,7 suggesting that the coating methodology maintained the anti-biofilm properties. The p-DEGMA coating also significantly (p = 0.002) reduced the bacterial coverage by 73% compared with the uncoated sample, whilst the bacterial coverage on the p-HPhOPA coating was not statistically different from the uncoated catheter samples.
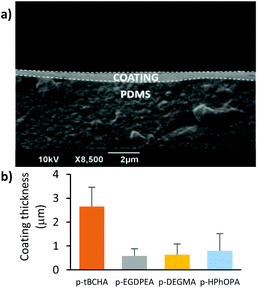 |
| Fig. 2 (a) Representative SEM image of the cross-sectional view of a coated catheter showing coating thickness. (b) Measured coating thickness for all four polymers using a polymer solution concentration of 1% (w/v). Scale bars equal one standard deviation, n = 5. | |
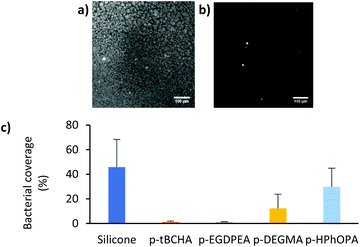 |
| Fig. 3 (a and b) Representative fluorescence images of bacterial coverage measured on (a) uncoated PDMS and (b) p-tBCHA coated samples. (c) S. aureus coverage after 72 h culture with uncoated and polymer coated catheters. Bacteria were stained with SYTO64 (Ex = 599 nm, Em = 619 nm) and imaged by confocal microscopy. Average of 4 technical replicates taken from two biological replicates, N = 2. Error bars equal ± 1 standard deviation unit. | |
Antimicrobial delivery experiments
Next, the delivery of antimicrobials through a polymer coating and how polymer hydrophobicity influenced antimicrobial release was investigated. Silicone catheter sections were impregnated with the antimicrobials rifampicin, sparfloxacin and triclosan according to a previously published procedure.16 Improved prevention of biofilm formation and emergence of resistant strains has previously been demonstrated when using multiple antimicrobials compared with a single drug.3a,14c These compounds were chosen due to their activity against CAUTI pathogens and chemical compatibility with the impregnation procedure.14b Rifampicin was included because of its activity alone and in combination against staphylococci and its synergistic activity against many mutlidrug-resistant Gram negative bacteria.17 Sparfloxacin has been withdrawn from the market as it has been overtaken by other quinolone antimicrobials, but it is still available for medical applications, is active against CAUTI pathogens and is safe for use in the lower and upper urinary tracts.18 Triclosan is approved for use in medicinal products within the EU and by the FDA and is a component of Vicryl Plus surgical sutures.19 The impregnated catheter sections were dip-coated 3 times in a 1–5 wt% polymer in toluene solution and dried for 24 h under vacuum (Fig. 4). Non-impregnated plain silicone catheter controls were also dip-coated similarly using the four polymer solutions.
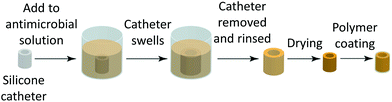 |
| Fig. 4 Schematic depiction of the production of coated and impregnated catheters. | |
Serial plate transfer tests were carried out for 10 days on the coated, impregnated catheters using plates inoculated with Staphylococcus aureus (S. aureus) and Escherichia coli (E. coli) and compared to the uncoated impregnated control (Fig. 5). All four polymer coatings showed zones of inhibition for both bacterial species indicating that antimicrobials could be successfully delivered through a poly(meth)acrylate coating. However, the zones of inhibition were reduced relative to that of the impregnated catheters without a polymer coating. No correlation between the cLog
P of the monomer, as a measure of hydrophobicity,8a,c and zone of inhibition size could be seen (see Fig. SI4†). Coatings of p-tBCHA prepared using a 5 wt% solution did not achieve a zone of inhibition with E. coli (Fig. 5b), however, reducing the solution concentration to 1 wt% achieved a zone of inhibition of ≈90% of an uncoated catheter (Fig. SI5†).
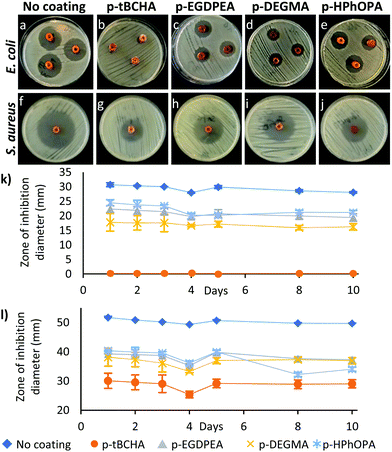 |
| Fig. 5 Assessment of the zone of inhibition for antimicrobial impregnated catheters with (a–e), E. coli and (f–j) S. aureus for (a and f) no coating, (b and g) p-tBCHA, (c and h) p-EGDPEA, (d and i) p-DEGMA, and (e and j) p-HPhOPA. Photographs after 1 day. Samples located in 90 mm Petri dishes. (k) 10 day serial plate transfer, E. coli (l) 10 day serial plate transfer, S. aureus. Error bars equal ± one standard deviation unit, N = 3. | |
Next, a series of assays of the time taken to kill 100% of attached E. coli (tK100) were carried out on the coated catheters to determine whether the coatings were still able to kill all attached bacteria (Fig. 6a). The attached bacteria on the catheters coated with p-DEGMA or p-HPhOPA were reduced after 24 h but could not be taken to <1 Log10 cfu per mL after 72 h, suggesting that antimicrobial permeability through these coatings was reduced. Catheters coated with p-EGDPEA and p-tBCHA were both able to reach <1 Log10 cfu per ml after 72 h. In comparison, the uncoated impregnated catheter reached <1 Log10 cfu per mL viability at 48 h. There was no difference in viability between plain silicone catheter and plain silicone catheter coated with the polymers (Fig. 6b). Thus, the coatings that were more hydrophobic showed increased permeation of the antimicrobials compared with the more hydrophilic coatings despite an increased or similar thickness (Fig. 2b). No significant difference in the amount of bacteria on the different coatings was observed in the absence of impregnated anti-microbials, despite the different chemistries producing significantly different amounts of biofilm formation.7 Thus, the polyacrylate coatings did not prevent association with planktonic bacteria, which is consistent with the mode of action of these polymers acting specifically on biofilm formation and not involving a killing mechanism.
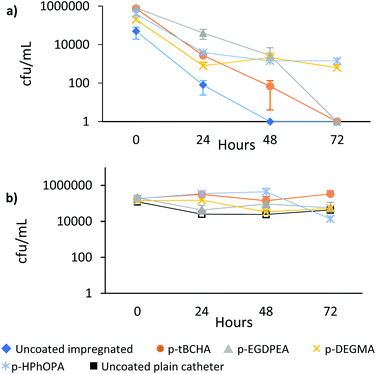 |
| Fig. 6 Assessment of the ability of catheters impregnated with antimicrobials and coated with different polymers to kill associated bacteria. (a) tK100 for impregnated catheters (b) tK100 for catheters without impregnated antimicrobials. Error bars equal ± one standard deviation unit, N = 3. | |
Quantification of antimicrobial release
With the initial bacterial studies indicating a reduction in antimicrobial release through the polymer coatings, we next quantified drug elution from the catheters. The catheter sections were immersed in deionised water for 48 h at 37 °C and the solution analysed by liquid chromatography mass spectrometry (LC-MS). The percentage of drug elution (Fig. SI7–13†) was normalised relative to the uncoated impregnated catheter set at 100% and plotted against cLog
P (Fig. 7). No monomer or coating oligomers were detected in the analysed solutions suggesting that the coating was stable in the aqueous environment over 48 h. Antimicrobial release was achieved through all four polymer coatings although at a reduced quantity as compared with the uncoated impregnated catheter. The smallest reduction for all three antibiotics was observed for the coating of tBCHA, where 43%, 97% and 90% of sparfloxacin, triclosan and rifampicin was released, respectively, compared with the uncoated catheter (Fig. 7a–c). p-HPhOPA exhibited the greatest reduction in sparfloxacin release, with 15 μg mL−1 detected compared to 91 μg mL−1 for the uncoated sample (Fig. 7a). For the other coated samples a range of 24–39 μg mL−1 detected concentration was observed. p-DEGMA showed the greatest reduction in triclosan release, with 50 μg mL−1 detected compared to 78 μg mL−1 detected for the uncoated catheter (Fig. 7b). A correlation between cLog
P and percentage elution of triclosan was seen, suggesting that intermolecular interactions between the antibiotic and the polymers may play a role in the drug elution (Fig. 7b and d). Rifampicin release was most affected by the p-DEGMA coating; in 2 out of 3 repeats, the level of rifampicin was shown to be below the limit of detection on the LC-MS system, making the detected level statistically non-significant (Fig. 7c). The other 3 coatings exhibited a reduced effect on rifampicin release, delivering around 1.3 μg mL−1versus the uncoated sample at 1.5 μg mL−1.
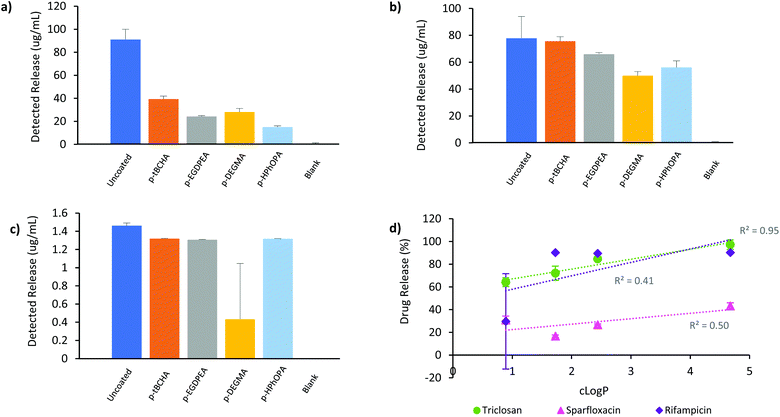 |
| Fig. 7 LC-MS assessment of the antimicrobial release from impregnated catheters through different polymer coatings. (a) Sparfloxacin drug elution, (b) triclosan drug elution, (c) rifampicin drug elution, (d) percentage of drugs eluted through coatings relative to uncoated catheter against cLog P. The line of best fit and associated coefficient of determination (R2) is shown for each antibiotic: triclosan (green), sparfloxacin (yellow) and rifampicin (orange). Error bars equal ± 1 standard deviation unit, n = 3. | |
These results were consistent with the tK100 tests, where only p-tBCHA and p-EGDPEA coatings were able to reach <1 Log10 cfu per ml after 72 h (Fig. 6a). p-tBCHA showed the greatest level of detected drug elution using LC-MS with all 3 antimicrobials, possibly due to reduced intermolecular interactions between the antibiotics and the polymer. The p-tBCHA coating was thicker than the other samples (Fig. 2b), which was anticipated to impede antimicrobial release. Counter to this, relatively high drug release was observed through the p-tBCHA coating compared with the other samples (Fig. 7), thus, it was likely that intermolecular interactions rather than coating thickness played the key role in determining drug elution through the samples studied. It is important to note that the zone of inhibition and tK100 tests were not carried out with the catheter sections fully immersed in solution, which may have resulted in less coating swelling and consequently more restricted drug elution. There was no strong correlation between cLog
P and elution of rifampicin or sparfloxacin (R2 = 0.41 and R2 = 0.50 respectively, Fig. 7d); in contrast, triclosan elution showed a strong correlation to cLog
P (R2 = 0.95, R2(adj) = 0.92, p = 0.03, Fig. 7d) It is possible that the bulkier molecules were more affected by the polymer network than triclosan, making hydrophobicity of the coating less of a factor determining release.
Coating characterisation
Time of flight secondary ion mass spectrometry (ToF-SIMS) depth profiling analysis, using an Ar-cluster sputter beam at 10 keV with Bi3+ primary beam at 25 keV over a random raster area, was then used to assess the depth distribution of the coating and antimicrobials within the coated, impregnated devices. Molecular ions for all 3 antimicrobials could be detected; rifampicin (M + H+ = C43H59N4O12) at 823.4, sparfloxacin (M + H+ = C19H23F2N4O3) at 393.2 and triclosan (M + H+ = C12H8Cl3O2) at 289.0, whilst characteristic ions for p-tBCHA (C4H9+), p-EGDPEA (C5H7+), p-DEGMA (C3H7O+) and p-HPhOPA (C6H5+) were also identified.20 Both the p-tBCHA and p-EGDPEA coatings appeared to have a lower intensity of antimicrobial ions in the polymer coating, which increased to a maximum at the interface between the coating and silicone catheter before decreasing again (Fig. 8a and b). The more hydrophilic polymer coatings p-DEGMA and p-HPhOPA appeared to have greatest intensity of antimicrobial peaks in the coating, which then decreased in line with the coating ion intensities (Fig. 8c and d). It is possible that during the coating procedure more of the impregnated antimicrobials leached out into the polymers p-DEGMA and p-HPhOPA than p-tBCHA and p-EGDPEA. This may also account for the observed discrepancy between the zone of inhibition and tk100 tests and the detected drug elution in the LC-MS studies; if the rate of drug elution through the polymers differed between samples, then an increased initial concentration of drug in the coating may have produced a greater biological response. The uncoated impregnated sample showed uniform antimicrobial distribution through the profiled area (see Fig. SI14–16†).
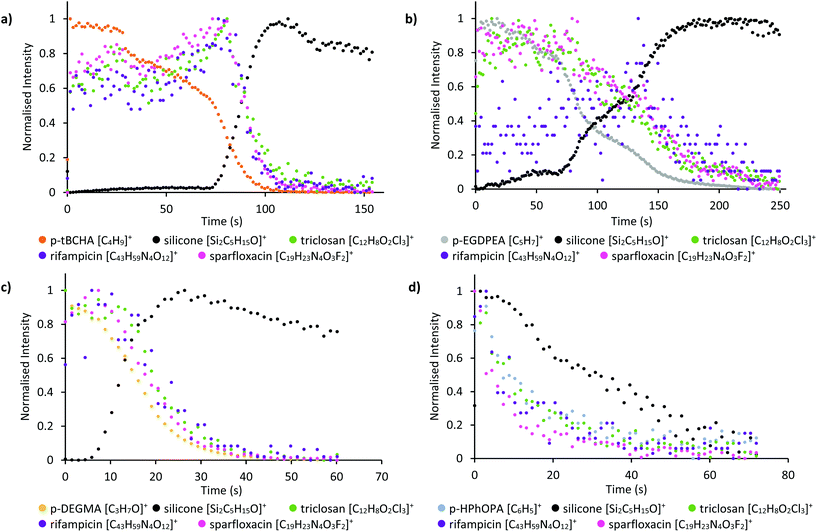 |
| Fig. 8 Normalised ToF-SIMS depth profile analysis for (a) p-tBCHA coated impregnated catheter, (b) p-EGDPEA coated impregnated catheter, (c) p-DEGMA coated impregnated catheter, and (d) p-HPhOPA coated impregnated catheter. Normalised ion intensities for ions characteristic of the polymer coatings, the antimicrobials and silicone are shown. | |
Biocompatibility studies
The components of the dual anti-biofilm anti-microbial device have previously been demonstrated to not be cytotoxic.17–19,21 To further assess the biocompatibility of polymer coatings, primary human monocyte-derived macrophages were incubated with the coated or uncoated plain or antibiotic-impregnated catheters for 24 h. The effect of polymer coatings on macrophage function was assessed by stimulating these cells with lipopolysaccharide (LPS) for a further 24 h. Cell viability before and after LPS addition was determined by measuring the amount of lactate dehydrogenase in the cell supernatants (ESI†). None of the coatings tested appeared to increase cell death appreciably compared to a macrophage only control (no catheter condition).
To determine the effect of the coatings on immune cell function, the production of two pro-inflammatory cytokines: tumour necrosis factor alpha (TNF-α) and interleukin 6 (IL-6), by macrophages was also measured before and after addition of LPS (Fig. 9a). Macrophages did not produce the pro-inflammatory cytokines TNF-α or IL-6 when incubated with coated or uncoated plain catheters alone, i.e. in “unstimulated samples” (Fig. 9b and c). Thus, the catheters or coating polymers did not cause inappropriate macrophage activation.
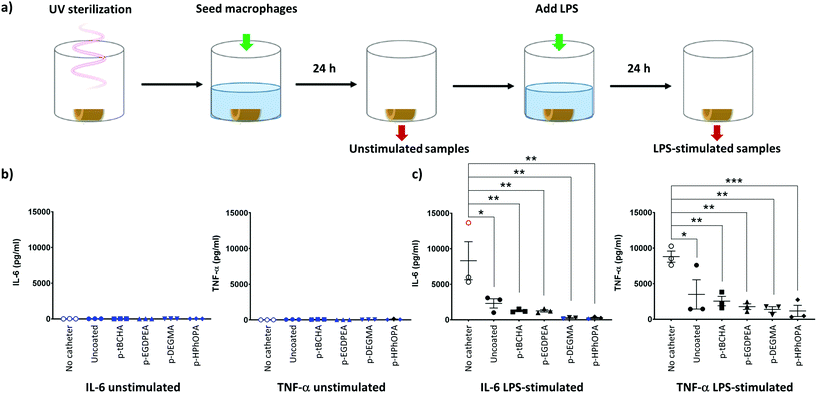 |
| Fig. 9 Effect of catheter coatings on primary human monocyte-derived macrophages. (a) Schematic representation of experimental procedure for macrophage-catheter assays for cytokine determination. (b and c) TNF-α and IL-6 production from macrophages that had been incubated with coated and uncoated plain catheters for (b) 24 h (unstimulated) and (c) followed by stimulation with 100 ng ml−1 LPS for 24 h (LPS-stimulated). Graphs represent mean ± SEM for n = 3 (3 independent experiments using 3 separate donors). ANOVA with Tukey's multiple comparison post-test conducted, *, p ≤ 0.05, **, p ≤ 0.01, and ***, p ≤ 0.001. Blue symbols denote values that were below the standard range of the assay. Red symbols denote values that were above the standard range of the assay. | |
Upon LPS stimulation, macrophages alone (i.e. in the absence of catheters) produced TNF-α and IL-6 (Fig. 9b and c), as expected. Significantly lower TNF-α and IL-6 production was seen by LPS-stimulated macrophages in all catheter samples (uncoated and coated) (Fig. 9b, c and Fig. SI17†), indicating that these samples were suppressing the normal inflammatory response. Further work would be necessary to determine the cause of this effect.
Materials and methods
General information
All polymerisation reactions were carried out under an atmosphere of argon using degassed monomer solutions. Degassing was carried out by bubbling argon through the monomer solution for 40 minutes immediately before use. Monomers and 2,2′-azobis(2-methylpropionitrile) (AIBN) were purchased from Sigma-Aldrich and were used as received. All temperatures are referred to the temperatures of the oil baths used. Nuclear magnetic resonance (NMR) spectra were recorded on a Bruker AV400 (400.3 MHz) spectrometer at ambient temperature; chemical shifts are quoted in parts per million (ppm) and were referenced as follows: chloroform-d, 7.26 ppm; benzene-d6 7.16 ppm for 1H NMR data.22 Coupling constants (J) are quoted in Hertz. Gel Permeation Chromatography (GPC) was carried out on a Polymer Labs GPC 50 with 2 × PLgel Mixed-D columns and N,N-dimethylformamide containing 0.1 wt% LiBr eluent. GPC calibration was carried out using poly(methyl methacrylate).
Polymerisation reactions
All polymerisation reactions were carried out under an atmosphere of argon using degassed monomer solutions. Degassing was carried out by bubbling argon through the monomer solution for 40 minutes immediately before use. Monomers and 2,2′-azobis(2-methylpropionitrile) (AIBN) were purchased from Sigma-Aldrich and were used as received. All temperatures are referred to the temperatures of the oil baths used. Nuclear magnetic resonance (NMR) spectra were recorded on a Bruker AV400 (400.3 MHz) spectrometer at ambient temperature; chemical shifts are quoted in parts per million (ppm) and were referenced as follows: chloroform-d, 7.26 ppm; benzene-d6 7.16 ppm for 1H NMR data.22 Coupling constants (J) are quoted in Hertz. Gel Permeation Chromatography (GPC) was carried out on a Polymer Labs GPC 50 with 2 × PLgel Mixed-D columns and N,N-dimethylformamide containing 0.1 wt% LiBr eluent. GPC calibration was carried out using poly(methyl methacrylate).
Antimicrobial impregnation of catheters
Silicone catheter tubing with a lumen diameter of 3.2 mm (Appleton Woods) was impregnated with sparfloxacin (SIGMA), triclosan (CIBA) and rifampicin (SIGMA) dissolved in chloroform to give concentrations of 1%, 1% and 0.2% respectively (Fig. 4), as described previously.16
Coating of catheters
Catheters were cut into sections measuring 1 cm in length. These were then attached to a 21 gauge needle by piercing the catheter wall with the needle and immersed by hand into a monomer solution of desired concentration (5 wt% or 1 wt%) in toluene for 1 s before being removed and left to dry under ambient conditions for 15 minutes. This procedure was repeated twice before the catheter sections were placed under vacuum (<1 mbar) for 24 h. Coating thickness was determined using a JEOL JSM-6490LV SEM using an accelerator voltage of 10 kV. Prior to analysis, coated samples were fractured after immersion in liquid nitrogen to expose the sample cross-section. Samples were mounted on carbon tape and gold coated using a Polaron SC7640 sputter coater, sputter time was 90 s, plasma current was 18–19 mA and a base chamber pressure of 0.6 mbar was achieved prior to coating.
Liquid chromatography mass spectrometry
Liquid chromatography mass spectrometry (LC-MS) was carried out on a ThermoFisher Exactive using a Sunfire C18 column stationary phase. Mobile phase was gradient elution from 95
:
5 0.1% NH3 in H2O
:
CH3CN to 5
:
95 0.1% NH3 in H2O
:
CH3CN with a flow rate of 0.800 mL min−1. Experiment stop time was 10.00 min.
Time-of-flight secondary ion mass spectrometry
Dual beam depth profiling time-of-flight secondary ion mass spectrometry (ToF-SIMS) measurements were conducted using a ToF-SIMS IV (IONTOF GmbH) instrument operated using a 25 kV Bi3+ primary ion source in combination with a 10 kV Arn+ sputter beam. Samples were scanned with a random raster over an area of 200 × 200 μm. Positive secondary ion spectra were collected. Owing to the non-conductive nature of the samples, a low energy (20 eV) electron flood gun was applied to provide charge compensation.
Serial plate transfer test assay
Test bacteria, methicillin resistant Staphylococcus aureus (MRSA) and extended spectrum beta-lactamases producing E. coli, were isolated from patients with CAUTI. MRSA was sensitive to rifampicin and triclosan, but resistant to sparfloxacin. E. coli was resistant to both rifampicin and sparfloxacin but susceptible to triclosan. Minimum inhibitory concentrations were determined by agar incorporation or in the case of rifampicin, by Etest (AB Biodisk, Solna, Sweden). A serial transfer of the material to fresh plates will show how long the material produces a zone of inhibition (Serial Plate Transfer Test, SPTT).23 Mueller Hinton agar (Oxoid) plates were seeded with the test bacteria (A630 0.6, ∼1 × 107 cfu mL−1) and impregnated silicone catheters with and without a coating were placed in triplicate on their surfaces and incubated overnight. Zones of inhibition were measured with calipers and the catheters were transferred to a fresh seeded plate and incubated at 37 °C for 24 h. The process was repeated for up to 10 days.
tK100 assay
The plain and test catheters were immersed in a suspension (approximately 1 × 108 cfu mL−1) of early log phase test bacteria and incubated at 37 °C for 1 h for attachment to take place. After rinsing to remove unattached bacteria, triplicates of discs were placed in diluted Tryptone Soya Broth (Oxoid) for up to 72 h, the dilution necessary for survival of attached bacteria to controls without planktonic multiplication, being found by experiment for each test isolate. At intervals of 0, 24, 48 and 72 h, after rinsing and medium replacement each day, triplicates of catheters were removed and sonicated (50 Hz for 20 min) and surviving colonies plate – counted.
Generation of monocyte-derived macrophages
Monocyte-derived macrophages were generated from buffy coats (Blood Transfusion Service, Sheffield, UK). Peripheral blood mononuclear cells (PBMCs) were isolated by density gradient centrifugation using Histopaque-1077 (Sigma-Aldrich, UK). The PBMC layer was collected, washed and the monocyte fraction (CD14+ cells) was obtained by positive selection using human CD14 MicroBeads and LS MACS columns (Miltenyi Biotec, UK), following the manufacturer's instructions. Purified monocytes were re-suspended in RPMI complete medium RPMI 1640 (Sigma-Aldrich, UK) containing 15% human AB serum (Sigma-Aldrich, UK), 2 mM GlutaMAX (Gibco, UK), 10 mM HEPES (Invitrogen, UK) and 50 ng mL−1 recombinant human macrophage colony stimulating factor (rhM-CSF, premium grade, Miltenyi Biotec, UK) and plated on ultra-low attachment 24-well flat bottom plates (Corning incorporated, USA) at a density of 1 × 106 monocytes per 500 μL. On Day 3, 500 μL per well of fresh RPMI complete medium containing 50 ng mL−1 rhM-CSF was added. Macrophages were harvested on Day 6 and used for assays with catheters.
Macrophage response to catheters
Catheters were placed in a 24-well tissue culture-treated (TC) plate (Costar, UK) and UV-sterilised for 30 min. Day 6 macrophages were harvested, washed once with RPMI complete medium, counted, and seeded in the 24-well TC plate containing sterilised catheters at 2.5 × 105 cells per 750 μL per well in RPMI complete medium containing 50 ng mL−1 rhM-CSF. The volume of culture medium was sufficient to completely submerge the catheters. Cells were incubated with catheters for 24 h at 37 °C, 5% CO2 in a humidified incubator. The following day, supernatants were removed from each well and stored at −20 °C for cytokine determination (referred to in the text as “unstimulated samples”). 100 ng mL−1 ultrapure LPS from E. coli (Invivogen, UK) in fresh RPMI complete medium containing 50 ng mL−1 rhM-CSF was then added and the cells were re-incubated for a further 24 h at 37 °C, 5% CO2 in a humidified incubator. Supernatants from each well were collected the following day and stored at −20 °C for cytokine analysis (referred to in the text as “LPS-stimulated samples”). Macrophage supernatants (appropriately diluted) were tested for presence of the cytokines TNF-α and IL-6 using DuoSet ELISA kits (R&D Systems, Inc., UK) following the manufacturer's instructions. To assess macrophage viability, uncoated or coated antibiotic-impregnated catheters were placed in a 24-well tissue culture-treated (TC) plate (Eppendorf) and UV-sterilised for 30 min. Day 6 macrophages were harvested, washed 3 times with X-Vivo 15 serum-free medium (Lonza, UK), counted, and seeded in the 24-well TC plate containing sterilised catheters at 2.5 × 105 cells per 1.5 mL per well in X-Vivo 15 medium containing 50 ng mL−1 rhM-CSF. The volume of culture medium was sufficient to completely submerge the catheters. Cells were incubated with catheters for 24 h at 37 °C, 5% CO2 in a humidified incubator. The following day, 750 μL of cell supernatants were removed from each well and stored at −20 °C for lactate dehydrogenase (LDH) determination (referred to in the text as “unstimulated samples”). 100 ng mL−1 ultrapure LPS from E. coli (Invivogen, UK) was then added and the cells with catheters were re-incubated for a further 24 h. The remaining 750 μL of cell supernatants from each well were collected the following day and stored at −20 °C for LDH analysis (referred to in the text as “LPS-stimulated samples”). LDH in the supernatants was measured using the Cytotoxicity Detection Kit (LDH) kit (Roche, UK) as per the manufacturer's instructions.
Statistical analysis
Linear regression analysis was carried out using the built-in linear model function in R version 3.6.1. Statistical analysis for macrophage cytokine responses was carried out in Prism v8.2.1, a repeated measures ANOVA with Tukey's multiple comparison post-test was used.
Conclusions
In summary, two different strategies for preventing bacterial biofilm formation on catheters have been combined. We have shown that multiple antimicrobials can be delivered through polyacrylate coatings on impregnated silicone catheters. The rate of delivery through the coating was modulated by the coating chemistry and thickness. The zone of inhibition and prevention of biofilm formation was not adversely affected on the optimised device after application of the anti-biofilm coating. Surface characterisation and depth profiling by ToF-SIMS confirmed the presence of the polymer coatings on the devices and demonstrated the ability of the antibiotics to permeate through the polyacrylate layer. No adverse immunological response was observed to the coatings. The multi-functional nature of the device developed in the study makes it an important approach to combatting medical device associated infections.
Conflicts of interest
There are no conflicts to declare.
Acknowledgements
This work was supported by the Bridging the Gaps project at The University of Nottingham, funded by the Engineering and Physical Sciences Research Council grant reference EP/M027333/1. Assistance with ToF-SIMS measurements by David Scurr and with the macrophage assays by Georgina Taylor is kindly acknowledged. Andrew Hook kindly acknowledges the University of Nottingham for funding his Nottingham Research Fellowship.
Notes and references
-
F. M. Aarestrup, A. Aidara-Kane, N. van de Sande-Bruinsma, D. Falzon, H. Grundmann, M. Lahra, E. Mathai, M. L. Narasimhan, C. Oxenford, P. R. Pardo, P. Ringwald, A. Sharma, J. Stelling, K. Weerasuriya and M. Zignol, Antimicrobial Resistance: Global Report on Surveillance, ed. H. Cadman and L. Martinez, World Health Organisation, 2014 Search PubMed
.
- R. D. Monds and G. A. O'Toole, Trends Microbiol., 2009, 17, 73–87 CrossRef CAS PubMed
.
-
(a) L. E. Fisher, A. L. Hook, W. Ashraf, A. Yousef, D. A. Barrett, D. J. Scurr, X. Chen, E. F. Smith, M. Fay, C. D. J. Parmenter, R. Parkinson and R. Bayston, J. Controlled Release, 2015, 202, 57–64 CrossRef CAS PubMed
;
(b) L. E. Nicolle, Antimicrob. Resist. Infect. Control, 2014, 3, 23 CrossRef PubMed
.
-
(a) G. Cheng, Z. Zhang, S. F. Chen, J. D. Bryers and S. Y. Jiang, Biomaterials, 2007, 28, 4192–4199 CrossRef CAS PubMed
;
(b) X. Xie, J. C. Doloff, V. Yesilyurt, A. Sadraei, J. J. McGarrigle, M. Commis, O. Veiseh, S. Farah, D. Isa, S. Ghanis, I. Joshis, A. Vegas, J. Li, W. Wang, A. Bader, H. H. Tam, J. Tao, H.-J. Chen, B. Yang, K. A. Williamson, J. Oberholzers, R. Langer and D. G. Anderson, Nat. Biomed. Eng., 2018, 2, 894–906 CrossRef CAS PubMed
;
(c) C. Zhou, Y. Wu, K. R. V. Thappeta, J. T. L. Subramanian, D. Pranantyo, E. T. Kang, H. W. Duan, K. Kline and M. B. Chan-Park, ACS Appl. Mater. Interfaces, 2017, 9, 36269–36280 CrossRef CAS PubMed
.
-
(a) I. Banerjee, R. C. Pangule and R. S. Kane, Adv. Mater., 2011, 23, 690–718 CrossRef CAS PubMed
;
(b) M. Li, K. G. Neoh, L. Q. Xu, R. Wang, E. T. Kang, T. Lau, D. P. Olszyna and E. Chiong, Langmuir, 2012, 28, 16408–16422 CrossRef CAS PubMed
.
- Q. Wei, T. Becherer, P. L. M. Noeske, I. Grunwald and R. Haag, Adv. Mater., 2014, 26, 2688–2693 CrossRef CAS PubMed
.
-
(a) A. L. Hook, C. Chang, J. Yang, S. Atkinson, R. Langer, D. G. Anderson, M. C. Davies, P. Williams and M. R. Alexander, Adv. Mater., 2013, 25, 2542–2547 CrossRef CAS PubMed
;
(b) A. L. Hook, C. Chang, J. Yang, J. Luckett, A. Cockrayne, S. Atkinson, Y. Mei, R. Bayston, D. J. Irvine, R. Langer, D. G. Anderson, P. Williams, M. C. Davies and M. R. Alexander, Nat. Biotechnol., 2012, 30, 868–875 CrossRef CAS PubMed
.
-
(a) A. A. Dundas, O. Sanni, J. Dubern, G. Dimitrakis, A. L. Hook, D. J. Irvine, P. Williams and M. R. Alexander, Adv. Mater., 2019, 1903513 CrossRef CAS PubMed
;
(b) P. Mikulskis, A. L. Hook, A. Dundas, D. J. Irvine, O. Sanni, D. G. Anderson, R. Langer, M. R. Alexander, P. Williams and D. A. Winkler, ACS Appl. Mater. Interfaces, 2018, 10, 139–149 CrossRef CAS PubMed
;
(c) O. Sanni, C. Chang, D. G. Anderson, R. Langer, M. C. Davies, P. M. Williams, P. Williams, M. R. Alexander and A. L. Hook, Adv. Healthcare Mater., 2015, 4, 695–701 CrossRef CAS PubMed
.
-
(a) K. Adlington, N. T. Nguyen, E. Eaves, J. Yang, C.-Y. Chang, J. Li, A. L. Gower, A. Stimpson, D. G. Anderson, R. Langer, M. C. Davies, A. L. Hook, P. Williams, M. R. Alexander and D. J. Irvine, Biomacromolecules, 2016, 17, 2830–2838 CrossRef CAS PubMed
;
(b) E. P. Magennis, A. L. Hook, P. Williams and M. R. Alexander, ACS Appl. Mater. Interfaces, 2016, 8, 30780–30787 CrossRef CAS PubMed
;
(c) B. J. Tyler, A. Hook, A. Pelster, P. Williams, M. Alexander and H. F. Arlinghaus, Biointerphases, 2017, 12, 02C412 CrossRef PubMed
.
- R. Hachem, R. Reitzel, A. Borne, Y. Jiang, P. Tinkey, R. Uthamanthil, J. Chandra, M. Ghannoum and I. Raad, Antimicrob. Agents Chemother., 2009, 53, 5145–5149 CrossRef CAS PubMed
.
-
(a) R. Pickard, T. Lam, G. MacLennan, K. Starr, M. Kilonzo, G. McPherson, K. Gillies, A. McDonald, K. Walton, B. Buckley, C. Glazener, C. Boachie, J. Burr, J. Norrie, L. Vale, A. Grant and J. N'Dow, Lancet, 2012, 380, 1927–1935 CrossRef CAS PubMed
;
(b) D. M. Riley, D. C. Classen, L. E. Stevens and J. P. Burke, Am. J. Med., 1995, 98, 349–356 CrossRef CAS PubMed
.
- T. B. L. Lam, M. I. Omar, E. Fisher, K. Gillies and S. MacLennan, Cochrane Database Syst. Rev., 2014, 9, 1465–1858 Search PubMed
.
- C. P. McCoy, N. J. Irwin, C. Brady, D. S. Jones, L. Carson, G. P. Andrews and S. P. Gorman, Mol. Pharm., 2016, 13, 2817–2822 CrossRef CAS PubMed
.
-
(a) R. Bayston, W. Ashraf and C. Bhundia, J. Antimicrob. Chemother., 2004, 53, 778–782 CrossRef CAS PubMed
;
(b) R. Bayston, W. Ashraf and C. Ortori, J. Neurosurg., 2016, 124, 375–381 CAS
;
(c) R. Bayston, L. E. Fisher and K. Weber, Biomaterials, 2009, 30, 3167–3173 CrossRef CAS PubMed
;
(d) R. Bayston, G. Ullas and W. Ashraf, Antimicrob. Agents Chemother., 2012, 56, 2842–2845 CrossRef CAS PubMed
.
- L. E. Scriven, MRS Proc., 1988, 121, 717 CrossRef CAS
.
- R. Bayston, N. Grove, J. Siegel, D. Lawellin and S. Barsham, J. Neurol., Neurosurg. Psychiatry, 1989, 52, 605–609 CrossRef CAS PubMed
.
-
(a) M. Bassetti, E. Repetto, E. Righi, S. Boni, M. Diverio, M. P. Molinari, M. Mussap, S. Artioli, F. Ansaldi, P. Durando, G. Orengo, F. B. Pallavicini and C. Viscoli, J. Antimicrob. Chemother., 2008, 61, 417–420 CrossRef CAS PubMed
;
(b) D. J. Hong, J. O. Kim, H. Lee, E. J. Yoon, S. H. Jeong, D. Yong and K. Lee, Diagn. Microbiol. Infect. Dis., 2016, 86, 184–189 CrossRef CAS PubMed
.
- D. Kowalczuk, G. Ginalska and A. Przekora, J. Biomed. Mater. Res., Part A, 2011, 98, 222–228 CrossRef PubMed
.
-
(a)
J. Davison, J.-Y. Maillard, J.-M. Pagès, K. Pfaff and S. C. Rastogi, Opinion on triclosan: antimicrobial resistance, Scientific Committee on Consumer Safety, Directorate-General for Health and Consumers, European Commission, Brussels, 2010 Search PubMed
;
(b) Food-and-Drug-Administration, Fed. Regist., 2016, 81, 61106–61130 Search PubMed
.
- A. L. Hook and D. J. Scurr, Surf. Interface Anal., 2016, 48, 226–236 CrossRef CAS PubMed
.
- N. Jeffery, K. Kalenderski, J. Dubern, A. Lomiteng, M. Dragova, A. Frost, B. Macrae, A. Mundy, M. Alexander, P. Williams and D. Andrich, Eur. Urol., Suppl., 2019, 18, e377 CrossRef
.
- H. E. Gottlieb, V. Kotlyar and A. Nudelman, J. Org. Chem., 1997, 62, 7512–7515 CrossRef CAS PubMed
.
- R. Bayston and R. D. G. Milner, J. Clin. Pathol., 1981, 34, 1057–1062 CrossRef CAS PubMed
.
Footnote |
† Electronic supplementary information (ESI) available. See DOI: 10.1039/d0bm00709a |
|
This journal is © The Royal Society of Chemistry 2020 |
Click here to see how this site uses Cookies. View our privacy policy here.