DOI:
10.1039/C9BM01691K
(Paper)
Biomater. Sci., 2020,
8, 2143-2155
Cu, Zn doped borate bioactive glasses: antibacterial efficacy and dose-dependent in vitro modulation of murine dendritic cells
Received
22nd October 2019
, Accepted 5th March 2020
First published on 31st March 2020
Abstract
Among emerging biomaterials, bioactive glasses (BGs) are being widely explored for various applications in tissue engineering. However, the effects of BGs (in particular BG ionic dissolution products) on immune cells and specifically on dendritic cells (DCs), which are the most potent antigen-presenting cells of the immune system, have not been previously investigated in detail. Such interactions between BGs and DCs must be assessed as a novel biocompatibility criterion for biomaterials, since, with the increased application possibilities of BGs, the modulation of the immune system may induce potential complications and undesired side effects. Indeed, the effects of BG exposure on specific immune cells are not well understood. Thus, in this study we investigated, for the first time, the effect of borate BGs doped with biologically active ions on specific immune cells, such as DCs and we further investigated the antibacterial properties of these borate BGs. The compositions of the borate BGs (B3) were based on the well-known 13–93 (silicate) composition by replacing silica with boron trioxide and by adding copper (3 wt%) and/or zinc (1 wt%). By performing an agar diffusion test, the antibacterial effect depending on the compositions of the borate BGs could be proved. Furthermore we found a dose-dependent immune modulation of DCs after treatment with borate BGs, especially when the borate BGs contained Zn and/or Cu. Depending on the ion concentration and the rise in pH, the phenotype and function of DCs were modified. While at low doses B3 and Zn-doped B3 BGs had no impact on DC viability, Cu containing BGs strongly affected cell viability. Furthermore, the surface expression of DC-specific activation markers, such as the major histocompatibility complex (MHC)-II, CD86 and CD80, was modulated. In addition, also DC mediated T-cell proliferation was remarkably reduced when treated with high doses of B3–Cu and B3–Cu–Zn BGs. Interestingly, the release of inflammatory cytokines increased after incubation with B3 and B3–Zn BGs compared to mock-treated DCs. Considering the essential role of DCs in the modulation and regulation of immune responses, these findings provide first evidence of phenotypic and functional consequences regarding the exposure of DCs to BGs in vitro.
1. Introduction
In the last 50 years, a significant amount of research efforts has been focused on the development of new biomaterials, which can be used to regenerate, repair and replace living tissues and their functions.1 An early example of a biomaterial that has the ability to bond to human tissues is 45S5 bioactive glass (BG), which was originally developed by Larry L. Hench for bone replacement applications.2 This original 45S5 BG composition (in wt%: 45.0 SiO2, 24.5 CaO, 24.5 Na2O and 6.0 P2O5) is based on silica as a glass network former, whereas nowadays also boron trioxide and phosphorus pentoxide are used as basic units for novel compositions of BGs.3 Borate BGs are receiving increasing interest for their notable biological performance, e.g. in contact with both bone and soft tissues.4–6 All types of BGs are found to be “bioactive” based on two mechanisms: (i) the formation of an apatite layer on the surface during the dissolution of the BG in a physiological environment and (ii) the release of biologically active ions. The first “bioactivity” mechanism is well described for the 45S5 BG composition.7 Briefly, the interaction of BG surfaces with body fluids starts with an ion exchange which leads to an increase of the pH of the medium, followed by the formation of a silica-rich layer and then by the growth of a calcium-phosphate rich layer on the BG surface. This layer then crystalizes to hydroxycarbonate apatite, which finally promotes bonding with bone.7 “Bioactivity” strongly depends on the glass composition and therefore on the glass reactivity. In general, borate BGs degrade faster than silicate BGs in contact with aqueous media, which is expected due to their relatively low chemical durability.8,9 This faster degradation rate and additionally the antiseptic properties of boric acid make borate BGs interesting for wound healing applications.10 In this study, (SiO2-free) borate BGs containing additionally magnesium and potassium were investigated.11
As mentioned above, the second mechanism behind the bioactivity of BGs is the release of biologically active ions.12 Depending on the composition, different therapeutic active ions such as B, Si, Ca, P, etc. are released into the human body during the implantation of BGs. These ions have important effects on cells. For instance, Ca and Si favor osteoblast differentiation, whereas B is known to stimulate fibroblast cells and angiogenesis.12 Increasing research efforts currently focus on doping BGs with such biological ions, which provide a specific, dose-dependent therapeutic effect. Typical examples are zinc and silver, both showing antibacterial effects, and copper and cobalt, which exhibit angiogenic properties.12–14 These ions can be generally incorporated into the glass structure in small amounts, since at higher concentrations they can have a cytotoxic effect. In the case of using copper, which is known to promote angiogenesis by mimicking hypoxia, therefore leading to the upregulation of the expression of the vascular endothelial growth factor,15 a cytotoxicity level at concentrations above 10 mg L−1 has been found.16 The present study has focused on the systematic evaluation of the dissolution behavior of borate BGs doped with copper and/or zinc and the investigation of their effect on dendritic cells. The antibacterial effects of such BGs have also been investigated.
Dendritic cells (DCs) are specialized immune cells, which play a crucial role in the initiation of primary immune responses and are capable of linking innate and adaptive immune responses.17 DCs are considered to be the most potent antigen-presenting cells, governing both T cell immunity and tolerance.18 Due to their ability to take up, process and present antigen, and their localization in peripheral lymphatic tissues, DCs are able to induce an antigen-dependent activation of naive T cells. DCs play distinct roles in shaping T cell development, differentiation and function.19 The outcome of the DC–T cell interaction is determined by the state of DC maturation, the type of DC subset, the cytokine microenvironment and the tissue location. DC maturation, which is associated with phenotypical and functional changes, is crucial for their strong immune stimulatory potential. In their immature state, DCs reside in peripheral tissues. Upon antigen uptake and in the presence of inflammatory and/or microbial stimuli, they start to mature and migrate to the T cell areas of the peripheral lymph nodes and potently stimulate T cells.20,21 Mature DCs are absolutely crucial in order to induce potent immune responses, while immature/semi-mature DCs have been reported to be involved in tolerogenic mechanisms.22 The phenotype of mature DCs is characterized by the up-regulation of co-stimulatory molecules. In addition, signals delivered via co-stimulatory molecules such as CD80 and CD86 are essential.23 The stimulation of the CD28 receptor via CD80 and CD86 leads to T cell activation, while the engagement of CTLA-4 (CD152), CD80 and CD86 results in the attenuation of T cell responses.24 The integration of signals through this family of co-stimulatory and inhibitory receptors and their ligands is critical for the activation of immune responses and tolerance. Nothing is known about the effect of BGs on DC functions. Accordingly, the present study has been carried out to examine different borate BGs, which were previously developed and completely characterized,25 in terms of their modulation capability of murine DCs. Specifically, the impact of the ionic dissolution products (IDPs) of different BGs on the phenotype and function of murine DCs and the possible toxic effects of such IDPs were evaluated for the first time. For instance, Zn was shown to influence the tolerogenic potential of DCs in vitro and in vivo.26 As discussed in a recently published review paper,27 the present study is relevant for BG applications because the immune system is involved in any regeneration process in humans and therefore the influence of BGs on the immune system needs to be considered. In addition, the BG compositions were investigated for their antibacterial effects since the immune system plays an important role in bacterial infections.
2. Materials and methods
2.1. Glass and cell culture media preparation
Different borate BGs doped with Cu and/or Zn (compositions are given in Table 1) were prepared (at Åbo Akademi, Finland) as described previously.25 Briefly, analytical grade reagents were melted in a platinum crucible at 1050 °C for 2 h, then casted, annealed at 520 °C and crushed. The glasses were melted twice to ensure homogeneity. Then, the glasses were crushed and sieved to obtain a particle size in the range of 300–500 μm (according to scanning electron micrographs of the produced BG particles25). Based on microscopy observations25 and the fact that the fabrication methods, including crushing and sieving, were the same for all glasses, the specific surface area values of the different BGs used in this study were regarded as comparable. The glass powders were heat sterilized at 180 °C for 2 h.
Table 1 Composition of the investigated bioactive glasses in wt%
Name |
Na2O |
K2O |
MgO |
CaO |
B2O3 |
P2O5 |
ZnO |
CuO |
B3 |
5.5 |
11.1 |
4.6 |
18.5 |
56.6 |
3.7 |
— |
— |
B3–Cu |
5.5 |
11.1 |
4.6 |
15.5 |
56.6 |
3.7 |
— |
3.0 |
B3–Zn |
5.5 |
11.1 |
4.6 |
17.5 |
56.6 |
3.7 |
1.0 |
— |
B3–Cu–Zn |
5.5 |
11.1 |
4.6 |
14.5 |
56.6 |
3.7 |
1.0 |
3.0 |
To produce the conditioned media containing IDPs from different bioactive glasses, 1 g of bioactive glass was added to 10 ml of cell culture medium (CCM) to form a 10% suspension and media were incubated for 24 h at 37 °C under a humidified atmosphere of 95% air and 5% CO2. The used CCM was composed of RPMI 1640 (Lonza, Veviers, Belgium) supplemented with penicillin (100 U ml−1, Sigma), streptomycin (100 mg ml−1, Sigma), L-glutamine (2 mM, Sigma), 2-mercaptoethanol (50 mM, C. Roth) and 10% heat-inactivated fetal calf serum (FCS, Merck). During incubation, the pH of the suspension was measured after 3 h, 6 h, 12 h and 24 h. The remaining glass particles in the CCM were then removed and this solution is named 10% CCM. In order to evaluate the pH increase for 1% and 0.1% dilution, 10 ml of CCM containing either 0.1 g or 0.01 g of different bioactive glasses was prepared and the pH value was measured after 3 h, 6 h, 12 h and 24 h of dissolution time. The concentration of IDPs in the 10% dilution was measured by inductively coupled plasma atomic emission spectroscopy (ICP-OES, Thermo Scientific iCAP 6500) using Ar as plasma (flow rate: 10 L min−1) and nebulizer gas (0.6 L min−1). The auxiliary gas (0.2 L min−1) was N2. The aqueous calibration and sample solutions were fed to the nebulizer at a flow rate of 3 mL min−1. The plasma power was 1500 W and a 6-point calibration was performed for the elements Na, K, Mg, Ca, P, Cu and Zn in the concentration range between 1 mg L−1 and 100 g L−1. The emission lines at 589.592 nm (Na), 766.49 nm (K), 285.213 nm (Mg), 317.933 nm (Ca), 214.914 nm (P), 327.393 nm (Cu) and 206.200 nm (Zn) were evaluated for quantification. The calibration solutions were obtained by diluting the respective 1000 mg L−1 standard solutions specified for ICP-OES (Carl Roth, Germany). All samples were measured in triplicates. The 10%-CCM was then further diluted to prepare 1% and 0.1% dilution of IDPs in CCM for in vitro cell culture tests. As control, CCM without IDPs (mock) and CCM containing 10 mM 4-(2-hydroxyethyl)piperazine-1-ethanesulfonic acid, N-(2-hydroxyethyl)piperazine-N′-(2-ethanesulfonic acid) (mock buffered ) were used.
2.2. Antibacterial analyses
Antibacterial agar diffusion tests were carried out against Staphylococcus aureus (Gram-positive) and Escherichia coli (Gram-negative) bacteria (obtained from the Microbiology Department of the University of Erlangen-Nuremberg). Bacteria were prepared and expanded according to a previously developed protocol.28 Briefly, bacteria were suspended in Luria broth medium until an optical density of 0.015 was reached. Then, 20 μL of this medium containing bacteria was deposited and spread homogeneously on a Petri dish (diameter 10 cm) containing a uniform layer of Luria broth agar. Pellets containing milled bioactive glass powders (particle size <10 μm) were then placed on the agar and incubated for 24 h at 37 °C. BG pellets were made from the powders using an hydraulic press (PE-010, Mauthe Maschinenbau) with a load of 1 ton (no additives were used). All samples were analyzed in triplicates for Gram-positive and Gram-negative bacteria. After 24 h, the inhibition zones around the samples were assessed using ImageJ (calculated from 10 measurements).
2.3. Mice
C57BL/6 and BALB/C mice were purchased from Charles River/Wiga (Sulzfeld, Germany) or bred within our own facilities and maintained under pathogen-free conditions according to the institutional and national guidelines for the care and use of laboratory animals. The studies were approved by the animal ethical committee of the Government of Unterfranken, Würzburg.
2.4.
In vitro preparation of bone marrow-derived DCs
Bone marrow derived DCs from mice were generated as described previously.29 In brief: bone marrow precursor cells were flushed from the femurs and tibias of C57BL/6 mice and cultured for 6 days in 10 ml of CCM medium at a density of 2 × 106 cells per 10 cm dish (Falcon, no. 1029, bacterial quality, Heidelberg, Germany). GM-CSF supernatant (1
:
10) from a cell line transfected with the murine GM-CSF gene was used as described.30 On day 3, a volume of 10 ml of CCM medium containing 1 ml of GM-CSF supernatant was added to the cultures. 50% of the culture supernatant was removed at day 6 and the cells were fed again with fresh 10 ml of 1
:
10 diluted CCM-medium containing GM-CSF supernatant. On day 7 immature DCs were harvested and used for different experiments.
2.5. DC maturation and treatment with BGs
Immature DCs were cultured at a density of 1 × 106 cells per ml in a 24 well plate together with increasing concentrations of CCM containing IDPs from BGs. Cultures were also left untreated (mock) or in buffered CCM (mock buffered; pH 8.5 adjusted with NaOH) for 36 h. After this time period LPS (Escherichia coli 0127:B8, 100 ng ml−1, Sigma) was added to the different cell cultures and left for an additional 12 h. After a total cultivation time of 48 h mature DCs were harvested and used for further phenotypical and functional analyses.
2.6. Phenotypic characterization of murine DCs by flow cytometry
To determine the effect of the BG ionic dissolution products on DC modulation, the above mentioned DCs co-incubated with varying amounts of CCM containing IDPs from B3, B3–Cu, B3–Zn, and B3–Cu–Zn BGs were analyzed for the expression of DC specific surface markers using flow cytometry. The mature surface DC phenotype was analyzed using antibodies specific for CD11c (clone N418, BioLegend), MHCII (clone M5/114.15.2, BioLegend), CD86 (clone GL-1, BD Biosciences), CD80 (clone 16-10A1, BioLegend) and CD83 (clone Michel-19, BioLegend). Samples were stained using 7-AAD (7-aminoactinomycin D) to determine the toxicity of the substances and to perform the analysis of living cells (ThermoFisher Scientific). Samples were measured using the BD FACS Canto II cytometer and were analyzed using FCS Express 5 Flow Cytometry Software (DeNovo software).
2.7. Mixed leukocyte reaction (MLR)
In order to analyze if the above described pretreated DCs have an impact on DC-mediated allogeneic T-cell stimulation, functional MLR assays were performed. Mature murine DCs, which were generated as described in section 2.5, were harvested, washed with PBS and counted. Afterwards the isolated DCs were co-cultured at different ratios with allogeneic murine splenic cells from BALB/c mice for 72 h in 200 μl of CCM in 96-well cell culture plates, without any further addition of CCM containing IDPs. Finally the co-cultures were pulsed with 3H-thymidine (1 μC per well) (PerkinElmer) for 16 h to determine T-cell proliferation. Culture supernatants were then harvested onto glass fibre filters (PerkinElmer) using an ICH-110 harvester (Inotech) and the filters were counted in a 1450 microplate reader (Wallac).
2.8. Cytometric bead array (CBA)
Supernatants of murine DC-T cell co-cultures were harvested after 72 h and the quantities of interleukin IL-6, TNF-α, IL-1α, GM-CSF and interferon-γ (IFN-γ) were determined using the LEGENDplex™ Murine Inflammation Panel (BioLegend) according to the manufacturer's protocol.
2.9. Statistical analyses
If not stated otherwise, results are displayed as mean ± standard error of the mean (SEM). For multiple comparisons, data were analyzed using two-way ANOVA or one-way ANOVA and the Bonferroni or Dunnett multiple comparison post hoc test. Significant differences were assumed if p was <0.05.
3. Results
3.1. Characterization of conditioned cell culture media
The used borate BGs were completely characterized in a previously published study, showing that the fabricated glasses were successfully doped with Cu and/or Zn.25 As previously reported,25 all fabricated BGs exhibited amorphous structure and FTIR measurements confirmed their borate glass network. Moreover, no pronounced effect of adding copper or zinc on the thermal properties of the borate BGs could be observed.25 As shown in the previous work, during the dissolution of different borate BGs, ions were released into the medium, which led to a pH increase. In Fig. 1, the pH increase as a function of time can be seen for the different prepared conditioned media. During dissolution, all four types of BGs reached the highest pH value for the 10% dilution, and after 24 h the pH value was 8.7 ± 0.1. As expected, for the 0.1% dilution and 1% dilution the BGs reached lower pH values, of 8.2 ± 0.1 and 8.5 ± 0.1, respectively, after 24 h.
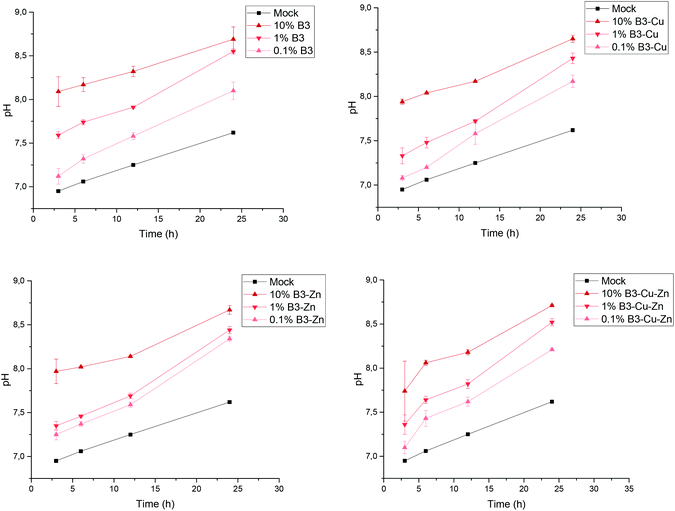 |
| Fig. 1 Change in pH during the preparation of conditioned cell culture media. Time-dependent increase of pH is observed in BG conditioned cell culture media which is stabilized at 8.7 ± 0.1 after 24 h. This observation is similar for the different borate-based BGs. All samples were measured in triplicates. | |
The conditioned 10%-CCM was further analyzed by ICP-OES in order to determine the concentration of the different ions released by the BGs (Fig. 2). Based on the amount of B released, the addition of Cu and Zn seems not to have any influence on the network stability of the BGs, and consequently on the ion release profile, which could be observed also in the previously published study25. Moreover, according to the ICP-OES measurements, the amount of P decreased in the conditioned media compared to the reference. This result indicates that both P and Ca (proven by the small amount of Ca released) are involved in the precipitation of amorphous CaP-rich species. Most interestingly, Cu could be released from the Cu-doped and Cu–Zn-doped BGs, with the concentration of (61.8 ± 3.7) mg L−1 and (54.8 ± 1.0) mg L−1, respectively. In contrast, Zn was not released or released at a very low concentration (below the detection limit of the instrument), which is in accordance with the results obtained in our previous study on borate BGs.25
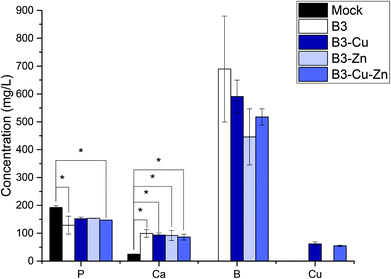 |
| Fig. 2 Ion concentration of 10%-CCM containing IDPs of the different glasses measured by ICP-OES. No remarkable difference between the fabricated borate-based BGs could be found (besides the release of copper from Cu and Cu–Zn doped BGs), proving that the addition of Cu and/or Zn did not significantly influence the glass network. The release of Zn could not be detected. All samples were measured in triplicates. One-way ANOVA statistical analysis denotes significant differences compared with the reference (*p < 0.05). | |
3.2. The antibacterial effect of BGs
The results of the agar-disk diffusion tests are shown in Fig. 3. Data showed an inhibition zone greater than 1 mm, which, in accordance with the Standard SNV 195920-1992, can be considered antibacterial.28 Therefore, all BG pellets which were in contact with Gram-positive and Gram-negative bacteria showed antibacterial effect to some extent. A clear difference was observed when copper and/or zinc was added: copper had the greatest effect, while Zn-doped BG was found to have only a minor antibacterial effect improvement, compared to the undoped BGs. This result is in accordance with the ion release measurements (section 3.1) showing that zinc might not be or be only slightly released. This behavior could also be observed in BG pellets doped with zinc and copper, where the inhibition zone was found to be intermediate between Zn-doped and Cu-doped BGs. Furthermore, a significant different effect was observed between Gram-positive and Gram-negative bacteria. This is consistent with previous literature reports31 and might be due to the higher susceptibility of Gram-positive bacteria, related to differences in metabolism, cell wall composition, cell physiology or cell structure, than Gram-negative bacteria. Due to their highly organized compact structure, Gram-negative bacteria are “more protected” against diffusion and penetration of antibacterial ions.32
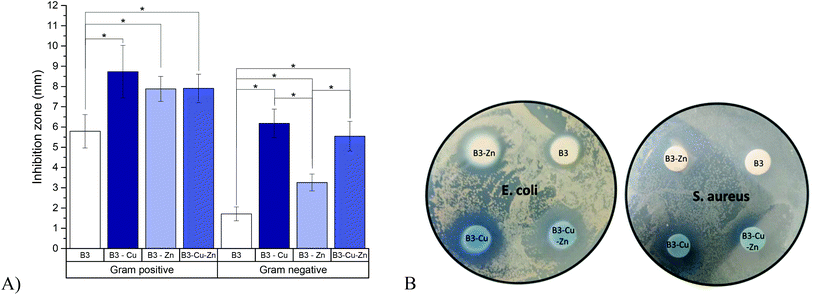 |
| Fig. 3 Antibacterial effects of BGs against Gram-positive (S. aureus) and Gram-negative (E. coli) bacteria, 24 h after incubation. (A) Summary of mean inhibition zones of different BG pellets assessed using an agar-disk diffusion assay; (B) example of a well-plate assay showing the inhibition of E. coli and S. aureus bacteria growth, by different BGs. All samples were measured in triplicates. One-way ANOVA statistical analysis denotes significant differences (*p < 0.05). | |
3.3. Cell viability
BGs are being used in several biomedical applications and are being increasingly considered for bone and soft tissue replacement thanks to their ability to form strong bonding with tissues and, in some cases, to stimulate tissue regeneration.1,5,33,34 Thus, BGs should show no or very low toxicity and have only limited side effects. The immune system of the host, and thus a functional and competent immune system, is important for maintaining control of an unwanted immune response. However, due to their high reactivity once in contact with water-based solutions BGs rapidly exchange ions with the surrounding environment which can lead to an undesired increase in the pH value under static in vitro conditions making it difficult or even impossible to perform cell culture studies.35 Therefore it is very important to analyze/identify possible immune-modulating properties of these BG compounds to avoid an unwanted immune response or a decreased function of the immune system of the host. Such an effect may result in an increased susceptibility towards other infections, e.g. bacterial or fungal, particularly in patients with an already compromised immune system. DCs are known to be the most potent antigen-presenting cells and thus are key players during the induction of an effective and potent antiviral immune response. Efficient maturation of these cells is indispensable to gain their migratory and T cell stimulatory capacity.20 Considering these facts, we examined individual BG compounds regarding their toxicity and their impact on specific immune cells. Toxicity was determined by flow cytometry using a standard cell viability assay (Fig. 4). To address this demand, we generated DCs, which was achieved by flushing bone marrow from the tibia of mice and culturing in medium supplemented with BGs, as described in section 2.5. Immature DCs were stimulated for 48 h in the presence of B3, B3–Cu, B3–Zn and B3–Cu–Zn and then matured with LPS overnight. The solutions of 0.1% and 1% B3 glass showed no significant toxic effects when compared with the mock and mock buffered control. In contrast, 10% B3 solutions showed toxic effects (Fig. 4A). As shown in Fig. 4B, 1% B3–Cu and 10% B3–Cu samples showed a significant increase in cell toxicity compared to mock controls, which is likely due to the toxic effects of Cu concentration. However, the concentration of 0.1% B3–Cu solution had no impact on the cell viability. As B3 solution alone, only 10% B3–Zn solution led to a significant increase in cell toxicity (Fig. 4C) compared to mock controls. As shown in Fig. 4D both 1% and 10% B3–Cu–Zn solutions showed toxic effects on DCs compared with untreated controls. Since in the present context pH values were not expected to cause toxicity, these effects can be explained by the already mentioned high Cu concentrations.
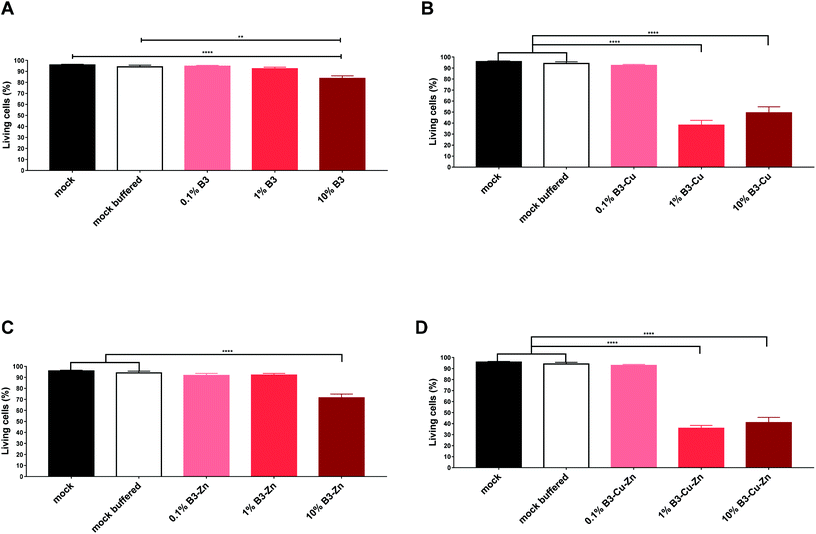 |
| Fig. 4 BGs modulate DC viability. Bone marrow was flushed from the femur and tibia of C57BL/6 mice and cultured in GM-CSF supplemented media for 8 days. Immature DCs were cultivated in the presence of the indicated concentrations of (A) B3, (B) B3–Cu, (C) B3–Zn, and (D) B3–Cu–Zn BGs and then matured with LPS. After 48 hours, cells were harvested and the percentage of living cells was determined. Mean ± SEM of individual experiments is shown. The experiment was conducted up to six times with cells derived from different donors. Significant changes are marked with asterisks (*: p < 0.05, **: p < 0.01, ***: p < 0.001). | |
3.4. BGs interfere with the expression of functionally important activation molecules on DCs
Next, we investigated the impact of BGs for adverse immune-modulatory effects on DCs. To do so, immature DCs were cultivated in the presence of B3, B3–Cu, B3–Zn and B3–Cu–Zn BGs for 48 hours, as mentioned above. Mock and mock buffered cells served as negative control. The effect of BGs on activation/phenotypic maturation of the murine DCs was assessed by measuring the expression of the cell surface markers CD11c, MHC-II, CD80, CD83, CD86 and CD25 (= IL-2R), as indicators of the maturation status of the generated matured DCs (Fig. 5). Following isolation, cell surface characterization was performed using flow cytometry by gating on the CD11c population. As shown in Fig. 5A, 48 h of B3 BG treatment had a significant dose-dependent impact on the expression of the markers CD11c, MHCII and CD86 under the 1% conditions as compared to untreated (mock) DCs or mock buffered DCs. The expression of the surface markers CD83 and CD25 was unaffected. Interestingly, treatment with B3–Cu BG led to a dose-dependent reduction of the expression of all surface markers, especially MHCII, CD80, CD86 and CD25 were significantly reduced (Fig. 5B). The addition of B3–Zn BG to DC cultures showed a similar effect to B3 BG alone. The expression of CD11c, CD80 and CD86 cells was significantly increased depending on the concentration (Fig. 5C). After 48 h of B3–Cu–Zn BG treatment, the expression of MHCII, CD80, CD86 and CD25 was significantly decreased as compared to the control. Furthermore the expression of CD83 was affected, but not significantly. These data suggest a phenotypic DC maturation or at least activation of the cells after incubation with B3 and B3–Zn BGs. The maturation of DCs in the presence of Cu containing BGs, like 10% B3–Cu and 10% B3–Cu–Zn, led to a reduced DC activation and maturation.
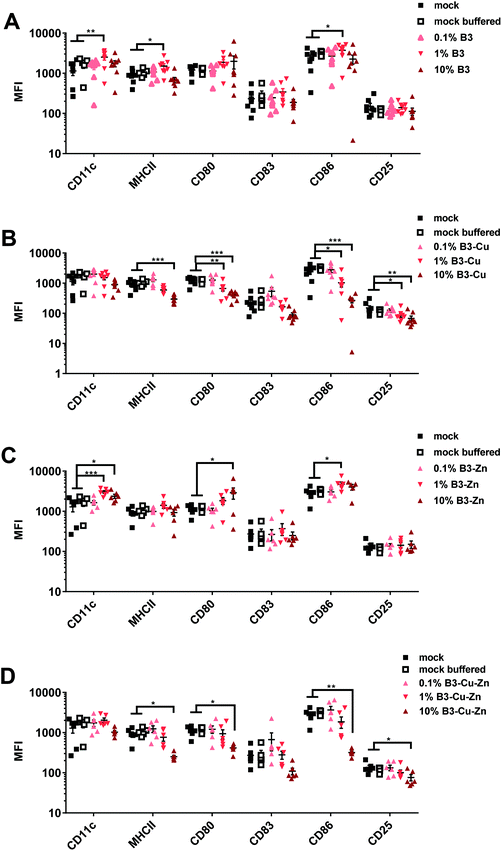 |
| Fig. 5 BGs modulate the phenotype of mature murine DCs. Immature DCs were cultivated in the presence of the indicated concentrations of CCM with BGs. After maturation with LPS, cells were harvested and the MFI of CD11c+, MHCII+, CD80+, CD83+ and CD80+ and CD25+ on DCs, which were treated with (A) B3, (B) B3–Cu, (C) B3–Zn, and (D) B3–Cu–Zn BGs, was determined. Mean ± SEM of individual experiments is shown. The experiment was conducted up to six to eight times with cells derived from different donors. Significant changes are marked with asterisks (*: p < 0.05, **: p < 0.01, ***: p < 0.001). | |
3.5. DC-mediated allogeneic T-cell proliferation is influenced by BGs in a dose-dependent manner
Considering that BGs altered the immunogenic phenotype of DCs, we further analyzed the effects of treatment with BG dissolution products on DCs through in vitro mixed leucocyte reactions. This is a relatively simple way of assessing the DC-mediated allogeneic T cell proliferation capacity and the functionality of DCs. For this reason, DCs were treated with different BGs as described above and co-cultured with allogeneic lymphocytes. The immunogenic potential or the ability of DCs to induce the proliferation and/or the activation of allogeneic lymphocytes was analyzed using T-cell proliferation assays. In this study, immature DCs were cultivated in the presence of BGs with different concentrations for 48 h, matured with LPS and they were then incubated with different ratios of allogeneic murine lymphocytes. T cell proliferation was assessed 72 h after incubation. Mock and mock buffered treated samples were used as controls (Fig. 6). While both 10% and 1% B3 and B3–Zn BG conditions led to a significant increase in DC mediated T cell proliferation (Fig. 6A and C), 10% B3–Cu and 10% B3–Cu–Zn BGs inhibited T cell proliferation almost completely (Fig. 6B and D). On the other hand, 1% B3–Cu and 1% B3–Cu–Zn BGs increased the potential of DCs to stimulate alloreactive T cells. Thus, the interference of BGs with full DC maturation (B3 and B3–Zn) or reduced DC maturation (B3–Cu and B3–Cu–Zn), as described above, has also functional consequences, i.e. a reduced capacity to stimulate T cells.
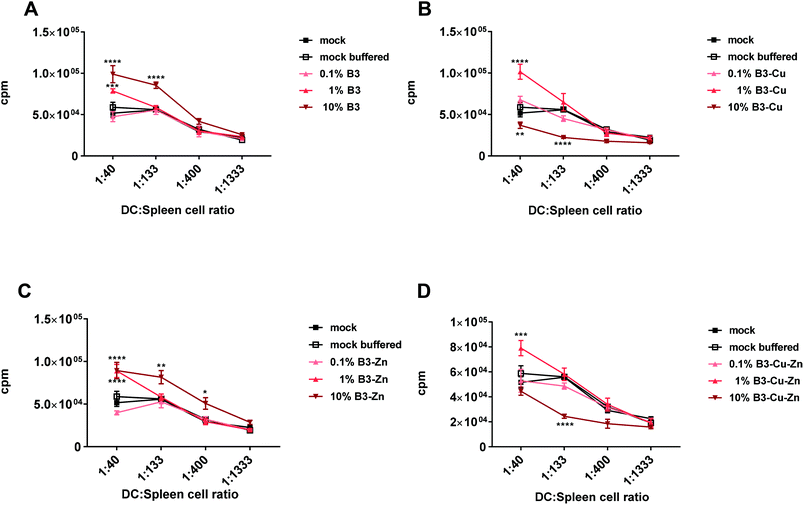 |
| Fig. 6 Treatment of DC with BGs modulates their ability to stimulate allogeneic splenic cells. Murine DCs were cultivated for 48 hours in the presence of both mock control (black square) and buffered medium (open square), or the indicated concentrations of (A) B3, (B) B3–Cu, (C) B3–Zn and (D) B3–Cu–Zn BGs, and consequently matured with LPS. Afterwards cells were co-cultured with allogeneic murine splenic cells for 72 hours and cell proliferation was assessed using Thymidin incorporation. Mean ± SEM of three biological triplicates is shown. One representative experiment out of four individual experiments is shown. Significant changes are marked with asterisks (*: p < 0.05, **: p < 0.01, ***: p < 0.001, ****: p < 0.0001). | |
3.6. The effects of different BGs on pro-inflammatory cytokine formation
Furthermore, we also analyzed the secretion of pro-inflammatory cytokines from the supernatants of the previously described DC-T cell co-cultures. Thus, titrated numbers of pre-conditioned B3, B3–Cu, B3–Zn or B3–Cu–Zn BG DCs were incubated with allogeneic T cells for 72 h and the cytokine concentrations in the supernatants were determined using a murine CBA kit (Fig. 7). Interestingly, DCs cultivated in B3 and B3–Zn BG conditioned medium led to an increased production of TNF-α, GM-CSF, IL-17A and IL-6 in a concentration-dependent manner, and significance was reached when DCs were pre-treated with 10% media of both B3 and B3–Zn BGs (see Fig. 7A). Thus, the dose-dependent increase of DC-mediated T cell proliferation correlates with an increased cytokine expression profile. Interestingly in DC-T cell co-cultures, where DCs were cultivated in 0.1% and 1% B3–Cu and B3–Cu–Zn doped media, increased levels of TNF-α, IL-1α, IL-17A and IL-6 were measured, whereas the co-cultures with DCs pre-treated with 10% B3–Cu and B3–Cu-Zn BGs showed clearly reduced cytokine levels. The reduced DC-mediated T cell proliferation correlates with a repressed cytokine expression profile.
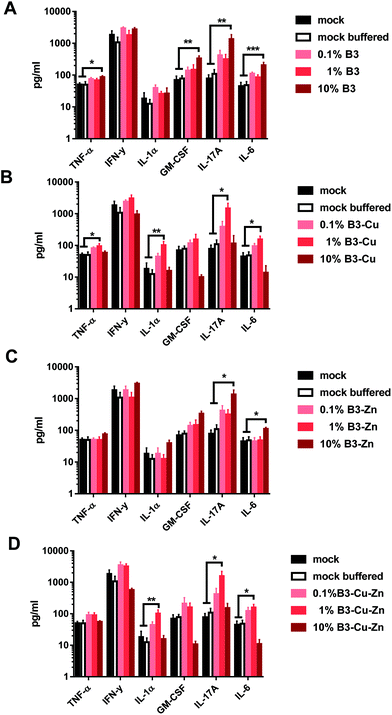 |
| Fig. 7 BG treatment leads to altered cytokine production by murine DC–T cell co-cultures. The production of TNF-α, IFN-y, IL-1α, GM-CSF, IL-17A and IL-6 during murine DC–T cell co-cultures was determined from the culture supernatants. Therefore DCs which were pre-incubated with different BGs for 48 h were stimulated with LPS and co-cultured with allogeneic T cells (ratio 1 : 40) for 72 h. The supernatants were assayed using a CBA kit. Mean ± SEM of four individual experiments is shown. Significant changes are marked with asterisks (*: p < 0.05, **: p < 0.01). | |
4. Discussion
4.1. The ion release effects of BGs
BGs have the capability to release biologically active ions and have the ability to form hydroxyapatite layers on their surface during dissolution in aqueous environments. However, this bioactive mechanism can also have a negative impact, since the dissolution of BGs leads to an increase in the pH value and osmotic pressure of the surrounding medium, which on one hand generates a hostile environment for bacterial growth and on the other hand can induce cytotoxic effects on immune cells after implantation in the human body. Furthermore, the ions released from BGs can have cytotoxic effects when exceeding a critical concentration level, thereby influencing gene expression.35 Therefore, in the present study both the pH increase and the concentration of ions released from the fabricated borate BGs doped with copper and/or zinc were evaluated. As expected, depending on the concentration of BGs, an increase in the pH value of the CCM was observed (Fig. 1). However, the addition of Cu and/or Zn to the borate BGs investigated here did not significantly influence the increase of pH, which is in accordance with the concentration of ions released from the different fabricated BGs and the obtained results in the previously published study.25
4.2. The antibacterial efficacy of borate BGs doped with copper and zinc
The antibacterial properties of BGs can be explained by a change in pH and by the effect of the osmotic pressure. The shift in the pH value to a more alkaline range induces a hostile environment for bacteria, which leads e.g. to changes in their morphology.36 Furthermore, changes in the ion concentration in the bacterial surrounding lead to decreased pressure across the bacterial cell membrane, which induces bacterial shrinkage and damage to the bacterial cell membrane.36 Additionally, BGs offer the advantage, compared to antibiotics, that bacteria are unlikely to develop resistant mechanisms against their antibacterial properties, since even after repeated exposure, minimal concentrations of BGs do not change the antibacterial efficacy.37 Using agar diffusion tests we demonstrated in this study an efficient antibacterial effect of the used borate BGs on both Gram-positive and Gram-negative bacteria (Fig. 3). Since the effect of B3 BG on Gram-negative bacteria was rather low, the biocide metal ions copper and/or zinc were additionally introduced into the B3 BG composition. Interestingly, although zinc could not be released during dissolution in CCM, Zn-doped BG showed a significant improvement of the antibacterial effect (Fig. 3). Zinc ions can act as either bactericidal or bacteriostatic. Whereas ZnO directly leads to the disruption of the bacterial cell wall, Zn2+ can inhibit the glycolytic processes of bacteria.38–40 The dissolution of BGs and the release of ions (especially in the case of zinc) are strongly dependent on the surrounding pH value. During the growth of bacteria, an acidic environment during the agar diffusion test can occur and may lead to the release of zinc ions, resulting in the antibacterial properties of Zn-doped BGs. Moreover, also the presence of zinc on the surface of the tested BG pellet (similar to the antibacterial effect of ZnO particles) may affect the bacterial growth. On the other hand, the antibacterial effect of copper, also in the form of released ions from Cu-doped BGs, has been well studied.28 Similar to the effects of zinc, the bacterial membrane integrity is disrupted by the oxidative damage of membranous phospholipids. Furthermore, copper ions can penetrate into bacteria, leading to oxidative stress and DNA degradation.41 A clear antibacterial effect of copper in Cu-doped and Cu–Zn-doped BGs was detectable (Fig. 3), which correlates with the released copper ions from the fabricated BGs during dissolution (Fig. 2). However, by combining zinc and copper, no further improvement of the antibacterial effect, compared to the Cu only doped BG, was observed. This result is most probably due to the relatively minor antibacterial effect of zinc ions compared to copper ions. In summary, we hypothesize that the antibacterial effects of the ion-doped BGs are mechanistically related to the release of copper and/or zinc ions, since the measured pH changes were not significantly different between the different BGs. However, further studies involving the examination of the morphological features of the bacterial cell damage need to be performed in order to better understand the effects of released copper and/or zinc ions and to elucidate the specific antibacterial mechanisms involved.
4.3. Dose-dependent immune-modulating effects of borate BGs doped with copper and zinc on toxicity and the phenotype of mature murine DCs
Next we investigated the functional properties of BGs on DCs. Since BG research has focused mainly on the application of BGs in the field of bone tissue engineering, most conducted in vitro studies have examined the effects of different BGs in contact with cells involved in bone regeneration processes.42–44 Moreover, borate based BGs have been found to be of special interest regarding the regeneration of soft tissue, and in the last two decades several studies have shown that BGs have great potential in the field of wound healing45–48 and neural tissue engineering.49 Additionally, the effects of copper and/or zinc doped borate BGs have been evaluated in in vitro studies using fibroblasts, osteoblasts and endothelial cells considering similar BG compositions to those used in the present study.40,45,46,48,50–55 Although these published results are promising, they also showed that borate BGs, especially when doped with copper, could induce cytotoxic effects, particularly at high doses.48,50
BGs have been extensively evaluated both in vitro and in vivo in their ability to improve bone and soft tissue regeneration, and so far several FDA approved BG compositions are commercially available in different forms (e.g. granules for bone regeneration and fibers for wound healing applications).6,7,56 However the influence of BGs on the immune system has not been investigated substantially. Given that the immune system is strongly involved in the processes of wound healing and bone regeneration, the specific effects of BGs on the immune response during soft tissue and bone regeneration processes need to be examined. Besides recognizing and combatting pathogens possibly occurring in wound beds, the immune system is also vitally involved in wound excision and in the healing process.57 In this respect, DCs play a crucial role during the induction of an effective and potent immune response and were therefore used (surprisingly for the first time in this study) to evaluate the influence of different BG compositions on this specific cell phenotype. Immature DCs are located in almost every organ and monitor the environment, e.g. for the presence of pathogens and in the case of an infection DCs mature and induce an antigen-specific activation of naive T cells.17,18,58 Furthermore it was shown that DCs infiltrate and interact with implanted biomaterials.59–61 In order to mimic this process in vitro and to analyze the effects of BGs on the behavior of DCs, immature DCs were matured in the presence of IDPs derived from different BGs. At low concentrations, borate B3 BG did not show any cytotoxic effect on matured DCs,42,44,48,49 but, as previously reported in the literature for other cell types, 10% B3 BG showed toxic effects also on mature DCs. However, it has been shown that by using dynamic instead of static conditions, the presence of 10% BG does not negatively influence cell viability.44 According to ICP-OES measurements, no significant differences were observed between B3 BG and B3–Zn BG (Fig. 1 and 2). However, the addition of copper showed a significant effect on the viability of DCs. In the case of B3–Cu and B3–Cu–Zn BGs, the viability of cells was significantly decreased under both the 1% and 10% conditions. According to ICP-OES measurements, the 10% B3–Cu BG solution contained (61.75 ± 7.15) mg l−1 Cu ions and the 10% B3–Cu–Zn BG solution contained (54.77 ± 2.76) mg l−1 Cu ions. We conclude from these results that there is a critical biological level of Cu2+ below 5 mg l−1 for the survival and growth of DCs. Cu2+ ions can be released in a controlled manner and the released concentration of Cu ions can be tuned with the substitution ratio of Cu in BGs. In a previous study, using fibroblasts, the critical biological level of Cu2+ was 10 mg l−1.16 Since these data indicate that DCs are more sensitive to copper ions, more investigations are needed and should include also other immune cells (e.g. B cells and T cells) to further study the impact of metallic ions on immunogenic responses.
Next, the maturation of DCs in the presence of BGs was evaluated. When DCs capture antigens during infection, they start to upregulate the expression of specific cell surface molecules like MHCII and CD86 and via the interaction of these molecules with T-cells, DCs induce an antigen-dependent T-cell activation.58 Flow cytometric analysis revealed that CD11c, which is a surface marker for myeloid DCs, was highly expressed by all DCs treated with different investigated BG formulations, confirming that DCs mature in the presence of BGs. For the initiation of an adaptive immune response, DCs present antigenic peptides in association with major histocompatibility complex class II (MHCII) molecules to naive CD4+ T lymphocytes.62 It is worth noting that only terminally differentiated mature DCs can efficiently induce immunity, whereas immature DCs rather promote immune tolerance.63 The treatment of DCs with B3 and B3–Zn BGs showed similar MHC-II expression levels under all treatment conditions, when compared to mock controls (Fig. 5). However, high concentrations of B3–Cu and B3–Cu–Zn BGs led to reduced MHC-II expression levels. In the case of B3 (10%) and B3–Zn (1% and 10%) BGs, higher concentrations of CCMs, released from the BGs, led to an activation of the cells shown by increased expression of CD80 and CD86. On the other hand, BGs releasing copper led to a significant decrease of these surface markers, indicating that higher concentrations of copper induce a reduction of DC activation and maturation. Of note, the increased expression of co-stimulatory molecules, such as CD80 and CD86, went along with antigen transport to the surface of DCs. Both T cell receptor signaling and T cell activation are promoted by these molecules.64 The expression levels of CD83 were reduced to some extent by treatment with 10% B3–Cu and B3–Cu–Zn BGs, but CD25 was significantly influenced by treatment with these BGs. Overall, B3 and B3–Zn BGs promoted DC maturation, while treatment with B3–Cu (10%) and B3–Cu–Zn (10%) BGs induced rather an immature phenotype.
Furthermore, DCs matured in the presence of B3 and B3–Zn (in 10% and 1%) BGs showed an increased capacity to stimulate T-cell proliferation (Fig. 6), which is in accordance with the increased expression levels of maturation specific molecules on the cell surface. Additionally, these treated DCs affect T cell polarization into a more pro-inflammatory Th17 milieu, since the supernatants of co-cultures showed increased expression levels of pro-inflammatory cytokines like IL-17, IL-6, TNF-α and GM CSF (Fig. 7A and C). In contrast, the presence of copper modulated the capacity of DCs to stimulate T-cells in a dose-dependent manner. At high concentrations (10% B3–Cu and B3–Cu–Zn), the capacity of DCs to prime T cells was significantly reduced, whereas the 1% concentration increased the T-cell proliferative capacity. This effect could be further specified by measuring the cytokine expression levels within the supernatant of these co-cultures. In the case of B3–Cu BG conditioned medium (1% concentration), an increased production of cytokines like IL-1, IL-17A and IL-6 was observed (Fig. 7B and D), whereas the 10% concentration led to a decreased cytokine production. Of note, all BGs tested at a concentration of 0.1% did not alter DC mediated T cell proliferation. Taken together, our results show that DCs treated with different BGs can be differentially modulated both in respect to the T cell phenotype and the polarizing cytokine profiles. Our results indicate that IL-17 may be a key cytokine in BG driving the functional modulation of DC-T cell interaction. IL-17 has recently drawn much attention in the field of immunology,65 since this cytokine plays a key regulatory role in host defense and inflammatory diseases. IL-17 induces the production of many other cytokines (such as IL-6, GM-CSF, TNF-α, etc.). Moreover high levels of this cytokine are associated with several chronic inflammatory diseases, including rheumatoid arthritis, psoriasis and multiple sclerosis.65 In contrast to host defense, IL-17A has been shown to be beneficial against infections caused by extracellular bacteria and fungi.66 Furthermore IL-17A has been shown to recruit myeloid derived suppressor cells (MDSCs) to dampen the anti-tumor immunity.67 Thus, these bioactive materials are discussed as an interesting tool for DC specific regulation of different disease entities. To control the host immune response, either by reducing or enhancing the immune responses via DCs, BGs harbor an interesting potential as immune-modulators. Understanding the correlation between BG properties and DC phenotype/function is very important for future applications of BGs in vivo, since specific compositions of BGs could effectively modulate the immune system in both directions, inducing and dampening an immune response.
In summary, our results revealed that the presence of different compositions of BGs differentially interferes with the phenotype and function of DCs. The special properties of Cu-BGs could be of interest in circumstances where the immune system is overreacting, i.e. in the case of autoimmune disorders or in the case when one aims to prevent transplant rejections. On the other hand, some BGs, e.g. Zn doped BGs studied here, induced an enhanced DC mediated T cell proliferation, and these properties could be advantageous when one aims to induce a potent immune response, as in the case of infections or tumor growth. Thus, BGs possess exciting immune-modulatory properties and their prophylactic and therapeutic potential should be explored in more detail in future studies, particularly considering novel compositions with controlled ion releasing capability.
5. Conclusion
From the results of this study, we conclude that BGs, especially doped with copper and/or zinc, represent potent agents to avoid bacterial growth exploiting ion release during their dissolution in body fluids. Furthermore, BGs have very interesting immune-modulatory effects on DCs, which we identified here for the first time. Therefore in future studies we will further analyze their potential to specifically modulate the immune responses, (i) in the case of autoimmunity and transplantation and (ii) in the case of infections and neoplastic disorders.
Ethical statement
All animal procedures were performed in accordance with the Guidelines for Care and Use of Laboratory Animals of the German Animal Welfare Act and approved by the Unit of Animal Protection of the University of Erlangen-Nuremberg.
Conflicts of interest
There are no conflicts to declare.
Acknowledgements
Åbo Akademi's Johan Gadolin Scholarship is gratefully acknowledged for a visiting fellowship for KS. We thank Prof. Leena Hupa (Åbo Akademi, Turku, Finland) for support during the glass fabrication. We thank Dr. Linda Grosche (Department of Immune Modulation) for her contribution to the cover artwork.
References
- E. Fiume, J. Barberi, E. Verné and F. Baino, J. Funct. Biomater., 2018, 9, 24 CrossRef PubMed.
- L. L. Hench, Biomed. Glasses, 2015, 1, 1–11 Search PubMed.
-
D. S. Brauer and D. Möncke, in Bioactive Glasses: Fundamentals, Technology and Applications, 2017, pp. 61–88 Search PubMed.
- P. Balasubramanian, T. Büttner, V. Miguez Pacheco and A. R. Boccaccini, J. Eur. Ceram. Soc., 2018, 38, 855–869 CrossRef CAS.
- V. Miguez-Pacheco, L. L. Hench and A. R. Boccaccini, Acta Biomater., 2015, 13, 1–15 CrossRef CAS PubMed.
- S. Naseri, W. C. Lepry and S. N. Nazhat, J. Mater. Chem. B, 2017, 5, 6167–6174 RSC.
- J. R. Jones, Acta Biomater., 2015, 23, S53–S82 CrossRef PubMed.
- Q. Fu, M. N. Rahaman, H. Fu and X. Liu, J. Biomed. Mater. Res., Part A, 2010, 95, 164–171 CrossRef PubMed.
- Y. Li, M. N. Rahaman, Q. Fu, B. S. Bal, A. Yao and D. E. Day, J. Am. Ceram. Soc., 2007, 90, 3804–3810 CAS.
- P. Wray, Am. Ceram. Soc. Bull., 2011, 90, 25–29 CAS.
-
S. Fagerlund and L. Hupa, in Bioactive Glasses: Fundamentals, Technology and Applications, ed. A. R. Boccaccini, D. S. Brauer and L. Hupa, 2017, pp. 1–26 Search PubMed.
- A. Hoppe, N. S. Güldal and A. R. Boccaccini, Biomaterials, 2011, 32, 2757–2774 CrossRef CAS PubMed.
- D. S. Brauer, Angew. Chem., Int. Ed., 2015, 54, 4160–4181 CrossRef CAS PubMed.
- S. H. Luo, W. Xiao, X. J. Wei, W. T. Jia, C. Q. Zhang, W. H. Huang, D. X. Jin, M. N. Rahaman and D. E. Day, J. Biomed. Mater. Res., Part B, 2010, 95 B, 441–448 CrossRef PubMed.
- C. K. Sen, S. Khanna, M. Venojarvi, P. Trikha, E. C. Ellison, T. K. Hunt and S. Roy, Am. J. Physiol.: Heart Circ. Physiol., 2002, 282, H1821–H1827 CrossRef CAS PubMed.
- X. Wang, F. Cheng, J. Liu, J.-H. Smått, D. Gepperth, M. Lastusaari, C. Xu and L. Hupa, Acta Biomater., 2016, 46, 286–298 CrossRef CAS PubMed.
- K. Liu and M. C. Nussenzweig, Immunol. Rev., 2010, 234, 45–54 CrossRef CAS PubMed.
- A. Waisman, D. Lukas, B. E. Clausen and N. Yogev, Semin. Immunopathol., 2017, 39, 153–163 CrossRef CAS PubMed.
- M. Merad, J. Helft, P. Sathe, J. Miller and A. Mortha, Annu. Rev. Immunol., 2013, 31, 563–604 CrossRef CAS PubMed.
- J. Banchereau and R. M. Steinman, Nature, 1998, 392, 245–252 CrossRef CAS PubMed.
- R. M. Steinman and J. Banchereau, Nature, 2007, 449, 419–426 CrossRef CAS PubMed.
- M. B. Lutz and G. Schuler, Trends Immunol., 2002, 23, 445–449 CrossRef CAS PubMed.
- M. A. Wallet, P. Sen and R. Tisch, Clin. Med. Res., 2005, 3, 166–175 CrossRef CAS PubMed.
- B. M. Carreno and M. Collins, Annu. Rev. Immunol., 2002, 20, 29–53 CrossRef CAS PubMed.
- K. Schuhladen, X. Wang, L. Hupa and A. R. Boccaccini, J. Non-Cryst. Solids, 2018, 502, 22–34 CrossRef CAS.
- M. M. George, K. Subramanian Vignesh, J. A. Landero Figueroa, J. A. Caruso and G. S. Deepe, J. Immunol., 2016, 197, 1864–1876 CrossRef CAS PubMed.
- F. Zhu, Y. Tong, Z. Sheng and Y. Yao, Acta Biomater., 2019, 94, 132–144 CrossRef CAS PubMed.
- M. A. Ur Rehman, S. Ferraris, W. H. Goldmann, S. Perero, F. E. Bastan, Q. Nawaz, G. G. Di Confiengo, M. Ferraris and A. R. Boccaccini, ACS Appl. Mater. Interfaces, 2017, 9, 32489–32497 CrossRef CAS PubMed.
- M. B. Lutz, N. Kukutsch, A. L. J. Ogilvie, S. Roßner, F. Koch, N. Romani and G. Schuler, J. Immunol. Methods, 1999, 223, 77–92 CrossRef CAS PubMed.
- T. Zal, A. Volkmann and B. Stockinger, J. Exp. Med., 1994, 180, 2089–2099 CrossRef CAS PubMed.
- A. Selahattin, G. Kadri and C. Ramazan, Turk. J. Med. Sci., 1998, 28, 595–597 Search PubMed.
- G. Bruni, L. Visai, S. Fiorilli, M. Vallet-Regí, C. Vitale-Brovarone, A. Torres-Pardo, N. Bloise, J. M. González-Calbet, A. Bari and G. Novajra, Acta Biomater., 2017, 55, 493–504 CrossRef PubMed.
- M. N. Rahaman, D. E. Day, B. Sonny Bal, Q. Fu, S. B. Jung, L. F. Bonewald and A. P. Tomsia, Acta Biomater., 2011, 7, 2355–2373 CrossRef CAS PubMed.
- S. Kargozar, F. Baino, S. Hamzehlou, R. G. Hill and M. Mozafari, Trends Biotechnol., 2018, 36, 430–444 CrossRef CAS PubMed.
- F. E. Ciraldo, E. Boccardi, V. Melli, F. Westhauser and A. R. Boccaccini, Acta Biomater., 2018, 75, 3–10 CrossRef CAS PubMed.
- L. Drago, M. Toscano and M. Bottagisio, Materials, 2018, 11, 1–11 CrossRef PubMed.
- L. Drago, E. De Vecchi, M. Bortolin, M. Toscano, R. Mattina and C. L. Romanò, Future Microbiol., 2015, 10, 1293–1299 CrossRef CAS PubMed.
- J. Pasquet, M.-A. Bolzinger, Y. Chevalier, D. Bouvier, E. Couval and J. Pelletier, Colloids Surf., A, 2014, 457, 263–274 CrossRef CAS.
- J. D. Baldeck, T.-N. Phan, J. Sheng, R. E. Marquis and T. Buckner, Oral Microbiol. Immunol., 2003, 19, 31–38 Search PubMed.
- A. Wajda, W. H. Goldmann, R. Detsch, A. R. Boccaccini and M. Sitarz, J. Non-Cryst. Solids, 2019, 511, 86–99 CrossRef CAS.
- M. Vincent, R. E. Duval, P. Hartemann and M. Engels-Deutsch, J. Appl. Microbiol., 2018, 124, 1032–1046 CrossRef CAS PubMed.
- Q. Fu, M. N. Rahaman, B. Sonny Bal, L. F. Bonewald, K. Kuroki and R. F. Brown, J. Biomed. Mater. Res., Part A, 2010, 95, 172–179 CrossRef PubMed.
- Y. Gu, W. Huang, M. N. Rahaman and D. E. Day, Acta Biomater., 2013, 9, 9126–9136 CrossRef CAS PubMed.
- V. C. Modglin, R. F. Brown, S. B. Jung and D. E. Day, J. Mater. Sci. Mater. Med., 2013, 24, 1191–1199 CrossRef CAS PubMed.
- S. Chen, Q. Yang, R. K. Brow, K. Liu, K. A. Brow, Y. Ma and H. Shi, Mater. Sci. Eng., C, 2017, 73, 447–455 CrossRef CAS PubMed.
- Q. Yang, S. Chen, H. Shi, H. Xiao and Y. Ma, Mater. Sci. Eng., C, 2015, 55, 105–117 CrossRef CAS PubMed.
- J. Zhou, H. Wang, S. Zhao, N. Zhou, L. Li, W. Huang, D. Wang and C. Zhang, Mater. Sci. Eng., C, 2016, 60, 437–445 CrossRef CAS PubMed.
- P. Balasubramanian, L. Hupa, B. Jokic, R. Detsch, A. Grünewald and A. R. Boccaccini, J. Mater. Sci., 2016, 52, 8785–8792 CrossRef.
- L. M. Marquardt, D. Day, S. E. Sakiyama-Elbert and A. B. Harkins, J. Biomed. Mater. Res., Part A, 2014, 102, 2767–2775 CrossRef PubMed.
- A. Ali, M. Ershad, V. K. Vyas, S. K. Hira, P. P. Manna, B. N. Singh, S. Yadav, P. Srivastava, S. P. Singh and R. Pyare, Mater. Sci. Eng., C, 2018, 93, 341–355 CrossRef CAS PubMed.
- B. Gupta, J. B. Papke, A. Mohammadkhah, D. E. Day and A. B. Harkins, Ann. Biomed. Eng., 2016, 44, 3468–3477 CrossRef PubMed.
- S. Zhao, L. Li, H. Wang, Y. Zhang, X. Cheng, N. Zhou, M. N. Rahaman, Z. Liu, W. Huang and C. Zhang, Biomaterials, 2015, 53, 379–391 CrossRef CAS PubMed.
- Y. Lin, R. F. Brown, S. B. Jung and D. E. Day, J. Biomed. Mater. Res., Part A, 2014, 102, 4491–4499 Search PubMed.
- H. Wang, S. Zhao, J. Zhou, Y. Shen, W. Huang, C. Zhang, M. N. Rahaman and D. Wang, J. Mater. Chem. B, 2014, 2, 8547–8557 RSC.
- L. Bi, M. N. Rahaman, D. E. Day, Z. Brown, C. Samujh, X. Liu, A. Mohammadkhah, V. Dusevich, J. D. Eick and L. F. Bonewald, Acta Biomater., 2013, 9, 8015–8026 CrossRef CAS PubMed.
- F. Baino, S. Hamzehlou and S. Kargozar, J. Funct. Biomater., 2018, 9, 25 CrossRef PubMed.
- A. K. Tsirogianni, N. M. Moutsopoulos and H. M. Moutsopoulos, Injury, 2016, 37, S5–S12 CrossRef PubMed.
-
C. Schütt and B. Bröker, Grundwissen Immunologie, 2011 Search PubMed.
- P. M. Kou, N. Pallassana, R. Bowden, B. Cunningham, A. Joy, J. Kohn and J. E. Babensee, Biomaterials, 2012, 33, 1699–1713 CrossRef CAS PubMed.
- J. E. Babensee and A. Paranjpe, J. Biomed. Mater. Res., Part A, 2005, 74, 503–510 CrossRef PubMed.
- M. Yoshida and J. E. Babensee, J. Biomed. Mater. Res., Part A, 2017, 79, 393–408 Search PubMed.
- T. ten Broeke, R. Wubbolts and W. Stoorvogel, Cold Spring Harbor Perspect. Biol., 2013, 5, 1–23 Search PubMed.
- A. M. Dudek, S. Martin, A. D. Garg and P. Agostinis, Front. Immunol., 2013, 4, 1–14 CAS.
- T. S. Lim, J. K. H. Goh, A. Mortellaro, C. T. Lim, G. J. Hämmerling and P. Ricciardi-Castagnoli, PLoS One, 2012, 7, 1–8 CrossRef.
- W. Jin and C. Dong, Emerging Microbes Infect., 2013, 2, 1–5 CrossRef PubMed.
- J. K. Kolls and S. A. Khader, Cytokine Growth Factor Rev., 2010, 21, 443–448 CrossRef CAS PubMed.
- S. H. Chang, S. G. Mirabolfathinejad, H. Katta, A. M. Cumpian, L. Gong, M. S. Caetano, S. J. Moghaddam and C. Dong, Proc. Natl. Acad. Sci. U. S. A., 2014, 111, 5664–5669 CrossRef CAS PubMed.
|
This journal is © The Royal Society of Chemistry 2020 |