DOI:
10.1039/C9BM01310E
(Paper)
Biomater. Sci., 2020,
8, 426-437
3D spheroids generated on carbon nanotube-functionalized fibrous scaffolds for drug metabolism and toxicity screening
Received
19th August 2019
, Accepted 4th November 2019
First published on 5th November 2019
Abstract
The mechanical and electrical stimuli have a profound effect on the cellular behavior and function. In this study, a series of conductive nanofibrous scaffolds are developed by blend electrospinning of poly(styrene-co-maleic acid) (PSMA) and multiwalled-carbon nanotubes (CNTs), followed by grafting galactose as cell adhesion cues. When the mass ratios of CNTs to PSMA increase up to 5%, the alignment, Young's modulus and conductivity of fibrous scaffolds increase, whereas the average diameter, pore size and elongation at break decrease. Primary hepatocytes cultured on the scaffolds are self-assembled into 3D spheroids, which restores the hepatocyte polarity and sufficient expression of drug metabolism enzymes over an extended period of time. Among these conductive scaffolds, hepatocytes cultured on fibers containing 3% of CNTs (F3) show the highest clearance rates of model drugs, offering a better prediction of the in vivo data with a high correlation value. Moreover, the drug metabolism capability is maintained over 15 days and is more sensitive towards the inducers and inhibitors of metabolizing enzymes, demonstrating the applicability for drug–drug interaction studies. Thus, this culture system has been demonstrated as a reliable in vitro model for high-throughput screening of metabolism and toxicity in the early phases of drug development.
1. Introduction
Drug-induced hepatotoxicity is a leading cause of clinical trial failures and postmarket drug withdrawals. In vitro liver models have been adopted in the early stages of drug development to evaluate the hepatotoxicity and efficacy of drug candidates. Compared to animal models, in vitro hepatocyte-based models are more economical, easier to carry out, and less controversial. Freshly isolated human primary hepatocytes currently represent the gold standard for building in vitro liver models, but are limited by their scarce availability and high costs. Therefore, primary rats or murine hepatocytes are widely used for in vitro metabolic and toxicity studies. Yet primary hepatocytes typically undergo a rapid loss of liver specific functions and metabolic competence when maintained under conventional two-dimensional (2D) culture conditions.1,2 To overcome this limitation, three-dimensional (3D) hepatocyte cultures have been developed. 3D hepatocyte cultures improve cell–cell and cell–matrix interactions and better mimic liver functions, thus allowing for a more informed forecast of drug safety and efficacy. Spheroids are one of the most characterized and versatile 3D culture models for drug testing, liver engineering and liver diseases. They can be formed in spinner or rocker systems, in nanofiber scaffolds or hydrogels, and on decorated 2D substrates or micropatterned platforms.3 For example, Vorrink et al. used long-term stable primary hepatic 3D spheroid cultures as an in vitro model system for the evaluation of drug hepatotoxicity. This model accurately distinguished between hepatotoxic and nontoxic structural analogues with improved sensitivity and specificity.4
Cellular behaviors in native tissues are regulated by topographical, chemical, mechanical, and electrical cues which are offered by the extracellular matrix. In particular, electrical stimulation plays a critical role in the modulation of a multitude of cellular activities such as adhesion, proliferation, migration, differentiation, protein secretion, and DNA synthesis, and have shown to have an effect on cardiac myocytes, osteoblast, and skeletal muscle cells.5 Conductive polymers, such as polypyrrole, polyaniline, polythiophene and their derivatives, have been widely investigated in the field of tissue engineering. However, these conductive polymers exhibit poor polymer–cell interactions, solubility and processability, as well as high hydrophobicity and uncontrollable mechanical properties, hindering their wide application as scaffolding materials.6,7
Carbon nanotubes (CNTs) are considered a promising replacement for conventional conductive polymers, since they are inert, mechanically robust, electrically conductive and highly biocompatible. Numerous studies have suggested that CNTs have positive effects on the adhesion, growth, proliferation and differentiation of neural, bone, endothelial and smooth muscle cells.8 However, only a few studies have examined their effects on hepatocyte culture up to now. Koga et al. found that rat hepatocytes on single-walled CNT-coated scaffolds tended to form spheroids. The liver-specific functions of these spheroids were elevated compared with conventional monolayers.9 Abdullah et al. revealed that multiwalled CNT-based scaffolds could closely mimic the extracellular matrices (ECM) and improve liver-specific functions including albumin synthesis, metabolizing enzyme activity, and transporter activity.10 CNTs can be easily functionalized and incorporated into matrix materials to produce composites with unique properties, which may be optimal for tissue engineering and drug discovery applications.11 To better mimic the physiological environment, various nanofibrous scaffolds have displayed beneficial effects on the in vitro maintenance of primary hepatocytes. Chu et al. incorporated conductive polypyrrole into poly(lactic-co-glycolic) acid (PLGA) nanofibers via electrospinning and oxidative polymerization.12 The polypyrrole/PLGA conductive nanofibers could well retain the adhesion and cellular activities of hepatocytes, but there was no significant difference in hepatocyte functions between PLGA and polypyrrole/PLGA fibers.12 So far, the use of conductive scaffolds for in vitro maintenance of hepatocytes is still very limited and their applications in drug screening have not been reported.
Herein, a series of electrically conductive nanofibrous meshes with varied CNT contents were produced by blend electrospinning of poly(styrene-co-maleic acid) (PSMA) and multiwalled CNTs. Electrospinning is a facile and effective technique to generate composite fibers, which offer an ECM-mimicking nanofibrous architecture with high porosity and surface-to-volume ratio. Compared to the corresponding film scaffolds, electrospun fibers can attract more integrin-binding proteins and promote cell attachment, proliferation, migration and differentiation.13 Aligned fiber topography was conducive to the extension and growth of cardiac myocytes, osteoblasts, vascular cells, neural cells, and skeletal muscle cells.14 Galactose ligands can send an adhesive cue to hepatocytes by selectively interacting with asialoglycoprotein receptors (ASGPR) on the cell surface, which can help to maintain the cell morphology and trigger the spheroid formation, leading to a high level of liver-specific functions.15 Therefore, galactose moieties were decorated on the surfaces of the aligned conductive fibers to enhance cell–cell and cell–substrate interactions, and the morphologies, alignment, conductivities and mechanical properties of the resulting fibrous meshes were characterized. Primary hepatocytes were seeded on these fibrous meshes to investigate cell adhesion, morphology and proliferation. The fibrous scaffolds were further screened to form hepatocyte spheroids with better liver-specific functions (i.e. syntheses of albumin and urea and the activities of phase I and II metabolizing enzymes). The optimized 3D spheroids were utilized for drug metabolism tests to establish correlations of intrinsic in vitro and in vivo clearance. Moreover, the induction/inhibition responses to model drugs were investigated to present the potential application of this in vitro model for drug safety screening.
2. Results
2.1 Characterization of fibrous meshes
The electrically conducting fibrous meshes (F0, F1, F2, F3, F4 and F5) were prepared by blend electrospinning of CNTs and PSMA in the ratio of 0, 1%, 2%, 3%, 4% and 5% (w/w), followed by galactose grafting on the fiber surface. The PSMA/CNT ratios were varied to finely tune the fiber properties in terms of dispersity, alignment, diameter and pore size. Fig. 1a shows the SEM images of these fibrous meshes, revealing aligned fibers with a smooth and tenuous morphology. When the CNT content increased up to 5%, they could no longer disperse well in the electrospinning suspensions, resulting in the formation of aggregates or bundles in fibers (F5). With increasing CNT concentrations, the fiber alignments increased (Fig. 1b), while the fiber diameters and pore sizes of fibrous meshes decreased (Fig. 1c and d).
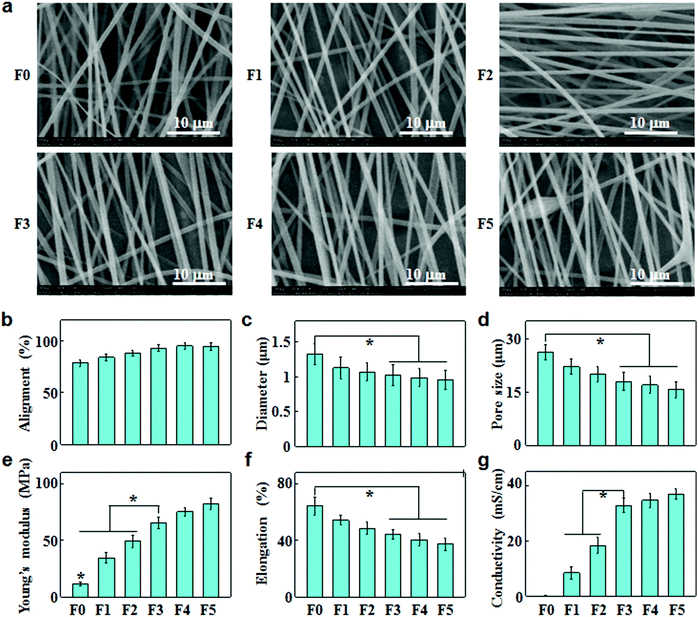 |
| Fig. 1 Characterization of conductive fibrous scaffolds. (a) SEM images, (b) alignment, (c) diameter, (d) pore sizes, (e) Young's modulus, (f) elongation and (g) conductivities of PSMA fibrous mats containing none (F0), 1% (F1), 2% (F2), 3% (F3), 4% (F4) and 5% (F5) of CNTs (n = 3, p < 0.05). | |
Based on the tensile stress–strain studies, the Young's modulus and elongation at break of fibrous meshes were calculated and are summarized in Fig. 1e and f. With increasing CNT contents, the Young's modulus increased simultaneously with a decrease in the elongation at break. The average Young's modulus/elongation at break values for F0, F1, F2, F3, F4 and F5 were 11.5/64.4, 34.6/54.4, 49.3/48.6, 65.3/44.4, 75.6/40.4, and 82.5/37.4 MPa/%, respectively. This suggested that the increase in CNT concentrations led to less elasticity but strengthened fibrous meshes.
Fig. 1g shows the conductivities of fibrous meshes with different amounts of CNTs. The conductivities of fibrous meshes increased with increasing CNT contents, and the addition of 3% CNTs led to a sharp increase in the conductivity. When the CNT content increased to 5%, the conductivity of F5 tended to be ‘saturated’ at 37 mS cm−1.
2.2 Morphology and polarity of hepatocytes on fibrous meshes
The morphologies of hepatocytes were observed by using a scanning electronic microscope (SEM) after culture for 10 days on fibrous meshes. As shown in Fig. 2a, the non-conductive fibrous meshes (F0) could not well induce spheroid formation. In contrast, hepatocytes on the conductive fibrous meshes (F3) self-assembled into 3D spheroids with a diameter of 100 μm, having multiple attachment points on the underlying fibrous meshes (Fig. 2b). The organization of F-actin in the hepatocyte spheroids was further assessed by tetramethylrhodamine isothiocyanate (TRITC)-phalloidin staining. Consistent with the SEM observation, the distribution of dense F-actin on the cell periphery indicated the formation of hepatocyte aggregates with strong cell–cell and cell–ECM interactions (Fig. 2c). In the liver, hepatocytes were polarized and their 3D arrangement included a functionally distinct bile canaliculus. The biliary excretory function of the hepatocyte spheroids was detected by staining with fluorescein diacetate, which diffused into cells and was hydrolyzed by intracellular esterases into fluorescent fluorescein and excreted by bile canaliculi.16 As shown in Fig. 2d, fluorescence signals were distributed in the intercellular space, indicating that these hepatocytes maintained their ability to uptake chemicals and secret bile acids.
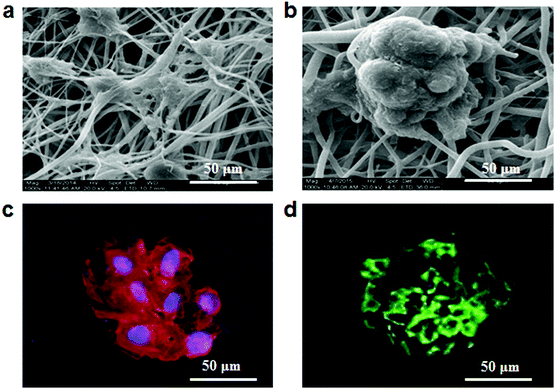 |
| Fig. 2 Characterization of hepatocyte spheroids. (a) Typical SEM image of hepatocytes after culture on F0 and (b) F3 fibrous meshes for 10 days. (c) CLSM images after F-actin staining with TRITC-phalloidin and (d) after fluorescein diacetate staining to reveal the hepatocyte polarity after culture for 10 days on F3 fibrous meshes. | |
2.3 Viability and function of hepatocytes on fibrous meshes
To evaluate their applicability as scaffolds for hepatocyte culture, fibrous meshes were first examined in terms of cell viabilities. As shown in Fig. 3a, the hepatocyte viabilities retained about 80% in all groups within the first day after the onset of culture. However, the cell viabilities in the non-conductive fiber group (F0) decreased rapidly after 3 days of culture and dropped to around 50% on day 10, and further reduced to around 20% on day 15. In comparison, the conductive fiber groups (F1, F2, F3, F4 and F5) showed significantly higher viabilities throughout the culture period. This result suggested that CNTs had beneficial effects on hepatocyte survival due to their conductive nature.9
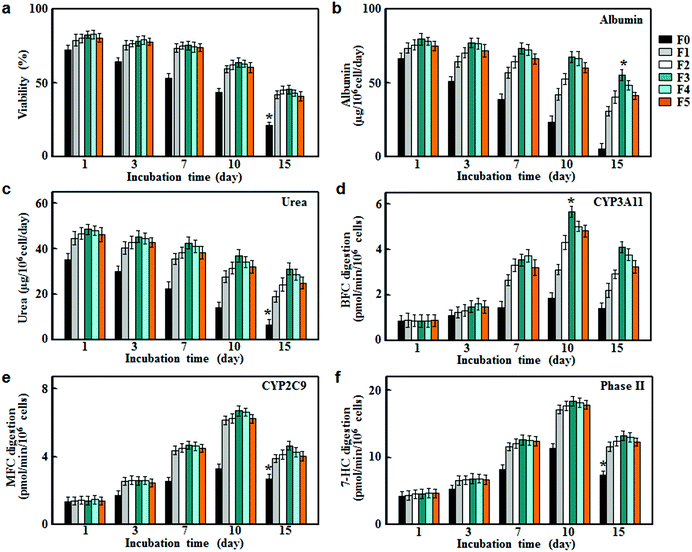 |
| Fig. 3 Hepatic function of hepatocyte spheroids. (a) Cell viability, (b) albumin secretion, (c) urea syntheses and (d) CYP3A11, (e) CYP2C9, and (f) phase II enzyme activities of hepatocyte spheroids after culture on F0, F1, F2, F3, F4 and F5 fibrous scaffolds for 15 days (n = 5, p < 0.05 vs. other groups). | |
To assess the stability of liver-specific functions for hepatocyte culture on fibrous meshes, we measured surrogate markers of protein synthesis and nitrogen metabolism. As shown in Fig. 3b and c, hepatocytes cultured on F0 lost their functional characteristics rapidly over time. In contrast, the albumin and urea secretion of hepatocytes on conductive fibers (F1, F2, F3, F4 and F5) were significantly higher at each time point and sustained during 15 days of incubation. In particular, hepatocytes cultured on F3 showed strong liver function for 15 days and the albumin and urea secretion rates were 55.4 ± 3.8 and 31.3 ± 2.5 μg per 106 cells per day, respectively. However, higher CNT levels (>3%) led to decreases in albumin and urea production (Fig. 3b and c), most likely due to the decreased cell viabilities (Fig. 3a).
Cytochrome P450 enzymes (CYPs) are involved in the oxidative activation or deactivation of both endogenous and exogenous compounds such as drugs, environmental toxins and dietary constituents. More than half of clinical drugs and xenobiotics in use today are metabolized by CYP3A4 (the human ortholog of the mouse CYP3A11)17 and CYP2C9.18 For each individual P450 activity assay, we selected the fluorescent probe that showed the highest metabolic rates. Specifically, 7-benzyloxy-4-trifluoromethylcoumarin (BFC), 7-methoxy-4-trifluoromethylcoumarin (MFC) and 7-hydroxycoumarin (7-HC) were selected to evaluate the drug metabolizing activities of CYP3A11, CYP2C9 and phase II enzymes respectively.19 As shown in Fig. 3d–f, hepatocytes on the fibrous meshes experienced a rapid increase in the enzymatic activities during 10 days of culture, followed by a slight decline. On day 10, the enzymatic activities in conductive fiber cultures increased by 3–6 fold, whereas the metabolic activities in F0 culture increased slightly (p < 0.05). Among the fibrous scaffold (F0, F1, F2, F3, F4 and F5) cultures, F3 culture showed the highest activities of CYP3A11, CYP2C9 and phase II enzymes on day 10, at 5.66, 6.62 and 18.3 pmol per min per 106 cells, respectively. Therefore, among the fibrous scaffolds, the F3 culture had the highest albumin and urea production as well as CYP activities thus it was selected for the following drug metabolism testing.
2.4 Gene expression of metabolic enzymes for hepatocytes on fibrous meshes
The activity variations of metabolic enzymes were also confirmed from mRNA expressions in hepatocyte spheroids. Fig. 4 summarizes mRNA levels of CYP3A11, CYP2C9, and phase II enzymes, normalized to that of β-actin, for hepatocyte spheroids after culture on F3, F0 and tissue culture plates (TCP). The mRNA levels on F3 fibrous meshes were significantly higher than those of F0 and TCP. After 10 days of culture on F3 fibrous meshes, the mRNA levels of CYP3A11, CYP2C9, and phase II enzymes were 2.39, 2.14 and 4.86 fold higher than those of F0. In addition, the culture on F3 for 15 days showed much less significant reductions in the mRNA levels of CYP3A11 (7.3%), CYP2C9 (15.3%), and enzymes (18.0%). The mRNA levels of CYP3A11, CYP2C9, and phase II enzymes for hepatocyte spheroids on F3 fibrous meshes after 15 days were 4.22, 3.90 and 23.1 fold higher than those on F0, respectively. It was indicated that hepatocyte spheroids on F3 fibrous meshes could retain significant higher expression levels of liver-specific genes relevant for the enzyme activity and drug metabolism, which paralleled the trend in the fluorometric activity analysis (Fig. 3).
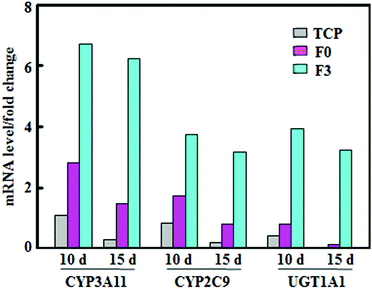 |
| Fig. 4 The mRNA levels of CYP3A11, CYP2C9, and UGT1A1 of phase II enzymes, normalized to that of β-actin, of hepatocyte spheroids after culture on TCP, F0 and F3 fibrous meshes for 10 and 15 days. | |
2.5
In vitro drug metabolism by hepatocytes on fibrous meshes
To assess the predictive capacities of F3 culture, the clearance rates of five model compounds were determined on F3, F0 and TCP cultures and compared with the in vivo clearance rates reported in the literature. Tolbutamide and S-warfarin were extensively used as the metabolic probes for enzyme CYP2C9. On day 10, the clearance rates of tolbutamide and S-warfarin were 0.43 and 0.24 mL min−1 kg−1 for F3 culture, which were significantly higher than those for F0 and TCP cultures (Fig. 5a and b). Moreover, they were close to the in vivo intrinsic clearance values for tolbutamide and S-warfarin (0.5 and 0.18 mL min−1 kg−1 respectively).20 After 15 days of F3 culture, the clearance rate of tolbutamide decreased slightly but was 1.9 and 5.5 fold greater than those after F0 and TCP cultures. Similarly, F3 culture displayed a slight decrease in the clearance rate of S-warfarin, which was still 1.8 and 5.0 fold higher than F0 and TCP cultures. Fig. 5c–e show the clearance of midazolam and testosterone by CYP3A11 and acetaminophen by phase II enzymes. On day 10, the clearance rates of testosterone, midazolam and acetaminophen for F3 culture were 56.4 ± 2.2, 81.2 ± 2.7 and 14.1 ± 0.3 mL min−1 kg−1 respectively, which were significantly higher than the corresponding values for F0 and TCP cultures. These data were quite close to the in vivo intrinsic clearance values for testosterone, midazolam and acetaminophen (62, 79 and 23.8 mL min−1 kg−1).20 F3 culture retained the higher metabolism capabilities after culture for 15 days compared with F0 and TCP cultures. The scaled clearance rates of testosterone, midazolam and acetaminophen for F3 culture were around 1.98, 2.17 and 2.07 times higher than those for F0 culture, respectively. Therefore, F3 culture maintained higher metabolic rates over long periods of time compared to F0 culture.
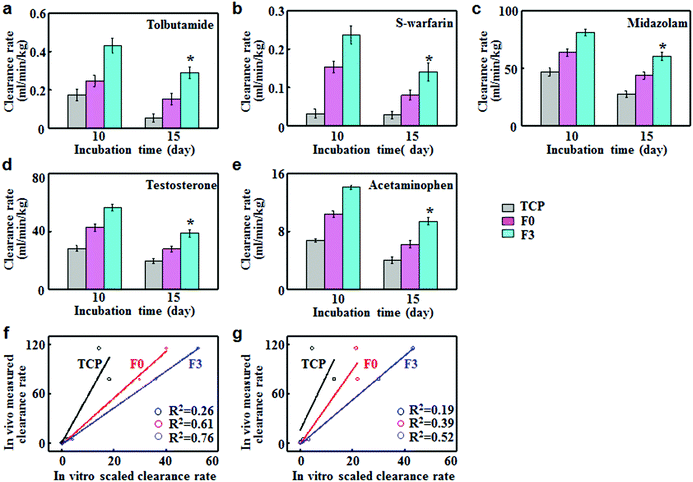 |
| Fig. 5 Drug metabolism examination on hepatocyte spheroids. (a) The in vitro scaled clearance rate of tolbutamide, (b) S-warfarin, (c) midazolam, (d) testosterone, and (e) acetaminophen during 2 h of metabolizing by hepatocyte spheroids after culture on F0 and F3 fibrous meshes for 10 and 15 days, compared with those after culture on TCP (n = 5). (f) The plots of in vitro scaled clearance rates against in vivo measured clearance rates of the above drugs by hepatocyte spheroids after culture on TCP, F0 and F3 fibrous meshes for 10 and (g) 15 days (n = 5, p < 0.05 vs. other groups). | |
In vitro–in vivo extrapolation (IVIVE) is a widely adopted method for predicting the hepatic metabolic clearance of drugs. Linear regression analyses were performed to evaluate the correlation between the predicted in vitro and actual in vivo clearance values of the five standard drugs.21 The correlation values (R2) indicated the accuracy of prediction for hepatic clearance. F3 culture displayed better correlation (R2 = 0.76) than F0 (R2 = 0.61) and TCP cultures (R2 = 0.26) after 10 days of culture (Fig. 5f). As shown in Fig. 5g, F3 culture had the lowest decline rate in R2 (0.52) after 15 days of culture, while the R2 value for TCP and F0 declined to 0.19 and 0.39 respectively. Therefore, F3 culture showed good correlation between the predicted and actual drug clearance for an extended period of time, thus representing an excellent in vitro model for drug metabolism testing.
2.6 Induction and inhibition effects of hepatocyte metabolism on fibrous meshes
The drug susceptibilities (inducer and inhibitor) were tested on the F3 culture, and compared with F0 and TCP cultures. As shown in Fig. 6, CYP3A4 and phase II enzymes were slightly induced or inhibited in TCP culture, while F3 culture led to the most significant inhibition or induction of CYPs after 10 and 15 days of incubation. Rifampicin and glutathione were used as inducers for CYP3A11 and phase II enzymes, respectively.22,23 The rifampicin treatment led to around 2.63 and 1.71-fold increases in the CYP3A11 activities for F3 and F0 cultures after 10 days of incubation, respectively. In the meantime, the activities of phase II enzymes were increased by around 2.08 and 1.80 fold, respectively. After 15 days of incubation, the increases in the enzyme activities were less significant compared with those after 10 days of incubation, and the inducer treatment led to more significant increases in F3 culture than those of F0. Ketoconazole and probenecid were used as the inhibitors of CYP3A11 and phase II enzymes, respectively.24,25 Under inhibition treatment, the activities of CYP3A11 and phase II enzymes showed around 43.3% and 37.5% reductions for F3 culture, while F0 culture exhibited less decrease in the enzyme activities (34.2% and 25.0% of decrease) after 10 days of incubation. After 15 days of incubation, the inhibition treatment on F3 culture still led to significant decreases in the activities of CYP3A11 and phase II enzymes, with about 38.5% and 34.4% of reduction, respectively.
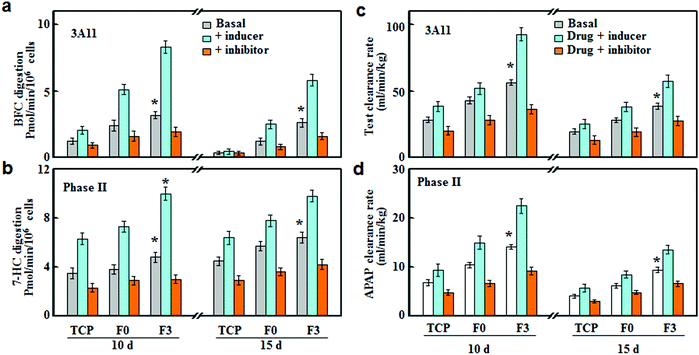 |
| Fig. 6 Drug–drug interaction examination on hepatocyte spheroids. (a) The CYP3A11 activities and (c) in vitro scaled clearance rates of testosterone by hepatocyte spheroids after culture on TCP, F0 and F3 fibrous meshes for 10 and 15 days in the presence of rifampicin and ketoconazole as an inducer and inhibitor of CYP3A11, respectively (n = 5). (b) The activities of phase II enzymes and (d) in vitro scaled clearance rates of acetaminophen by hepatocyte spheroids in the presence of glutathione and probenecid as an inducer and inhibitor of phase II enzymes, respectively (n = 5, p < 0.05 vs. other groups). | |
The inhibition or induction of CYPs can result in pharmacokinetic drug–drug interactions (DDI) with co-administered drugs, reducing drug effectiveness and causing unexpected adverse effects.26 Therefore, the evaluation of DDI is indispensable for an in vitro drug testing model to avoid potential therapeutic failures. Herein, we investigated the DDI as a result of the interference of the metabolic clearance of a drug by an enzyme inhibitor or inducer. The clearance rates of drugs (testosterone and acetaminophen) were determined on hepatocytes cultured on F3, F0 and TCP in the presence of a CYP inducer (rifampicin and glutathione) or inhibitor (ketoconazole and probenecid). As shown in Fig. 6c, after rifampicin induction treatment for 10 days, the testosterone clearance rates increased by around 1.64 and 1.35 fold for F3 and F0 cultures, respectively. Similarly, F3 and F0 cultures showed around 1.55 and 1.37 fold increases in the acetaminophen clearance rate after glutathione induction (Fig. 6c). It should be noted that the induction effect of rifampicin and glutathione retained after 15 days of culture and F3 culture exhibited higher drug clearance rates than F0 culture. For F3 culture, the clearance rates of testosterone and acetaminophen increased by around 1.43 and 1.44 fold, respectively, after induction treatment for 15 days. Fig. 6d summarizes the clearance rates of testosterone and acetaminophen after inhibition treatments. F0 culture experienced 29.3% and 29.4% of reductions in the clearance rates of testosterone and acetaminophen, while F3 cultures led to more significant decreases (36.2% and 35.1%) after 10 days of inhibition treatments. After 15 days of culture, the inhibition treatments were still effective at lowering the drug metabolism, and the clearance rates of testosterone and acetaminophen decreased by around 28.6% and 27.4% for F3 culture, respectively. Thus, F3 culture showed high sensitivities to CYP inducers and inhibitors over a long period of time, indicating that F3 culture could be applied for drug metabolism and DDI studies.
3. Discussion
Owing to their exceptional electrical and mechanical properties, CNTs have been extensively used to reinforce electrospun fibers in the field of neural or cardiac tissue engineering.27 The morphological, conductive and mechanical properties of the composite fibers could be regulated by adding different contents of CNTs. With increasing CNT levels, the fiber alignment increased while the fiber diameter decreased (Fig. 1b and c). The addition of CNTs enhanced the charge accumulation inside fibers, overcoming cohesive forces while intensifying repulsive forces among the charges. Thus, CNTs could align along the fiber axis during electrospinning, resulting in aligned fibers with smaller diameters.28 By increasing CNT concentrations in fibers, the Young's moduli reinforced, but the elongations at break decreased (Fig. 1e and f). The reinforcement was probably due to the impediment of crazing extension by CNT alignment in the crazing area, reduction of stress concentration by homogeneous dispersion of CNTs, and energy dissipation by pullout.28 The dispersion of CNTs in the polymer matrix was a major factor for the reinforcement effect. The reinforcement was prominent when CNTs were homogeneously dispersed in the concentration range of 1%–4%, whereas the Young's moduli had a slight increase when CNTs began to agglomerate and form tight bundles at 5% (Fig. 1a and e). The diminishing elongation at break might be attributed to the restricted mobility of polymer chains and stiffened fibers as a result of increasing CNT contents. Similar phenomena were observed by other researchers. For instance, Shao et al. investigated the mechanical properties of random oriented and aligned poly-DL-lactide (PLA)/CNT fibers, indicating that Young's modulus and maximum tensile strength increased while elongation decreased with increasing CNT concentrations.29
It has been reported that CNTs form a network in composite fibers to cause the electron transport by tunneling or electron hopping along CNT interconnects.30 Therefore, by increasing the concentration of CNTs, the surface resistance of fibers decreased, leading to a remarkable increase in the fiber conductivity (Fig. 1g). Based on the electrical percolation theory, the system became conductive when a critical concentration was reached which was called the percolation threshold. The composite fibers showed a percolation threshold of about 3% CNTs in PSMA, as the fiber conductivity increased dramatically above the threshold and became approximately constant as the CNT concentrations further increased (Fig. 1g). In addition, the alignment of CNTs within the host polymer was demonstrated to be beneficial for the mechanical and electrical properties of electrospun fibers based on polymer/CNT composites.28 Therefore, the orientation of these fibers was controlled to obtain a high alignment of CNTs along the fiber axis, and the aligned CNT/polymer composite fibers had higher Young's modulus and conductivity than randomly oriented ones.31 As shown in Fig. 1, when the CNT concentration reached 3%, the composite fibers exhibited superior alignment, conductivity and mechanical strength. This might be the reason that the culture of hepatocyte spheroids on F3 scaffolds achieved excellent liver-specific functions.
It is known that ion flows through channels and pumps segregate charges and generate a voltage across cell membranes called the resting membrane potential.32 The conductivities of the scaffolds increased the electrical communications, triggering signal cascades and leading to changes in resting membrane potentials of cells on conducting scaffolds. These changes could affect several specific subcellular events, including the import of Ca2+ and small molecule signals, changes in phosphatase activity and conformational changes in integrin signals, influencing the overall cell behaviors such as cell attachment, proliferation and migration.32 Therefore, the high proliferation rate of hepatocytes could be attributed to the enhanced electrical communications through conductive scaffolds.
Hepatocytes in their native environment have a unique polarized structure with apical and basolateral membrane domains which are responsible for the secretion of bile salts and serum proteins respectively. The reproduction and maintenance of hepatocyte polarity is essential for in vitro models to investigate metabolism and toxicology that are closely related to hepatic functions.33 The conventional methods for polarity establishment involved primary hepatocyte cultivation with ECM components in sandwich configuration, such as collagen, Matrigel and heparin gel sandwich cultures.34,35 Compared to hydrogel-based sandwich cultures, 3D multicellular spheroid cultures induced extensive cell–cell interactions that were essential for repolarization, thus representing the prevalent in vitro models for studies of drug metabolism and toxicity in the liver.36 In the current study, we developed the galactose grafted PSMA/CNT fibrous mats which promoted primary hepatocytes to generate polarized spheroids with functional bile canaliculi (Fig. 2). In contrast, the fibrous mats without CNTs could not induce spheroids, suggesting that the electrical signal was beneficial for hepatocyte polarity. This might be due to the indispensable role of cell–cell interaction in hepatic polarity and CNTs enhanced the interaction through electrical communication. Abdullah et al. fabricated aligned nanogrooved multiwalled-CNT sheets for sandwich cultures to recover and retain the polarity of hepatocytes, whereas the hepatocytes cultured in a monolayer displayed a flat and spread-out morphology.10 It was indicated that these 2D CNT sheets couldn't recreate the polarity unless they were organized in sandwich configuration for spatial presentation of polarity cues. In comparison, we integrated CNTs into 3D fibrous mats, which provided a ECM-mimicking fibrous structure, stiffness, electrical stimulation and adhesive signal, thus repolarization could be easily achieved by cultivating hepatocytes with F3.
As the cell polarity was correlated with hepatic functions such as chemical uptake, excretion and metabolism,33,37 F3 promoted the protein expression of hepatocytes, such as albumin, urea and metabolic enzymes and showed higher drug metabolism ability for an extended period of time compared to non-conductive scaffolds (Fig. 3, 4 and 5). F3 combined the remarkable mechanical and electronic properties of CNTs and ECM-mimicking fibrous structures to reconstitute physiological liver microenvironments. Therefore, spheroids cultured on these scaffolds could better mimic the in vivo liver, which could be used as an in vitro model for the prediction of in vivo drug clearance (Fig. 5f and g). Up to now, various in vitro liver models have been developed for drug metabolism testing. Sohlenius-Sternbeck et al. used the well-stirred model to predict the drug clearance rates by human hepatocytes and the R2 value for this system was 0.72.38 Chao et al. investigated the metabolic rates of acidic, basic and neutral drugs based on the microsome of liver tissues, and the calculated in vitro clearance correlated well with the corresponding in vivo values (R2 = 0.77).39 In addition, F3 cultures had a great advantage in simplicity compared with the in vitro models mentioned above. Therefore, F3 cultures exhibited great prediction in drug clearance values (R2 = 0.76) and acute responsiveness to drug–drug interactions (Fig. 6), making this easy-to-build system a potential model for in vitro drug testing.
4. Conclusions
We explored galactose grafted PSMA nanofibrous meshes with varied CNT contents, which provided topographical, electrical, mechanical and chemical cues to facilitate the formation and growth of hepatocyte spheroids. The PSMA/CNT ratios were varied to fine tune the properties of fibers in terms of dispersity, alignment, diameter, pore size, conductivity and mechanical properties. Cultured hepatocyte spheroids on these conductive meshes possessed good cell viability, in vivo-like structural polarity and functional bile canaliculi, thus retaining a high level of liver-specific functions. Among these conductive scaffolds, F3 culture had the highest albumin and urea production as well as CYP activities. We further investigated potential uses of F3 culture for drug development applications, and compared with TCP and non-conductive scaffold (F0) cultures. The clearance rates of five model compounds determined on F3 culture were significantly higher and correlated better with the actual in vivo clearance values than other groups, indicating greater predictive capacity for an extended period of time. In addition, F3 culture showed higher sensitivities to CYP inducers and inhibitors over 15 days, demonstrating its applicability for DDI studies. Therefore, F3 culture was able to mimic numerous features of in vivo liver and demonstrated great potential as an in vitro model with improved predictive capacity for the rapid screening of drug metabolism and hepatotoxicity.
5. Experimental
5.1 Materials
PSMA (Mw = 150 kDa, maleic anhydride content: 15 wt%) was obtained from Shanghai Zhaocheng Scientific Development Inc. (Shanghai, China). Multiwalled CNTs (outer diameter: 10–20 nm, length: 10–20 mm) were obtained from the Chengdu Institute of Organic Chemistry, Chinese Academy of Sciences (Chengdu, China). Lactobionic acid was purchased from Shanghai Kayon Biological Technology Inc. (Shanghai, China). 4,6-Diamidino-2-phenylindole dihydrochloride (DAPI) and TRITC-phalloidin were procured from Sigma-Aldrich (St Louis, MO), and fluorescein diacetate was obtained from Molecular Probes (Carlsbad, CA). BFC, MFC, and 7-HC were supplied by Tianjin Heowns Medicine Co. Ltd (Tianjin, China). Tolbutamide, S-warfarin, midazolam, testosterone, acetaminophen, rifampicin, glutathione, ketoconazole and probenecid were from Dalian Meilun Medicine Group Co. Ltd (Dalian, China). All other chemicals and solvents were of reagent grade or better, and purchased from Chengdu Kelong Reagent Co. (Chengdu, China) unless otherwise indicated.
5.2 Fabrication of conductive fibrous meshes
Multiwalled CNTs were dispersed in N,N-dimethylformamide with a concentration of 3%(w/v) under ultrasonication for 2 h and then stirred continuously overnight at room temperature. PSMA solutions in dichloromethane (15%, w/v) were mixed with well-dispersed CNT suspensions at various mass ratios of CNTs to PSMA (0%, 1%, 2%, 3%, 4% and 5%). These suspensions were ultrasonicated for 1 h and then stirred continuously for 24 h. Thereafter, the PSMA/CNT suspensions were loaded into a syringe with a stainless steel needle as the nozzle, and continuously pushed by using a microinjection pump (Zhejiang University Medical Instrument Co., Hangzhou, China) at a flow rate of 1.6 mL h−1. The electrospinning was operated under a fixed electric field of 20 kV/10 cm, using a high-voltage station (Tianjing High Voltage Power Supply Co., Tianjing, China). The aligned fibers were collected by using a rotating cylinder, followed by vacuum drying to completely remove solvent residues.19
Galactosylated fibrous meshes were obtained via a condensation reaction between the monoamino terminated galactose and the anhydride groups on the fiber surface as described previously.40 Briefly, PSMA/CNT fibrous meshes (100 mg) were immersed in 75% ethanol solution to wet their surfaces. After the removal of the ethanol solution and water rinsing, the meshes were incubated with 1% (w/w) aminated galactose solution and kept for 12 h with gentle shaking. Galactosylated meshes were thoroughly rinsed with distilled water and lyophilized.
5.3 Characterization of fibrous meshes
The meshes were coated in gold using sputter coating to observe their surface topographies by SEM (FEI, Quanta 200, Philips, Netherlands) at an accelerating voltage of 20.0 kV. The diameter, alignment, pore size and porosity of these fibrous meshes were measured from SEM images using Image J as described previously.41 The stress–strain analysis of the fibrous mesh was performed using a universal test machine (Instron 5567, Instron Co., MA). The samples were trimmed into cuboids with a length of 50 mm, width of 10 mm and thickness of 0.2 mm, and the distance between the gripping points was 30 mm. The tensile test was conducted with a 5 N load cell and a stretching speed of 2 mm min−1. The data acquisition ratio was set to 20.0 Hz. The conductivity of the fibrous meshes was measured using the four-point probe technique with an impedance/gain-phase analyzer (Solartron 1260) and electrochemical interface (Solartron 1287, Farnborough Hampshire, UK).42 The average value of the conductivities at different random sites represented the conductivity of fibrous meshes.
5.4 Hepatocyte culture on fibrous meshes
All animal procedures were performed in accordance with the National Institutes of Health Guide for Care and Use of Laboratory Animals of China and approved by the Animal Care and Use Committee of Southwest Jiaotong University. Male rats weighing from 20 to 25 g were purchased from Sichuan Dashuo Biotech Inc. (Chengdu, China). Primary hepatocytes were obtained by isolation from the livers of adult rats using a collagenase perfusion procedure as described previously.43 Briefly, the rats were sterilized in 75% ethanol to retrieve livers. The livers were quartered, and then repeatedly digested with trypsin and collagenase type II (Worthington Biochemical Corp., Lakewood, NJ) in Hank's balanced salt solution (Gibco, Carlsbad, CA). Cell suspensions were purified by gradient centrifugation. Hepatocytes with viability greater than 90% according to trypan blue exclusion assay were suspended in RPMI-1640 medium (Gibco BRL, Rockville, MD) supplemented with 10% fetal bovine solution, 100 U mL−1 penicillin and 100 mg mL−1 streptomycin (Gibco Invitrogen, Grand Island, NY), and adjusted to 1.0 × 106 viable cells per milliliter. Prior to cell seeding, fibrous meshes were cut into small squares with an area of 2 × 2 cm2 and sterilized under ultraviolet light overnight. Hepatocytes were seeded onto the fibrous meshes at a density of 5 × 105 cells per well in 12-well TCPs. The culture media were changed every alternate day.
5.5 Characterization of hepatocyte on the fibrous meshes
The viabilities and morphologies of hepatocytes cultured on the fibrous meshes were characterized by cell number quantification and microscopic techniques. The number of hepatocytes on fibrous meshes were quantified based on a DNA analysis method. Briefly, after incubation for 1, 3, 7, 10 and 15 days, cell-seeded fibrous meshes were retrieved and washed three times with PBS, and lysed with 0.5% Triton X-100 at 37 °C for 30 min. DNA concentration was quantified by using a PicoGreen dsDNA quantification kit (Molecular Probes, Grand Island, NY) according to the manufacturer's protocol.
The cell-loaded fibrous meshes were collected after culture for 10 days, fixed with 4% paraformaldehyde in PBS, and rinsed with distilled water. The samples were dehydrated through a series of graded ethanol solutions and then freeze-dried, and then the cell morphology was analyzed using SEM.41 In another batch of experiment, the samples were incubated with 0.2 μg mL−1 of TRITC-phalloidin for 20 min at 37 °C, followed by extensive washing with PBS. After counterstaining for 5 min with DAPI, the stained actin filaments (F-actin) of hepatocytes were observed by using a confocal laser scanning microscope (CLSM).44 The biliary excretion of hepatocytes on the fibrous meshes was determined by fluorescein diacetate staining as described previously.16 Briefly, cell-seeded fibrous meshes were incubated with 2 μg mL−1 of fluorescein diacetate in culture media for 45 min. After extensive washing with PBS, meshes were observed by CLSM under the excitation/emission wavelengths of 488/533 nm.
5.6 Hepatocyte functions on fibrous meshes
Albumin secretion and urea synthesis of hepatocytes on fibrous meshes were determined from their concentrations in the media conditioned as described previously.44 Briefly, media were collected after incubation for 1, 3, 7, 10 and 15 days, followed by centrifugation to collect the supernatant. The albumin secretion was determined by an enzyme-linked immunosorbent assay (ELISA) with an albumin quantification kit (Nanjing Jiancheng Bioengineering Institute, Nanjing, China). Urea synthesis was determined from its specific reaction with diacetylmonoxime by using a urea nitrogen kit (Nanjing Jiancheng Bioengineering Institute, Nanjing, China). Absorbance was measured by using a microplate reader (Elx-800, Bio-Tek Instruments Inc., Winooski, VT), and the amounts of albumin secretion and urea synthesis during 24 h were normalized by the cell numbers.
The activities of CYP3A11, CYP2C9 and phase II enzymes were determined using the specific substrates of BFC, MFC and 7-HC, respectively.19 Briefly, the substrates were dissolved in dimethyl sulfoxide and then diluted in culture medium. After culture for 1, 3, 7, 10 and 15 days, the hepatocytes on the meshes were incubated with 50 μM fluorogenic substrates. After incubation for 10, 20, 40, 60, 80 and 120 min, the media were retrieved from each well and added into a 96-well TCP, followed by the addition of 200 μL of acetonitrile to stop the reaction. All samples were sonicated for 3 min and centrifuged at 4000 rpm for 20 min. The fluorescence intensities of the supernatant were detected by using a fluorescence spectrophotometer (Hitachi F-7000, Japan), at the exciting/emission wavelength of 410/538, 333/416 and 370/450 nm for BFC, MFC and 7-HC, respectively. Standard curves were established with different concentrations of BFC, MFC and 7-HC, and the enzyme activities were normalized by the cell numbers.
5.7 Gene expression of enzymes from hepatocytes on fibrous meshes
Gene expressions profiles for CYP3A11, CYP2C9 and phase II enzymes were determined by a quantitative reverse-transcription polymerase chain reaction (RT-PCR) as described previously,45 and compared to that of β-actin. Due to the complicated phase II detoxification pathways, only UGT1A1, a major phase II metabolism enzyme, was determined.45 All the premiers for CYP3A11, CYP2C9, UGT1A1, and β-actin were synthesized by Beijing AuGCT DNA-SYN Biotechnology Co., Ltd (Beijing, China) and their sequences are designed as follows.46 CYP3A11 forward: TCTGTGCAGAAGCATCGAGTG and reverse: TGGGAGGTGCCTTATTGGG; CYP2C9 forward: AAAAGCACAATCCGCAGTCT and reverse: GCATCTGGCTCCTGTCTTTC; UGT1A1 forward: GGGATTCTCAGAATCTAGACATT and reverse: GTGTGTGGTATAAATGCTGTAGG; and β-actin forward: CTGGCACCCAGCACAATG and reverse: GCCGATCCACACGGAGTACT. The total mRNA from hepatocytes was prepared using Trizol (Aidlab, China) according to the manufacturer's instructions, and determined by optical densities at 260 nm using a spectrophotometer. The reverse transcription was carried out to generate complementary DNA (cDNA) using a TUREscript 1st-strand cDNA synthesis kit (Aidlab, China) according to the manufacturer's instructions. 10 ng of cDNA sample and 1 μL of 10 μM primer were added to 10 μL of the reaction mixture containing a SYBR Green I master mixture in a 96-well reaction plate. The thermocycling conditions were 95 °C for 10 s, 40 cycles of 95 °C for 5 s and 60 °C for 20 s, followed by a dissociation curve step. The reaction was performed using a 7500 Fast Real-Time PCR system (Applied Biosystems Asia, Singapore), and the gene transcription was evaluated using the ΔΔCt method and normalized to β-actin.47
5.8
In vitro drug metabolism by hepatocytes on fibrous meshes
Hepatocytes cultured on fibrous meshes were subjected to drug metabolism tests. Briefly, tolbutamide, S-warfarin, midazolam, testosterone and acetaminophen were dissolved in dimethyl sulfoxide to prepare stock solutions and diluted to working concentrations with culture media before using. After the culture of hepatocytes on the meshes for 10 and 15 days, 20 mM of these drugs were added and incubated for 2 h at 37 °C. The media were retrieved from each well, sonicated for 3 min and centrifuged at 4000 rpm for 5 min. Drug concentrations in the media were determined by high-performance liquid chromatography (HPLC, Agilent 1260 Infinity) using an Agilent C18 column. The mobile phase was a gradient with 0.1% formic acid in water (A) and 0.1% formic acid in acetonitrile (B) with a flow rate of 0.8 mL min−1. The initial composition of the mobile phase was 5% of B for 0.1 min, followed by a linear gradient to 90% of B over 1.1 min, held at 90% B for 0.2 min, and back to 5% B in 0.1 min.
For inducer and inhibitor studies, hepatocytes on the meshes were incubated with media in the presence of specific inducers (rifampicin for CYP3A11, glutathione for phase II enzymes) and inhibitors (ketoconazole for CYP3A11, probenecid for phase II enzymes) for 10 and 15 days.19 The reactions were stopped by the collection of the incubation media in fluorescein (BFC for CYP3A11, 7-HC for phase II enzymes), and followed by a rapid addition of 200 ml acetonitrile. The fluorescence intensities of the supernatant were detected by using a fluorescence spectrophotometer (Hitachi F-7000, Japan). Similarly, testosterone and acetaminophen were added into the incubation media to study pharmacological interaction for CYP3A11 and phase II enzymes.
The in vitro intrinsic clearance (CLin vitro) of each model drug was calculated from the substrate disappearance rate in the culture media according to the following equation:39
where
C0 and
Ct were the substrate concentration (μM) of a compound at time 0 and
t (min), respectively, and AUC
0−t was the area under the concentration–time curve from 0 to
t min with a unit of μM.
V was the volume of the incubation solution (μL), and
N was the number of hepatocytes. The intrinsic clearance was further normalized by 10
6 hepatocytes to have a unit of mL per min per 10
6 hepatocytes. In addition, the scaled hepatic clearance rates (CL
H, mL min
−1 kg
−1) were estimated from CL
in vitro using the well-stirred model as follows:
39where
Qh was the hepatic blood flow (90 mL min
−1 kg
−1), and
fu was the ratio of unbound (free) drug in the plasma to the total amount, which was 0.04, 0.11, 0.04, 0.4, and 0.82 for tolbutamide, S-warfarin, midazolam, testosterone, and acetaminophen, respectively.
19 The scaling factor (SF) was 6 × 10
9 kg
−1, assuming that 1 g of liver contained 10
6 hepatocytes, and the weight ratio was 50 g liver per kg body weight.
19
5.9 Statistical analysis
Statistical comparisons of specific pairs of data were undertaken using paired Student's t tests. The results were expressed as mean ± standard deviation (SD). The results with p values less than 0.05 were considered statistically significant.
Conflicts of interest
There are no conflicts to declare.
Acknowledgements
This work was supported by the National Natural Science Foundation of China (31771034) and the Analytical and Testing Center of Southwest Jiaotong University for SEM and CLSM analyses.
References
- J. H. Brown, P. Das, M. D. D. Vito, D. Ivancic, L. P. Tan and J. A. Wertheim, Acta Biomater., 2018, 73, 217 CrossRef CAS.
- G. Tuschl and S. O. Mueller, Toxicology, 2006, 218, 205 CrossRef CAS.
- M. W. Laschke and M. D. Menger, Trends Biotechnol., 2017, 35, 133 CrossRef CAS.
- S. U. Vorrink, Y. Zhou, M. Ingelman-Sundberg and V. M. Lauschke, Toxicol. Sci., 2018, 163, 655 CrossRef CAS.
- C. Ning, Z. Zhou, G. Tan, Y. Zhu and C. Mao, Prog. Polym. Sci., 2018, 81, 144 CrossRef CAS.
- G. Kaur, R. Adhikari, P. Cass, M. Bown and P. Gunatillake, RSC Adv., 2015, 5, 37553 RSC.
- X. Li, T. Zhao, L. Sun, K. E. Aifantis, Y. Fan, Q. Feng, F. Cui and F. Watari, J. Biomed. Mater. Res., Part A, 2016, 104, 322 CrossRef.
- F. M. P. Tonelli, A. K. Santos, K. N. Gomes, E. Lorençon, S. Guatimosim, L. O. Ladeira and R. R. Resende, Int. J. Nanomed., 2012, 7, 4511 CAS.
- H. Koga, T. Fujigaya, N. Nakashima and K. Nakazawa, J. Mater. Sci. Mater. Med., 2011, 22, 2071 CrossRef CAS.
- C. A. C. Abdullah, C. L. Azad, R. Ovalle-Robles, S. Fang, M. D. Lima, X. Lepró, S. Collins, R. H. Baughman, A. B. Dalton, N. J. Plant and R. P. Sear, ACS Appl. Mater. Interfaces, 2014, 6, 10373 CrossRef.
-
J. Kaur, G. S. Gill and K. Jeet, Characterization and Biology of Nanomaterials for Drug Delivery, ed. S. S. Mohapatra, S. Ranjan, N. Dasgupta, R. K. Mishra, S. Thomas, Elsevie, Amsterdam, Netherlands, 2019, ch. 5, pp. 113–135 Search PubMed.
- X. H. Chu, Q. Xu, Z. Q. Feng, J. Q. Xiao, Q. Li, X. T. Sun, Y. Cao and Y. T. Ding, Mater. Res. Express, 2014, 1, 035402 CrossRef.
- X. Wang, B. Ding and B. Li, Mater. Today, 2013, 16, 229 CrossRef CAS.
- C. Wu, H. Luan, S. Wang, X. Zhang, R. Wang, L. Jin, P. Guo and X. Chen, Molecules, 2013, 18, 15624 CrossRef CAS.
- R. A. Perez, C. R. Jung and H. W. Kim, Adv. Healthcare Mater., 2017, 6, 1600791 CrossRef PubMed.
- M. Sun, J. Y. Wong, B. Nugraha, A. Ananthanarayanan, Z. Liu, F. Lee, K. Gupta, E. L. S. Fong, X. Huang and H. Yu, Biomaterials, 2019, 201, 16 CrossRef CAS.
- S. Liu, Y. Cheng, M. Rao, M. Tang and Z. Dong, Pharmacology, 2017, 99, 205 CrossRef CAS.
- P. Manikandan and S. Nagini, Curr. Drug Targets, 2018, 19, 38 CAS.
- J. Wei, J. Lu, Y. Liu, S. Yan and X. Li, J. Mater. Chem. B, 2016, 4, 7155 RSC.
- I. A. M. Graaf, R. Kanter, M. H. Jager, R. Camacho, E. Langenkamp, E. G. V. Kerkhof and G. M.
M. Groothuis, Drug Metab. Dispos., 2006, 34, 591 CrossRef.
- P. Poulin, C. E.C.A. Hop, Q. Ho, J. S. Halladay, S. Haddad and J. R. Kenny, J. Pharm. Sci., 2012, 101, 4308 CrossRef CAS.
- Y. Yamasaki, K. Kobayashi, A. Inaba, D. Uehara, H. Tojima, S. Kakizaki and K. Chiba, Xenobiotica, 2018, 48, 1098 CrossRef CAS.
- S. Xu, D. Hou, J. Liu and L. Ji, Redox Rep., 2018, 23, 213 CrossRef CAS.
- Z. Zhou, Y. Lin, L. Gao, Z. Yang, S. Wang and B. Wu, Toxicol. Lett., 2019, 313, 188 CrossRef CAS.
- S. Ge, Y. Tu and M. Hu, Curr. Pharmacol. Rep., 2016, 2, 326 CrossRef CAS.
- V. Chu, H. J. Einolf, R. Evers, G. Kumar, D. Moore, S. Ripp, J. Silva, V. Sinha, M. Sinz and A. Skerjanec, Drug Metab. Dispos., 2009, 37, 1339 CrossRef CAS.
- Y. Liu, X. Liang, S. Wang and K. Hu, J. Biomater. Tissue Eng., 2016, 6, 719 CrossRef.
- S. G. King, N. J. Terrill, A. J. Goodwin, R. Stevens, V. Stolojan and S. R. P. Silva, Carbon, 2018, 138, 207 CrossRef CAS.
- S. Shao, S. Zhou, L. Li, J. Li, C. Luo, J. Wang, X. Li and J. Weng, Biomaterials, 2011, 32, 2821 CrossRef CAS.
- C. C. Cheng, Y. S. Wang, J. K. Chen and D. J. Lee, J. Mater. Chem. C, 2016, 4, 5207 RSC.
- S. Jiang, Y. Chen, G. Duan, C. Mei, A. Greiner and S. Agarwal, Polym. Chem., 2018, 9, 2685 RSC.
-
M. Raghavan, D. Fee and P. E. Barkhaus, Generation and propagation of the action potential, ed. K. H. Levin, P. Chauvel, Elsevier, Amsterdam, Netherlands, 2019, ch. 1, pp. 3–22 Search PubMed.
- A. Zeigerer, A. Wuttke, G. Marsico, S. Seifert, Y. Kalaidzidis and M. Zerial, Exp. Cell Res., 2017, 350, 242 CrossRef CAS PubMed.
- D. Deharde, C. Schneider, T. Hiller, N. Fischer, V. Kegel, M. Lübberstedt, N. Freyer, J. G. Hengstler, T. B. Andersson, D. Seehofer, J. Pratschke, K. Zeilinger and G. Damm, Arch. Toxicol., 2016, 90, 2497 CrossRef CAS.
- E. Foster, J. You, C. Siltanen, D. Patel, A. Haque, L. Anderson and A. Revzin, Eur. Polym. J., 2015, 72, 726 CrossRef CAS.
- L. J. Y. Ong, A. Islam, R. DasGupta, N. G. Iyer, H. L. Leo and Y. C. Toh, Biofabrication, 2017, 9, 045005 CrossRef.
- K. Kim, R. Utoh, K. Ohashi, T. Kikuchi and T. Okano, J. Tissue Eng. Regener. Med., 2017, 11, 2071 CrossRef CAS.
- A. K. Sohlenius-Sternbeck, L. Afzelius, P. Prusis, J. Neelissen, J. Hoogstraate, J. Johansson, E. Floby, A. Bengtsson, O. Gissberg, J. Sternbeck and C. Petersson, Xenobiotica, 2010, 40, 637 CrossRef CAS.
- P. Chao, T. Maguire, E. Novik, K. C. Cheng and M. L. Yarmush, Biochem. Pharmacol., 2009, 78, 625 CrossRef CAS.
- S. Yan, J. Wei, Y. Liu, H. Zhang, J. Chen and X. Li, Acta Biomater., 2015, 28, 138 CrossRef CAS PubMed.
- Y. Liu, L. Zhang, H. Li, S. Yan, J. Yu, J. Weng and X. Li, Langmuir, 2012, 28, 17134 CrossRef CAS PubMed.
- S. I. Jeong, I. D. Jun, M. J. Choi, Y. C. Nho, Y. M. Lee and H. Shin, Macromol. Biosci., 2008, 8, 627 CrossRef CAS.
-
P. O. Seglen, Methods in Cell Biology, ed. D. M. Prescott, Academic Press, Manhattan, New York, USA, 1976, ch. 4, pp. 29–83 Search PubMed.
- Y. Liu, H. Li, S. Yan, J. Wei and X. Li, Biomacromolecules, 2014, 15, 1044 CrossRef CAS.
- S. R. Khetani and S. N. Bhatia, Nat. Biotechnol., 2008, 26, 120 CrossRef CAS.
- Y. Huang, S. L. Zheng, H. Y. Zhu, Z. S. Xu and R. A. Xu, J. Ethnopharmacol., 2014, 151, 583 CrossRef CAS.
- K. J. Livak and T. D. Schmittgen, Methods, 2001, 25, 402 CrossRef CAS PubMed.
|
This journal is © The Royal Society of Chemistry 2020 |
Click here to see how this site uses Cookies. View our privacy policy here.