DOI:
10.1039/C9BM01207A
(Paper)
Biomater. Sci., 2020,
8, 313-324
The fabrication of a highly efficient self-healing hydrogel from natural biopolymers loaded with exosomes for the synergistic promotion of severe wound healing†
Received
1st August 2019
, Accepted 25th October 2019
First published on 29th October 2019
Abstract
Unhealable diabetic wounds and disabling scar formation in severe wounds need to be addressed with the help of multiple techniques. Here we put forward an idea to use exosomes loaded into a supporting scaffold to rebuild the vascular transportation system, which could solve hypoxia and infertility in these wounds. A highly efficient self-healing and biocompatible natural-based methylcellulose-chitosan hydrogel loaded with biological exosome nanoparticles has the appropriate strength and is made by an easy preparation process, and it eventually achieves the integrated structure needed for healing severe diabetic conditions. Hydrogels perform well in the cell proliferation and skin remodeling stages because of their three-dimensional porous structure, and self-healing and adhesion properties. Also, exosomes accumulated to an effective concentration for a period of time could induce proliferation, especially relating to vascular formation. After the overpass (vascular) has been constructed with the help of the base (hydrogel) and workers (exosomes), the society (skin) is reconstructed under a system of supply and regulation. The research results indicate that these novel complex hydrogels loaded with exosomes provide wide prospects for the healing of severe wounds.
Introduction
Diabetes mellitus (DM) is a chronic metabolic disease throughout the world. Delayed wound healing is one common complication of DM, which seriously impairs the life quality of patients, even leading to amputation or death.1–3 The high concentration of glucose in the blood causes vascular occlusion and nerve injury, especially in lower limbs. Therefore, once a small scratch is suffered, it may eventually become a large one which is easily infected and difficult to control.4–7 To reawaken the function of the cells around the wound, the key point is to avoid infection and solve the dysfunction caused by hypoxia and infertility.
Learning from the healing process in a creature's skin, an incrustation should be formed as soon as possible to keep the vulnerable tissue isolated from the exterior. So far, several kinds of artificial barriers and scaffolds, including biocompatible rubber, electrospun nanofibers and functional hydrogels, have been developed to promote wound healing.8–12 Among these biomaterials, self-healing hydrogels, especially those based on naturally derived polysaccharides, such as methylcellulose (MC), chitosan (CS) and their derivatives, are considered to be the most promising wound dressings, because they not only meet the requirements of a barrier, but also act as a multi-functional platform from application, through exertion until retirement.3,13–25 Application: the hydrogel could be easily injected in situ and integrate together quickly, to fill in amorphous wound tissue and prevent pathogen infection. Exertion: The porous structure provides an adhesive spot for neo-tissues. Retirement: The degradation process is propelled as the wound area is gradually filled with neo-genesis tissue. In general, there are three stages of inflammation, proliferation and remodelling in the wound healing process. However, recently investigated hydrogels could play a role only in the first or incomplete second stages for a normal type of wound and could seldom play a role in the final remodelling stage, let alone in serious wounds in diabetic patients.26–28 Thus, to overcome the limited role of biomaterials, a self-healing hydrogel loaded with effective biological factors that promotes healing of severe wounds and restores the original structure and functions is greatly needed.
Recently, mesenchymal stem cells (MSCs) loaded in a scaffold were used to effectively accelerate skin regeneration.21,29–34 Exosomes, a kind of biological nanoparticle secreted by MSCs, are widely involved in communication between cells as a substitute for MSCs. Exosomes show superiority in terms of easier endocytosis and higher loading efficiency based on the nanoscale dimension, compared to cells.34–38 During the healing process, exosomes can transfer their contents to recipient cells, promote the proliferation and migration of fibroblasts and regulate the expression of Type I and III collagen and fibronectin. But their therapeutic effect is greatly impeded because the injected exosomes cannot be enriched at designated positions because of the flow of body fluids. It is necessary to accumulate the exosomes on a scaffold to synergistically promote wound healing.
Herein, a highly efficient injectable self-healing methylcellulose-chitosan hydrogel loaded with exosomes (MC-CS-Exo) was constructed by Schiff base linkage for healing of severe wounds, as depicted in Fig. 1A. The aldehyde modified methylcellulose (MC-CHO) and chitosan grafted poly(ethylene glycol) (CS-g-PEG) were synthesized by DCC and EDC reactions, respectively. The placental mesenchymal stem cell derived exosomes (PMSCs-Exos) were effectively isolated from the human placenta cell culture. The two pre-polymer solutions of MC-CHO and CS-g-PEG in appropriate proportions were mixed with PMSCs-Exos to form a biocompatible complex hydrogel. Owing to the multifunctional features of a self-healing hydrogel scaffold and the induction effect of nanoscale exosomes, the MC-CS-Exo complex hydrogels presented an excellent wound healing function for diabetic mice. This work would provide an effective approach for more efficient complex hydrogels for integrated healing in severe applications.
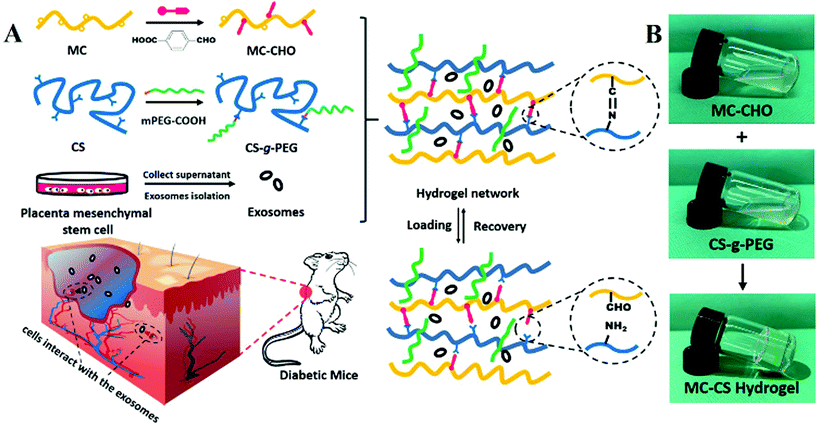 |
| Fig. 1 (A) A schematic illustration of the preparation and application of MC-CS self-healing hydrogels loaded with exosomes. (B) Photographs of the formation process of MC-CS hydrogels through the facile mixing of MC-CHO and CS-g-PEG solutions. | |
Experimental
Materials
Methylcellulose, 4-formylbenzoic acid, dicyclohexylcarbodiimide (DCC), 4-dimethylaminopyridine (DMAP), acetic acid, 1-(3-dimethylaminopropyl)-3-ethylcarbodiimide hydrochloride (EDC·HCl), N-hydroxysuccinimide (NHS), N,N-dimethylformamide (DMF) and ether were purchased from Admas and used without further purification. Chitosan (degree of deacetylation 90%, Mw = 200
000 Da) was purchased from Sinopharm Chemical Reagent Co., Ltd. mPEG-COOH were prepared by DCC reaction of mPEG-OH with succinic anhydride.39 Methylene blue and Congo red were purchased from Sigma-Aldrich and used directly. Dulbecco's modified Eagle's medium (DMEM) was purchased from Gibco. 2,5-Diphenyl-2H-tetrazolium bromide (MTT) and Dio cell membrane green fluorescent probe were purchased from Beyotime Biotechnology Co., Ltd (China). Total exosome isolation reagent (from cell culture media) was purchased from Thermo Fisher. The placental mesenchymal stem cells used in this experiment were obtained from fresh placentas from three normal, full-term (38–40 weeks gestation), healthy donor mothers who were admitted to the Tongji Hospital. The whole experimental process was approved with the written consent of the donor herself and the regulations of the Independent Ethics Committee of the Tongji Hospital affiliated with Tongji University School of Medicine. Human 293T cells were obtained from the cell bank of the Chinese Academy of Science, Shanghai. Experimental animals, 4–6-week-old C57BLKS-Leprdb mice were obtained commercially from the Model Animal Research Centre of Nanjing University (China).
Synthesis of aldehyde methylcellulose (MC-CHO)
MC-CHO was prepared by the DCC reaction of MC and 4-formylbenzoic acid. Briefly, MC (3.40 g, 0.02 mol saccharide) was dissolved in 45 mL of dry DMF. Then 4-formylbenzoic acid (0.19 g, 0.13 mol), DCC (0.25 g, 0.13 mol) and DMAP (0.15 g, 0.065 mol) were added to the above solution. The solution was stirred with a magnetic stirrer in N2 atmosphere at 25 °C for 20 h. After reaction, the mixture was dialyzed (MWCO 8000–14
000) with distilled water for 3 days, and then lyophilized to get the product MC-CHO.
Synthesis of chitosan-g-PEG (CS-g-PEG)
CS-g-PEG was prepared according to a method in the literature with minor modification.40 CS (0.3 g, 2 mmol saccharide) was dissolved in 30 mL of distilled water containing acetic acid (1.46 mL, 21.3 mmol). mPEG-COOH (2.1 g, 1 mmol) was dissolved in the above solution. EDC·HCl (0.2 g, 1 mmol) and NHS (0.12 g, 1 mmol) were dissolved in 20 mL of distilled water and the aqueous solution was added dropwise into the chitosan solution. The mixture was magnetically stirred at 25 °C for 4 h. Then the pH of the solution was adjusted to 7.4 by adding NaOH solution of 0.5 mol L−1. Thereafter, the solution was dialyzed (MWCO 8000–14
000) against distilled water for 3 days with repeated changes of water, followed by drying by vacuum rotary evaporation to obtain pure CS-g-PEG powder.
Preparation of MC-CS hydrogels
The preparation of the MC-CS hydrogels was conducted via Schiff base reactions between aldehyde groups in MC-CHO and the amino groups in the CS chains. PBS solutions of MC-CHO of different concentrations of 4.0, 6.0, 8.0, 10.0 wt% were mixed with a PBS solution of CS-g-PEG (3.0 wt%) at volume ratios of 1.0, respectively. The mixture was mixed uniformly by vortexer and eventually homogeneous hydrogels were obtained after 5–10 min.
Extraction procedure of exosomes from PMSC cell culture medium
The exosomes were extracted from PMSC cell culture. Firstly, PMSC culture was isolated when it reached 80% confluency. Secondly, PMSC culture was filtered with a 0.22 μm sterilized filter (Millipore®) and mixed with Total Exosome Isolation Reagent at a ratio of 1
:
1 and was held at 4 °C overnight. Thirdly, the mixture was centrifuged at 10
000g for 1 h. Afterwards, the concentrated exosomes which were dispersed in PBS solution were transferred into an ultrafiltration tube (Millipore®). Lastly, the exosome@PBS solution was centrifuged at 4000g at 4 °C and stored at −80 °C.
Preparation of MC-CS-Exo hydrogels
The preparation process for the MC-CS-Exo hydrogels was roughly similar to that for MC-CS hydrogels. An exosome@PBS solution with a concentration of 2 × 1012 mL−1 was evenly mixed with MC-CHO solution at a volume ratio of 1
:
10. A PBS solution of CS-g-PEG was added into the above mixture to form a homogeneous hydrogel after 5–10 min.
General characterization techniques
An 1H NMR spectrum of CS-g-PEG was obtained from a Bruker AVANCE-400 MHz NMR spectrometer with DMSO as the solvent. An FT-IR spectrum of MC-CHO was obtained from an EQUINOSS/HYPERRION 2000 spectrometer (Bruker, Germany). SEM images of lyophilized hydrogel were characterized using scanning electron microscopy (SEM) (FEI QUANTA FEG 250). The samples were sprayed with gold before observation. HR-TEM images for the morphology of the exosomes were acquired on a JEOL JEM-2010 TEM. The samples for TEM observation were prepared by placing a drop of 1×PBS suspension on carbon coated copper grids, followed by staining with 1 wt% phosphotungstic acid aqueous solution. Laser-scanning confocal microscope (Leica, Nussloch, Germany) images detected the distribution of Dio-labelled exosomes in the hydrogel. Dynamic light scattering (DLS) showed the hydrodynamic radius (Rh).
Rheological measurements
Rheology tests were conducted at 37 °C on a HAKKE MARS rheometer (Thermo Fisher) with a 25 mm parallel plate set-up and gaps set at 2 mm. A platform was adopted with a silicone oil seal. (1) The storage modulus of the concentration of 4.0, 6.0, 8.0, 10.0 wt% MC-CHO was measured under fixed 1% strain; (2) the MC-CS hydrogel disks (25 mm in diameter, 8.0 wt% MC-CHO and 3.0 wt% CS-g-PEG) were measured under strain amplitude sweep (γ = 0.01%–1000%) at a fixed angular frequency (10 rad s−1); (3) a time-dependent modulus observation at 1% strain decided the time to form the MC-CS hydrogel (25 mm in diameter, 8.0 wt% MC-CHO and 3.0 wt% CS-g-PEG); (4) an alternative step strain sweep of the MC-CS hydrogel disk (25 mm in diameter, 8.0 wt% MC-CHO and 3.0 wt% CS-g-PEG) was measured at a fixed angular frequency (10 rad s−1). Amplitude oscillatory strains were switched from small strain (γ = 1.0%) to subsequent large strain (γ = 500%) with 120 s for every strain interval.
Macroscopic self-healing experiments
Two pieces of MC-CS hydrogel disks were stained with methylene blue and Congo red, respectively. A central hole (6 mm in diameter) was made in the middle of each gel. Then the red round piece was tightly transferred into the blue matrix. The diminishing of the red hole piece and the interface between the red piece and the blue matrix were observed by an optical microscope (DM LP, Leica).
Biocompatibility tests
Extracts of 1 mL MC-CS hydrogel were immersed in 10 mL of DMEM for 24 h at 37 °C in 5% CO2 atmosphere. 293T cells (1 × 104 cells per well) were seeded into 96-well plates and then treated with different ratios of extracts: 25%, 50%, 75%, 100%, respectively. Untreated 293T cells were used as a control. After culturing for 24 h and 48 h, 10 μL of MTT (5 mg mL−1) stock solution was added to each well. After incubation for another 4 h, the resulting formazan crystals formed in the living cells were solubilized with DMSO (100 μL per well). After complete dissolution, the absorbance in each group was recorded with a microplate reader (iMark; Bio-Rad, Hercules, CA, USA) at 490 nm. The cell viability was calculated with eqn (1) |  | (1) |
In vivo wound healing in a full-thickness skin defect model
All the animal experiments were performed according to the criteria of the National Regulation of China for Care and Use of Laboratory Animals and approved by the ethics committee at Tongji University. To evaluate the synergetic healing effect of the multifunctional hydrogel and exosomes, wounds (7 mm in diameter) on the dorsal region of C57BLKS-Leprdb mice with blood glucose concentrations higher than 25 mmol L−1 were applied as models. 60 male C57BLKS-Leprdb mice of 6–8 weeks of age were used for the studies, because mice with the Lepr gene knocked-out suffer from congenital diabetes. All the mice were randomly divided into 4 groups: injecting PBS (PBS), injecting exosomes (E), injecting hydrogel (H) and injecting hydrogel loaded with exosomes (HE). Each group contained 15 mice. All mice were acclimatized for 2 weeks before surgery. All the surgical procedures were performed under aseptic conditions. After a standard anaesthesia procedure with an intraperitoneal injection of 2% pentobarbital (4–4.5 μL per g body weight), the hair on the dorsal region of the mice above the tail but below the back was removed using depilatory cream to prepare for surgery. 7 mm diameter full skin thickness round wounds were created by a needle biopsy. After removing the wound skin, the control group and E group wounds were injected with 100 μL of PBS or exosome@PBS at a concentration of 2 × 1011 mL−1 then dressed with Transparent Film Dressing Frame Style (3 M Health Care, USA), and the H group and HE group wounds were injected with 100 μL of MC-CS hydrogel or MC-CS-Exo hydrogel without dressing with anything. All the tissues were collected on 5 mice in each of the 4 groups on the 5th, 10th, and 15th days. The regeneration process of the wounds was assessed by the decrease in wound area and histomorphological and immunofluorescence determination. For wound area monitoring, on the 5th, 10th, and 15th days, the mice in each group underwent a standard anaesthesia procedure, then the wound areas were recorded with a camera (E-M10, Olympus) and measured by tracing the wound boundaries with Image J software.
Wound area (%) was calculated using eqn (2):
| Wound area (%) = (area (n day))/(area (0 day)) × 100%. | (2) |
Histological and immunofluorescence analysis
For histological analysis, the wound area in five mice from each group was surgically removed, including the surrounding tissue. Then the tissues were fixed in 4% (w/v) PFA for 24 h at 4 °C. Tissue samples were sealed with wax and cut vertically across the skin surface. Samples were stained by hematoxylin & eosin (H&E), Masson's trichrome, anti-human α-SMA and CD 31, respectively, using standard protocols.
Western blotting analysis
The exosomal characteristic markers, including CD 9, CD 63, CD 81 (Abcam) and actin, Bcl-2, Bax, VEGF (Abcam) protein level in the wound area, were analyzed by western blotting.
Statistical analysis
The data from the in vivo wound closure experiment was analyzed using one-way analysis of variance (ANOVA) and Student's t-test. A P value <0.05 was considered to be statistically significant. Results are expressed as the mean ± standard deviation (SD).
Results and discussion
Characterization and in situ formation of hydrogel
The biocompatible self-healing MC-CS hydrogel scaffolds were constructed based on the dynamic covalent Schiff base linkage between two naturally derived polysaccharides. The synthetic procedures for the precursor polymers (MC-CHO and CS-g-PEG) are depicted in Scheme S1 (ESI†). Firstly, aldehyde functionalized methylcellulose (MC-CHO) was successfully prepared via the DCC reaction with the hydroxyl groups in MC and aldehyde in 4-formylbenzoic acid (Scheme S1(a), ESI†). Water-soluble CS-g-PEG was prepared by conjugating PEG chains to the amino of chitosan (Scheme S1(b), ESI†). A Schiff base could form between the aldehyde groups in MC-CHO and the amino groups in CS-g-PEG (Scheme S1(c)†). The structures of MC-CHO (Fig. S1†) and CS-g-PEG (Fig. S2†) were measured by FT-IR and 1H NMR. Considering the solubility of CS-g-PEG in PBS and the cross-linking density of the formed hydrogel networks, the degree of substitution of PEG (DSPEG, defined as the moles of PEG per mole of glucose units) was controlled to 0.25 in this work. Because of the high efficiency between aldehyde groups and amino groups forming a Schiff base in mild conditions, the MC-CS self-healing hydrogels formed rapidly at physiological pH and room temperature after a facile mixing process, as shown in Fig. 1B.
Mechanical properties and self-healing properties of MC-CS hydrogel
The gelation time and mechanical property of the hydrogels were two significant factors for wound dressing, which could be assessed by rheological methods. The storage modulus could reflect the degree of Schiff base formation between amino and aldehyde groups. Fig. 2A(a) shows the storage modulus (G′) of the hydrogels with different concentrations of MC-CHO. The strength increased significantly from 550 to 2320 Pa, when the MC-CHO percentage varied from 4.0 to 10.0 wt%, because of the gradually increasing density of cross-linked networks. An 8.0 wt% of MC-CHO was adopted due to the acceptable fluidity of the solution and the highest mechanical strength, which were regarded as the most complete match between the two constituents. A strain amplitude sweep test of the adopted MC-CS hydrogels (8.0 wt% MC-CHO and 3.0 wt% CS-g-PEG) was performed, as shown in Fig. 2A(b). The platform of the critical strain of both moduli could be maintained up to 100%. However, the hydrogels presented a tendency to break into the liquid phase with greater applied strain. Breakage of the hydrogels happened when the values of the two moduli intersected at an oscillatory strain amplitude of 462%. The gelation time at physiological temperature was determined to be 300 s by the intersection of the two moduli in the dynamic time sweep rheological tests (Fig. 2A(c)).
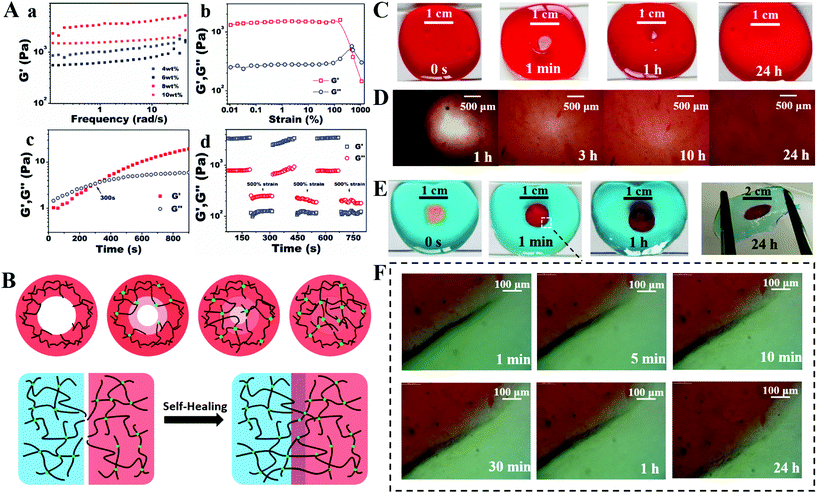 |
| Fig. 2 (A) Rheological experiments with MC-CS hydrogels: (a) G′ values of the hydrogels with different MC content values during the gelation process at 25 °C; (b) strain amplitude sweep test of G′ and G′′ of the hydrogels with 3.0 wt% CS-g-PEG and 8.0 wt% MC-CHO at 25 °C; (c) the gelation time for hydrogels with 3.0 wt% CS-g-PEG and 8.0 wt% MC-CHO at 25 °C; (d) continuous step-strain measurements were applied to the hydrogels in steps of 1% and 500% oscillatory strain for three cycles. (B) An illustration of the mechanism for the self-healing process. (C) Macroscopic self-healing test: the hole in the middle of hydrogel disks coloured with Congo red decreased gradually, until it disappeared at 24 h. (D) Microscopic photographs of the decreasing hole. (E) Macroscopic self-healing test: The hole in the hydrogel disks coloured with methylene blue was filled by the red circle; finally the two connected together to bear a tension force provided by tweezers. (F) Microscopic photographs of the fusion process at the two interfaces. | |
The reversibility of the Schiff base linkage endowed the hydrogel with a self-healing capacity. In order to determine the recovery behaviour, G′ and G′′ of the hydrogels under continuous step changes with 1% and 500% oscillation are shown in Fig. 2A(d). The hydrogel networks were conserved in their initial shapes under low oscillatory strains, while they were broken under a large strain. When the strain oscillation returned to a low value (1%), the two moduli both recovered immediately to their initial values, proving the fast recovery of the hydrogel networks after their destruction. After three circulation times, the recovery G′ of the hydrogels was still equivalent to the primary value, indicating that the hydrogel networks were repeatable with the same efficiency.
The self-healing property was further verified by a macroscopic test. Two dyed hydrogel disks each had a hole (6 mm in diameter) cut in the middle (Fig. 2C and D). When the blue circle hydrogel was placed tightly in the hole of the red hydrogel at room temperature under mild conditions for 24 h, the blue matrix and red circle interpenetrated deeply to form a complete hydrogel disk, and the formed hydrogel was strong enough to bear the tensile force without splitting. Fig. 2D shows that the boundary became unclear when the incubation time increased, and the two kinds of entrapped pigment could diffuse across the interface. As for the 6 mm-diameter hole in the red hydrogel, the size of the hole in the red hydrogel decreased gradually in 1 h and became linked at 3 h. The thickness of the adjacent hole area presented a funnel shape. The hole was filled and levelled up after being placed in moisture for 10 h and was restored to the initial shape after 24 h, which is also shown in the microscope images in Fig. 2F. The assumed mechanism is depicted in Fig. 2B. The polymer chains in the derived polysaccharide which did not consist of the skeleton of the hydrogels could move freely in the hydrogel cages. When the two kinds of functional groups on the different sides of the interface got close enough to interact with each other, a new Schiff base bond came into being without intervention. The two conditions simulated the breakage and even the defects of hydrogels in vitro. The fast linkage in mild conditions qualified it to be an excellent barrier.
Preparation of MC-CS-Exo complex hydrogel
The exosomes were extracted from the culture medium of PMSCs derived from human placenta by multiple centrifugations (Fig. 3A). The western blotting results (Fig. 3B) showed the expression of typical transmembrane proteins of exosomes, such as CD 9, CD 63 and CD 81. The DLS data (Fig. 3C) displayed a well-monodispersed state in PBS and the radius was about 62.5 nm, which generally corresponded with the result from transmission electron microscopy (TEM). All the above results demonstrated that the exosomes were successfully extracted from PMSC cell culture by an easy and efficient procedure. The morphology of the interconnected and hierarchical porous structure is shown in SEM images for the lyophilized hydrogels (Fig. 3D(a) and (b)), which had the capacity to be a scaffold for exosomes. After labelling with Dio, the uniform distribution of exosomes on the skeleton of the hydrogels could be captured by LCSM images (Fig. 3D(d)).
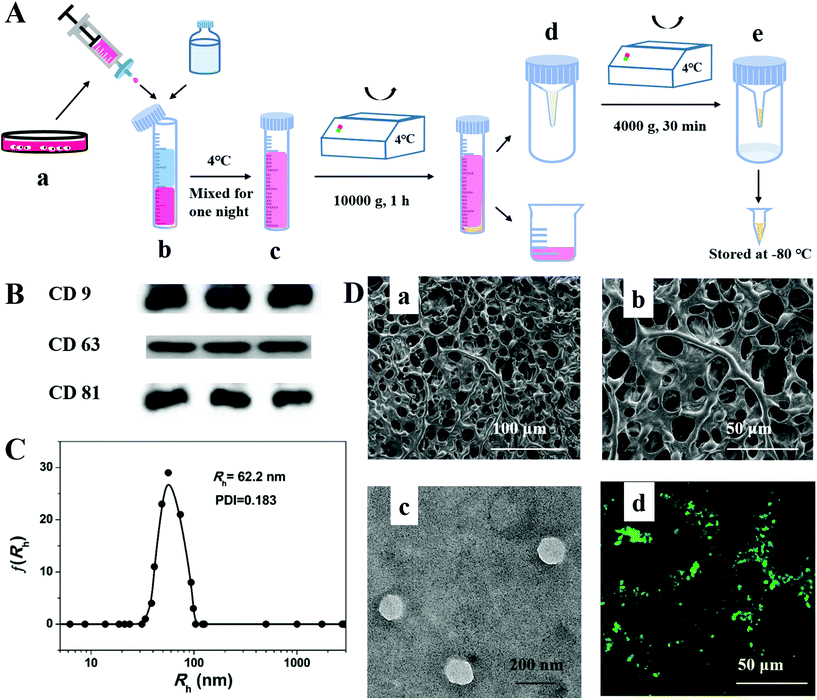 |
| Fig. 3 (A) The process of extracting exosomes from PMSC cell cultures: (a) PMSC culture reaching 80% confluency; (b) mixed PMSC culture filtered with a 0.22 μm sterilized filter (Millipore®) with Total Exosome Isolation Reagent (Thermo Fisher®) at a ratio of 1 : 1; (c) held at 4 °C overnight; (d) mixture centrifuged at 10 000g for 1 h and the exosome PBS solution transferred into an ultrafiltration tube (Millipore®) and the supernatant discarded; (e) centrifuged at 4000g at 4 °C reaching 200 μL and stored at −80 °C. (B) The expression of exosome markers of CD 9, CD 63 and CD 81. (C) The hydrodynamic radius of exosomes measured by dynamic light scattering (DLS). (D) (a) and (b) SEM images of hydrogels at different magnifications; (c) an SEM image of exosomes; (d) a confocal microscopy image of the distribution of exosomes in the hydrogels. | |
In vitro and vivo characterization
The biocompatibility of the hydrogels should be assessed because wound dressing materials are in direct contact with the skin area. An in vitro cytotoxicity test was performed with the help of MTT by using 293T cells cultured for 24 and 48 h with different ratios of hydrogel extract and DMEM. The cell viability (Fig. 4A) in each group remained at more than 90% after 24 h or 48 h of culture. The loss of nutrition may slightly reduce the cell viability in 48 h. The results indicated that the self-healing MC-CS hydrogel network showed no toxicity towards epithelial cells. We suggest that the MC-CS hydrogels could contact tissue directly, acting as an extracellular matrix.
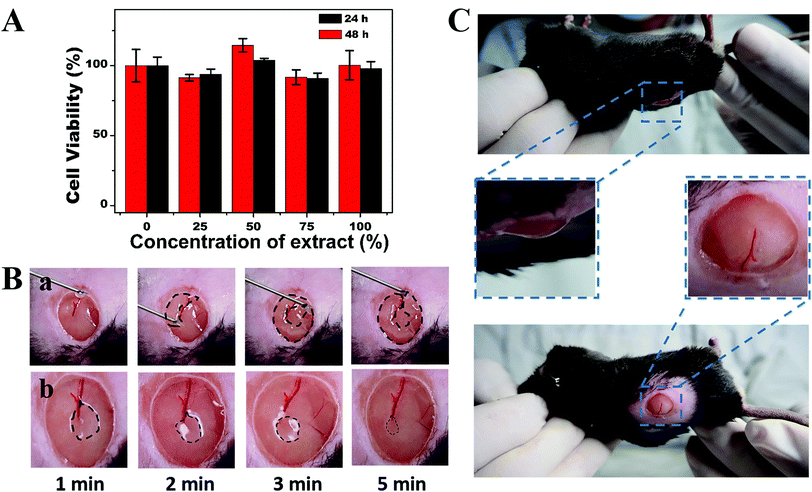 |
| Fig. 4 (A) Cell viability against different concentrations of extract for 24 h and 48 h; all the data shown are more than 92.5%. (B) Injectability performance and self-healing properties in vivo: (a) the hydrogels were injected with a 22-gauge needle into a 7 mm-diameter wound in the dorsal area of a diabetic mouse to form a hydrogel ring (dashed line describes the area). (b) The hole in the hydrogel ring diminished in 5 min in vivo. (C) Adhesive test in vivo. | |
The shear-thinning and self-healing properties provided the hydrogel with the ability to be injected through a narrow needle and allowed complete recovery of the integrated filler. The barrier was able to fill an irregular cavity and remain isolated from bacteria. The injectability and self-healing test of the MC-CS hydrogels were conducted on a 7 mm full-thickness wound mode created in the dorsal area of a mouse under anaesthetic conditions. The pre-formed MC-CS hydrogel was packaged in an injection syringe with a 22-gauge needle, then injected homogeneously and the syringe moved in a circular track at the same time (Fig. 4B). The hydrogel state was monitored by capturing pictures every minute. The photographs (Fig. 4B) showed that the injected hydrogel pieces merged together quickly to form a ring and had the same filling-hole tendency as in the macroscopic experiment. The ring shape was transformed into an integrated disk after 5 min with little trace of collapse. The movement of the subcutaneous tissue accelerated the flexibility of the MC-CS hydrogels, and consequently accelerated the closing rate of the hydrogel hole.
The adhesiveness of the hydrogels is essential for wound closure, since the hydrogels could attach to tissues and stick them together. A hydrogel can be used as an adhesive, hemostat or sealant to make bleeding easy to control. Aldehyde-rich polymers usually serve as tissue adhesives. Moreover, the aldehyde provided by MC-CHO can react with the amino from tissues, which leads to the chemical bond cross-linked adhesion. Thus, the adhesiveness in the in vivo test was applied to the wound area. As shown in Fig. 4C, the hydrogel dressing did not peel off or separate from the wound bed when the mouse was moved conversely or laterally in a gentle way. The adhesiveness could meet the requirements of wound dressing even in a moving area.
Evaluation of in vivo full-thickness wound closure in diabetic rats
As the largest organ in the human body, skin acting as the first barrier, has the ability to prevent pathogens from entering and retains water in the body. In diabetic patients, the ability of proliferation, migration and secretion of related proteins of fibroblasts is weakened due to local tissue ischemia, hypoxia and a high glucose environment, which seriously impair the healing process (inflammation, proliferation and remodelling). Complete recovery means restoring the structure and function of the full-thickness skin, and differentiating hair follicles and sweat glands, as shown in Fig. 5A. The size and morphology of the wound area were recorded by a camera on the 5th, 10th, and 15th days, as representative images over a certain period to reflect the healing process (Fig. 5B). During the 15-day process, it was found in all groups that the wound area contracted as well as the wound bed being propped up with neo-tissue. The body view showed that on the 5th day, a dry and thin scab with a black-brown colour formed on the wound surface of each group, and the surrounding skin became slightly red and swollen. From the 5th day, the wound area and inflammatory response decreased gradually. The PBS group still retained the dry scab and healed slowly. Large area collapse of the newly formed skin tissue was detected in the E group but with newborn black hair. The new tissue level was slightly lower than the normal skin surface and the area presented a shallow colour in H group. While the HE group was the first to show a newborn epidermis and grew surrounding hair on the 15th day. In summary, the groups involving exosome treatment had advantages in the formation of functional organs, while the groups involving hydrogel treatment had advantages in the enrichment in the wound bed. The lesion area and tissue thickness were measured as depicted in Fig. 5C and D. As a result, the wound area shrinkage rate and tissue thickness of the wound area were higher in mice treated with hydrogel-exosomes than in the other three groups. We speculated that these results have the following implications: exosomes stimulate collagen secretion of fibroblasts by inducing epithelial cell proliferation and promoting re-epithelization of severe skin wounds. The hydrogels acted as a filler in the wound area benefiting from their chemical and physical properties, which provided the conditions for granulation growth and enlarged the function of exosomes because of enrichment.
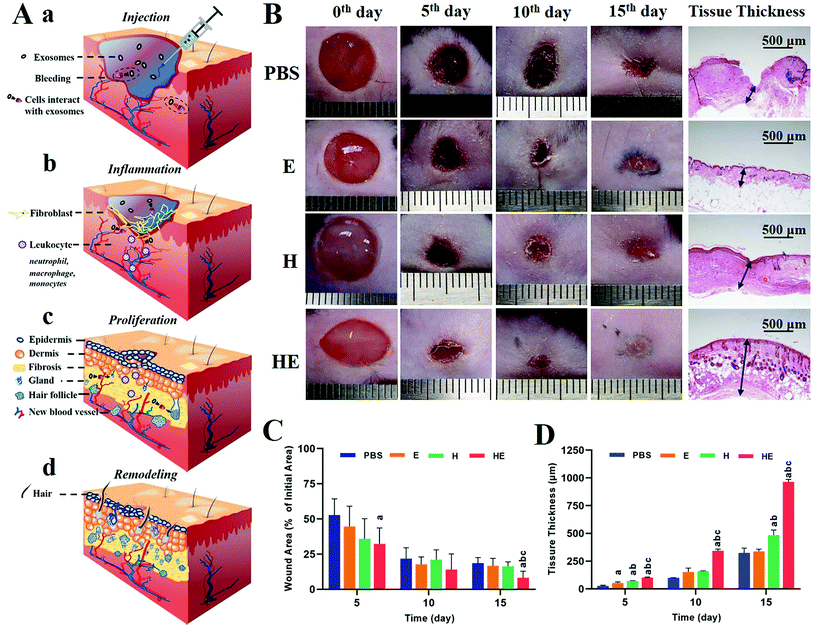 |
| Fig. 5 (A) Schematic illustrations of the wound healing process: (a) injection process; (b) inflammation phase; (c) proliferation phase; (d) remodeling phase. (B) Photographs of wounds on the 0th, 5th, 10th and 15th days. (C) Wound area on the 0th, 5th, 10th and 15th days. (D) Tissue thickness near the wound region on the 0th, 5th, 10th and 15th days. (a) P < 0.05 compared to the PBS group; (b) P < 0.05 compared to H group; (c) P < 0.05 compared to E group. | |
Histomorphological and immunofluorescence evaluation of wound regeneration
To detect the microstructure under the surface, histomorphological determination of wound regeneration was investigated by H&E staining and Masson's staining. The H&E stained section showed the re-epithelization, infiltration of inflammatory cells, fibroblast immigration and connective tissue synthesis in the four groups (Fig. 6a). On the 5th day post-surgery, the infiltration of inflammatory cells in both the PBS group and the H group was enhanced, including macrophages and other monocytes.36 In comparison with the other three groups, the HE group had a thin layer of neo-epidermis, which could correspond to the optical image in Fig. 5B. Interestingly, compared with the other three groups, there was little inflammatory cell infiltration in the HE group and more fibroblasts clustered around the damaged area which was filled with MC-CS-Exo hydrogel. The biocompatible hydrogel composed of chitosan, which had the function of hemostasis and an antibacterial property, may lead to the early activation of the inflammatory phase.18 Besides, on the 10th day, the regularity of the epithelium in the HE and E groups was denser than in the PBS and H groups, with deposition of more fibroblasts and an integrated epithelium structure, which was possibly due to the exosomes loaded in the MC-CS hydrogels promoting cellular movement, including fibroblasts, keratinocytes, nerves and sweat glands. On the 15th day post-surgery, wounds in the HE group showed an exceptional wound healing rate and a better re-epithelialization process, and even a few hair follicles. While the wounds of the PBS group were still filled with a lot of dense fibroblasts, those of the H group seemed to be filled with fibrous tissue.
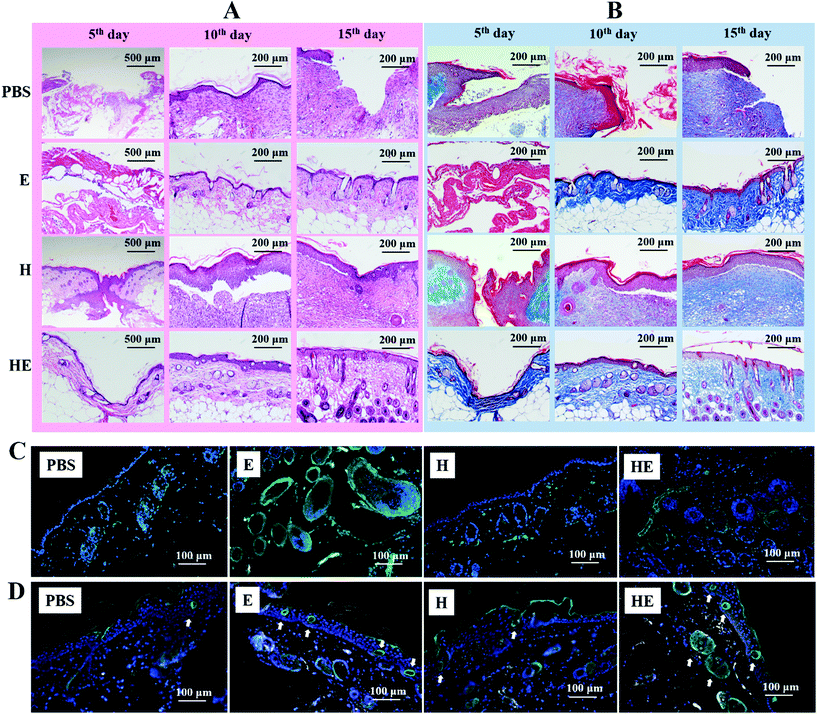 |
| Fig. 6 Representative transmitted light images of (A) H&E and (B) Masson's trichrome stained sections of the untreated defects in four groups on the 0th, 5th, 10th and 15th days. (C and D) Immunofluorescence staining for α-SMA and CD 31. | |
Masson's staining was applied to evaluate collagen production. On the 5th day and 10th day post-surgery, there was a lot of collagen deposition on the wound bed of the HE group in comparison with the other three groups (Fig. 6B). The collagen on the skin wound of the control PBS group was loose and wavy. Meanwhile, the arrangement of collagen was more regular, and the distribution was denser in the HE group. Moreover, on the 15th day post-surgery, there were most neogenetic microvessels between the collagen bundles in the HE group.
Angiogenesis plays an extremely important physiological role, and is closely related to wound repair. The condition of new vessel formation reflects the healing status. After immunofluorescence staining of α-SMA and CD 31, the mature vessels and neogenetic microvessels were labelled with green fluorescence. It was obvious that the tissue in the HE group had the highest density of neogenetic microvessels (labelled with white arrows in Fig. 6D); the E group was second. These vessel makers showed more deposition among the fibroblasts or intertwining against the functional gland in the complex hydrogel group and the exosome loaded hybrid hydrogel group than in the other groups (Fig. 6C and D). The increased distribution of vessels is essential for transporting oxygen, nutrients, immune cells and other substances to the site of injury so as to promote the proliferation of skin related cells and the deposition of an extracellular matrix. These results showed that the PMSC-derived exosomes had positive effects on angiogenesis.
Western blotting analysis of wound regeneration
To further investigate the biological mechanism of the repair process, the expression levels of Bcl-2, Bax and VEGF near the wound area were measured by western blotting (Fig. 7). Bcl-2, which could inhibit cell apoptosis, was up-regulated significantly on the 10th day in the HE group. Protein Bax could accelerate apoptosis. The levels of Bax were down-regulated significantly on the 5th and 10th days in the HE group. VEGF regulates various pathways during the wound healing process, including angiogenesis, re-epithelization and collagen synthesis. The expressions of VEGF in the HE group were significantly increased on the 10th and 15th days. These results revealed that the MC-CS-Exo hydrogel promoted the wound healing process by up-regulating the factor levels, like Bcl-2, Bax and VEGF, so the vessels were constructed as well as the cells proliferating quickly to promote ECM synthesis.
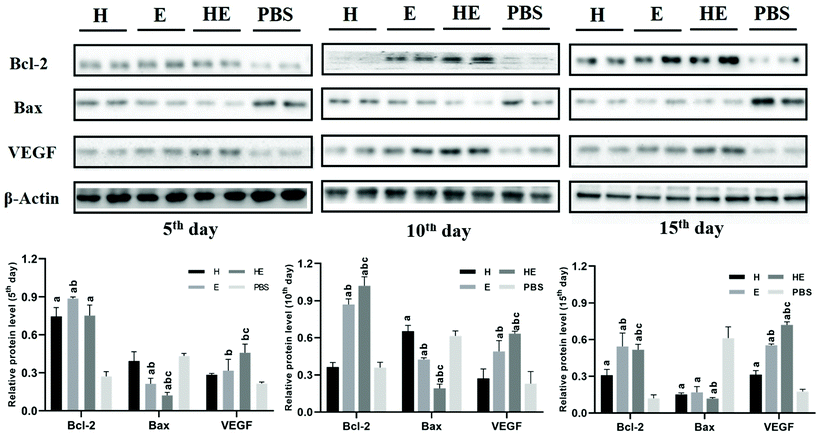 |
| Fig. 7 Expression of wound healing-related proteins on the 5th, 10th and 15th days of the four groups by western blot analysis. β-Actin served as an internal control. The relative protein levels were analyzed, and data are presented as the mean ± SD (n = 3). (a) P < 0.05 compared to the PBS group; (b) P < 0.05 compared to H group; (c) P < 0.05 compared to the E group. | |
Conclusions
Highly efficient self-healing hydrogels loaded with exosomes were successfully constructed from the facile one-pot mixing of MC-CHO, CS-g-PEG and exosomes through the formation of dynamic covalent Schiff base linkages between aldehyde and amino groups. Because of the reversible breaking and linking properties of the Schiff base linkages, the obtained complex hydrogels presented self-healing properties. An appropriate gelation time and mechanical properties and high self-healing efficiency made the hydrogels suitable as injectable wound dressings. Benefitting from the excellent biocompatibility and the self-healing properties of the hydrogels, a combination of biological exosome nanoparticles and hydrogels could synergistically promote skin wound healing to recover the integrated structure and function in a db/db mouse model by promoting angiogenesis and inhibiting apoptosis. The healed area in the HE group was filled with neo-tissues and neo-vessels, and even hair follicles and glands were detected. There was almost no difference compared with normal tissue. The self-healing hydrogels loaded with exosomes significantly improved the effects of the exosomes, and provided a new scaffold with wide prospects for the healing of severe wounds.
Ethics approval and consent to participate
All experiments were performed in accordance with the Guidelines of National Regulation of China for Care and Use of Laboratory Animals, and experiments were approved by the ethics committee at Tongji University.
Conflicts of interest
There are no conflicts to declare.
Acknowledgements
The authors are thankful for financial support from the National Natural Science Foundation of China (no. 81771942 and 81570738) and the Natural Science Foundation of Shanghai (no. 18ZR1433700).
Notes and references
- S. L. Wong, M. Demers, K. Martinod, M. Gallant, Y. Wang, A. B. Goldfine, C. R. Kahn and D. D. Wagner, Nat. Med., 2015, 21, 815 CrossRef CAS.
- L. I. F. Moura, A. M. A. Dias, E. Carvalho and H. C. de Sousa, Acta Biomater., 2013, 9, 7093–7114 CrossRef CAS.
- M. D. Konieczynska, J. C. Villa-Camacho, C. Ghobril, M. Perez-Viloria, W. A. Blessing, A. Nazarian, E. K. Rodriguez and M. W. Grinstaff, Mater. Horiz., 2017, 4, 222–227 RSC.
- M. Edmonds, J. L. Lázaro-Martínez, J. M. Alfayate-García, J. Martini, J.-M. Petit, G. Rayman, R. Lobmann, L. Uccioli, A. Sauvadet, S. Bohbot, J.-C. Kerihuel and A. Piaggesi, Lancet Diabetes Endocrinol., 2018, 6, 186–196 CrossRef CAS.
- D. G. Armstrong, A. J. M. Boulton and S. A. Bus, N. Engl. J. Med., 2017, 376, 2367–2375 CrossRef.
- A. Singh, R. Bhattacharya, A. Shakeel, A. K. Sharma, S. Jeevanandham, A. Kumar, S. Chattopadhyay, H. B. Bohidar, S. Ghosh, S. Chakrabarti, S. K. Rajput and M. Mukherjee, Mater. Horiz., 2019, 6, 274–284 RSC.
- J. S. Choi, K. W. Leong and H. S. Yoo, Biomaterials, 2008, 29, 587–596 CrossRef CAS.
- Y. Liang, X. Zhao, T. Hu, B. Chen, Z. Yin, P. X. Ma and B. Guo, Small, 2019, 15, 1900046 CrossRef.
- J. Qu, X. Zhao, Y. Liang, Y. Xu, P. X. Ma and B. Guo, Chem. Eng. J., 2019, 362, 548–560 CrossRef CAS.
- R. Xu, G. Luo, H. Xia, W. He, J. Zhao, B. Liu, J. Tan, J. Zhou, D. Liu, Y. Wang, Z. Yao, R. Zhan, S. Yang and J. Wu, Biomaterials, 2015, 40, 1–11 CrossRef CAS.
- F. Ding, H. Deng, Y. Du, X. Shi and Q. Wang, Nanoscale, 2014, 6, 9477–9493 RSC.
- A. Seth, Y. G. Chung, E. S. Gil, D. Tu, D. Franck, D. Di Vizio, R. M. Adam, D. L. Kaplan, C. R. Estrada, Jr. and J. R. Mauney, Biomaterials, 2013, 34, 4758–4765 CrossRef CAS.
- Y. Xi, J. Ge, Y. Guo, B. Lei and P. Ma, ACS Nano, 2018, 12, 10772–10784 CrossRef CAS.
- L. Zhou, Y. Xi, Y. Xue, M. Wang, Y. Liu, Y. Guo and B. Lei, Adv. Funct. Mater., 2019, 1806883 CrossRef.
- M. Li, J. Chen, M. Shi, H. Zhang, P. X. Ma and B. Guo, Chem. Eng. J., 2019, 375, 121999 CrossRef CAS.
- C. Wang, M. Wang, T. Xu, X. Zhang, C. Lin, W. Gao, H. Xu, B. Lei and C. Mao, Theranostics, 2019, 9, 65 CrossRef CAS.
- Y. Liang, X. Zhao, T. Hu, Y. Han and B. Guo, J. Colloid Interface Sci., 2019, 556, 514–528 CrossRef CAS.
- X. Zhao, H. Wu, B. Guo, R. Dong, Y. Qiu and P. X. Ma, Biomaterials, 2017, 122, 34–47 CrossRef CAS.
- X. Zhao, P. Li, B. Guo and P. X. Ma, Acta Biomater., 2015, 26, 236–248 CrossRef CAS.
- D. R. Griffin, W. M. Weaver, P. O. Scumpia, D. Di Carlo and T. Segura, Nat. Mater., 2015, 14, 737–744 CrossRef CAS.
- V. Miguez-Pacheco, L. L. Hench and A. R. Boccaccini, Acta Biomater., 2015, 13, 1–15 CrossRef CAS PubMed.
- X. Yang, G. Liu, L. Peng, J. Guo, L. Tao, J. Yuan, C. Chang, Y. Wei and L. Zhang, Adv. Funct. Mater., 2017, 27, 1703174 CrossRef.
- J. Qu, X. Zhao, Y. Liang, T. Zhang, P. X. Ma and B. Guo, Biomaterials, 2018, 183, 185–199 CrossRef CAS.
- V. Yesilyurt, M. J. Webber, E. A. Appel, C. Godwin, R. Langer and D. G. Anderson, Adv. Mater., 2016, 28, 86–91 CrossRef CAS.
- S. Azevedo, A. M. S. Costa, A. Andersen, I. S. Choi, H. Birkedal and J. F. Mano, Adv. Mater., 2017, 29, 1700759 CrossRef.
- O. Soehnlein, S. Steffens, A. Hidalgo and C. Weber, Nat. Rev. Immunol., 2017, 17, 248–261 CrossRef CAS.
- C. S. Klose and D. Artis, Nat. Immunol., 2016, 17, 765–774 CrossRef CAS.
- W. C. Gause, T. A. Wynn and J. E. Allen, Nat. Rev. Immunol., 2013, 13, 607–614 CrossRef CAS.
- S. Barrientos, O. Stojadinovic, M. S. Golinko, H. Brem and M. Tomic-Canic, Wound Repair Regen., 2008, 16, 585–601 CrossRef.
- G. S. Schultz and A. Wysocki, Wound Repair Regen., 2009, 17, 153–162 CrossRef.
- M. A. M. Jahromi, P. S. Zangabad, S. M. M. Basri, K. S. Zangabad, A. Ghamarypour, A. R. Aref, M. Karimi and M. R. Hamblin, Adv. Drug Delivery Rev., 2018, 123, 33–64 CrossRef.
- T. Burnouf, D. Strunk, M. B. Koh and K. Schallmoser, Biomaterials, 2016, 76, 371–387 CrossRef CAS.
- W. Wang, C. Yang, X. Y. Wang, L. Y. Zhou, G. j. Lao, D. Liu, C. Wang, M. D. Hu, T. T. Zeng, L. Yan and M. Ren, Diabetes, 2018, 67, 1627–1638 CrossRef CAS PubMed.
- Y. Hu, S. S. Rao, Z. X. Wang, J. Cao, Y. J. Tan, J. Luo, H. M. Li, W. S. Zhang, C. Y. Chen and H. Xie, Theranostics, 2018, 8, 169–184 CrossRef CAS.
- X. Liu, Y. Yang, Y. Li, X. Niu, B. Zhao, Y. Wang, C. Bao, Z. Xie, Q. Lin and L. Zhu, Nanoscale, 2017, 9, 4430–4438 RSC.
- M. Li, Q.-F. Ke, S.-C. Tao, S.-C. Guo, B.-Y. Rui and Y.-P. Guo, J. Mater. Chem. B, 2016, 4, 6830–6841 RSC.
- N. Xu, L. Wang, J. Guan, C. Tang, N. He, W. Zhang and S. Fu, Int. J. Biol. Macromol., 2018, 117, 102–107 CrossRef CAS.
- P. Kong, X. Xie, F. Li, Y. Liu and Y. Lu, Biochem. Biophys. Res. Commun., 2013, 438, 410–419 CrossRef CAS.
- W. Yuan, F. Zhang, X. Xie and C. Pan, Macromolecules, 2007, 40, 9094–9102 CrossRef CAS.
- C. Tsao, M. Hsiao, M. Zhang, S. Levengood and M. Zhang, Macromol. Rapid Commun., 2015, 36, 332–338 CrossRef CAS.
Footnote |
† Electronic supplementary information (ESI) available: A detailed Experimental section and FT-IR and 1H NMR spectra of materials. See DOI: 10.1039/c9bm01207a |
|
This journal is © The Royal Society of Chemistry 2020 |
Click here to see how this site uses Cookies. View our privacy policy here.