DOI:
10.1039/D0AY90112A
(AMC Technical Brief)
Anal. Methods, 2020,
12, 4496-4500
X-ray micro computed tomography in cultural heritage
Received
11th August 2020
First published on 16th September 2020
Abstract
X-ray micro computed tomography (μCT) can reveal the internal structure of several types of objects by exploiting differences in their X-ray absorption. It can: provide information about the objects’ relative composition and density without taking any samples or causing any damage; reveal invisible defects, cracks or damage; show if there are any inclusions and if the object is a single piece or is made of different parts joined together; and, uncover methods of construction and even show if repairs and additions have been made. For this reason, it has increasingly been used in archaeology and cultural heritage.
Accessing an object’s internal information has been an ongoing problem in many disciplines. Historically, while a number of fields of science could afford serial slicing of specimens to investigate their inner structure (e.g. biology, geology, materials science and occasionally palaeontology), this was not always possible for unique or rare objects or fossils; it was certainly problematic when diagnosing a patient! The discovery of X-rays in 1895 made it possible to see through matter non-invasively. Initially only 2D images (also called radiographs) could be generated, but in the late 1960s the development of computer-assisted tomography (CAT), also known as computed tomography (CT), made it possible to perform three-dimensional X-ray imaging. The first CT scanners were dedicated to medicine thereby protecting patients by limiting X-ray exposure. In the 1980s, micro-CT (μCT) was developed for biomedical research with higher spatial resolution (from a few millimetres to a few nanometres), contrast resolution and higher X-ray flux, to investigate objects where the radiation dose was less of an issue. Today, laboratory X-ray CT equipment is widely available and allows the characterisation of all sorts of objects in all science fields.
Procedure
All CT devices share a common procedure: a series of radiographs are taken during a 360° rotation of the object. As the object has to be completely still during the rotation, medical CT devices rotate the X-ray source and the detector around the patient who lies on a table. Conversely, for most laboratory devices the X-ray source and the detector are stationary, and the object is rotated on a turntable (Fig. 1).
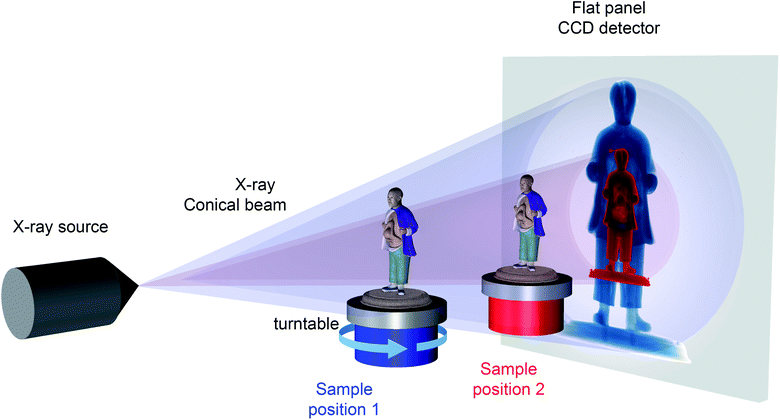 |
| Fig. 1 Diagram of a common laboratory X-ray micro-CT setup. The object is placed in the X-ray beam, attenuating it depending on its composition, density and size. The projected radiograph is recorded by the CCD flat panel detector. Depending on the position of the sample, the projected image is more or less magnified: in position 2, the sample is far from the source, projecting a small image on the panel (red image); in position 1, the sample is closer, projecting a larger image on the panel (in blue). © Natural History Museum and Royal College of Surgeons. | |
After recording thousands of images of the rotating object, a virtual volume composed of many ‘slices’ is reconstructed thereby mapping the density of the material in the volume of the object exposed to the X-rays. With this series of digital cross sections it is possible to isolate and visualise parts showing different densities but also, with more time and effort, to separate manually and therefore differentiate areas of similar density.
Software handling 3D datasets can separate parts in the volume by rendering them in different shades of grey (the whitest parts being the densest material in an object), and each separated part can be artificially coloured individually to help visualise the different materials and structural features.
Technical details
A laboratory X-ray source consists of an electron beam hitting a metallic target typically made of tungsten. A small opening in the source channels the X-rays out creating a conical beam. On the other side of the cabinet containing the equipment is a detector. Most detectors do not detect X-rays directly. Instead, a layer called a scintillator is used to convert X-rays to visible light (the material of the scintillator absorbs X-rays and emits visible light by fluorescence) which is then detected with a charge-coupled device (CCD) or complementary metal oxide semiconductor (CMOS) camera (Fig. 1). Because of the conical shape of the X-ray beam, the magnification in the recorded image will depend on the position of the sample between the source and the detector: the closer the object is to the source, the larger the projected image, and the finer the details revealed (e.g. positions 1 and 2 in Fig. 1).
Amongst the different interactions of X-rays with matter, the one measured here is absorption. Following a phenomenon described by the Beer–Lambert law, a single projection (or radiograph) shows the intensity of the transmitted signal. As X-rays interact with the electrons orbiting around an atom’s nucleus, the more electrons there are the more X-rays are absorbed. This can be seen when atoms are packed closer together (increasing density) or when the material is made of heavier elements (hence containing more electrons). This explains, for example, why radiographs of bone (largely composed of calcium and phosphorus) appear more opaque than surrounding muscles which are made of lighter elements such as hydrogen, carbon, nitrogen and oxygen.
What is X-ray μCT good for?
For an object to be successfully analysed by X-ray μCT, three conditions have to be fulfilled:
(1) The object has to fit inside the CT chamber.
(2) The object must also fit within the scanning envelope (i.e. the volume that can be exposed to X-rays and that can be seen by the detector within a given setup). If this is not the case, different portions of the object will need to be scanned separately and the results recombined later.
(3) The machine must be able to generate X-rays with enough energy to go through the densest part of the object. A cultural heritage object can be made of a mixture of materials, each with different attenuation coefficients, i.e. each reducing the X-ray intensity by differing amounts. For example, a clay figurine can have metallic wires inside, a wooden box may have metallic hinges, nails or locks. If the metallic part is too thick or too dense it can block the X-ray beam entirely making imaging impossible.
X-ray μCT works best when the object is not too dense and there is some density contrast within it. It will easily capture voids in organic materials. Paint with metallic content covering organic materials will be visible as well. The technique is sensitive enough to show growth rings in wood, which is helpful for distinguishing the different pieces making up an object. In heritage science the success of the technique will depend on the object of interest and the aim of the investigation: characterising porosity or cracks in a material is often successful, but isolating different parts might not work at all if they have similar composition.
Advantages of X-ray μCT
• X-ray μCT provides volumetric and density information about an object non-invasively.
• The object can be manipulated virtually without any risk of damage: the object can be virtually rotated, dissected, measured, disassembled or reassembled, etc.
• The technique is relatively easy to access. Beside its widespread availability in hospitals and private industries, the number of academic and museum laboratories equipped with such devices is growing.
• For museum objects, the technique provides a digital copy that can be shared across the world, facilitating dissemination and reducing potential damage by offering an alternative to the physical handling of the object.
Limitations of X-ray μCT
• X-ray μCT can be time-consuming. Data acquisition is relatively fast, from a few minutes to several days in extreme cases. If a specimen is large and/or dense, it attenuates X-rays more, therefore to get a good signal the exposure time is increased. However, the image processing and the separation of the subsets of the volume to represent the different components of the object is a time-consuming process that can take weeks, but the quality and relevance of the resulting information make this task worth the time and effort.
• The data generated is often several gigabytes in size, making data storage and handling resource- and computationally-intensive.
• The size of the object can be a problem. Firstly, most facilities with X-ray μCT systems will be able to investigate objects from a few millimetres to a few tens of centimetres; however, facilities suitable for the investigation of larger objects are rare. Secondly, the size limit is dependent on the composition of the object. For example, with a machine working up to 225 kilovolts (which is a common setup) one will be able to investigate, inter alia, a few tens of centimetres of wood, 10–20 cm for most fossils, ∼5 cm for rocks rich in metals or a couple of centimetres of stainless steel. Finding a facility with more energetic X-ray sources to extend the range and dimensions of the objects that can be analysed is possible but certainly less common.
• It is a relatively but not prohibitively expensive technique: looking at the full economic costing alone (i.e. considering only the cost of running the equipment) a good ball-park figure is £500–1000 for a full day of usage.
Case study 1 – clay model of a Chinese man
A clay model from the museum collection of the Royal College of Surgeons, due to undergo conservation treatment, depicts a man with a conjoined twin attached to his chest and shows visible damage, mostly on the back of the head. Using X-ray μCT, it was possible to understand how this model was constructed and if there had been any previous intervention. The analysis revealed the texture of the unfired clay and detected invisible inner cracks and air bubbles. The imaging also showed that different parts of the model (legs, head, parasitic twin) had been sculpted individually and then attached to the torso (Fig. 2c). Furthermore, the model does not have a metallic armature inside, but three pieces of organic material (probably wood) in the legs and the head (Fig. 2b). The paint on the cassock and face also stands out in the data indicating the metallic content (which was confirmed separately by other techniques). The blue paint on the cassock does not show any contrast with the clay. Identified as ultramarine blue by Raman microscopy, the blue pigment has a density too close to that of the clay (both in terms of actual composition and mass/volume), which translates to similar grey levels in the data. Because of this lack of contrast the software could not differentiate between the two different materials. It is however possible to select manually just the first few voxels (voxels are the 3D equivalent of 2D pixels) at the surface of the model, attribute them to the coat, and paint them virtually in blue (for more information, see ref. 1).
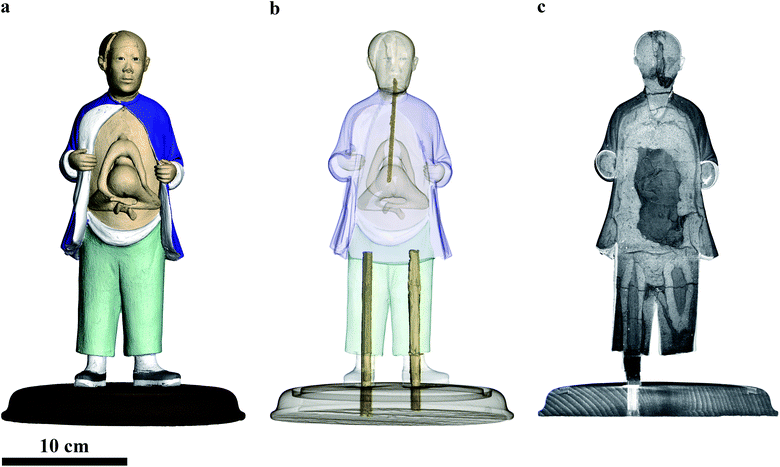 |
| Fig. 2 Digital 3D rendering of the figurine. (a) Artificially coloured rendering; (b), semi-transparent figurine revealing the organic material supporting the legs and head; (c), vertical section through the dataset showing the different parts of the model. © Natural History Museum and Royal College of Surgeons. | |
Case study 2 – Barniz de Pasto table cabinet
The lid from a rare 17th-century table cabinet from South America, from the Victoria and Albert Museum collections (Fig. 3), was analysed by X-ray μCT to visualise the original decoration hidden under a 20th-century paint scheme. The analysis facilitated visually ‘peeling off’ the modern surface paint to reveal the original pigments. The latter contained mostly mercury and were therefore easily distinguishable from the wooden substrate and the other organic components of the paint (for more images see ref. 2). See ref. 3–5 for further examples of where X-ray μCT has successfully been used in heritage science.
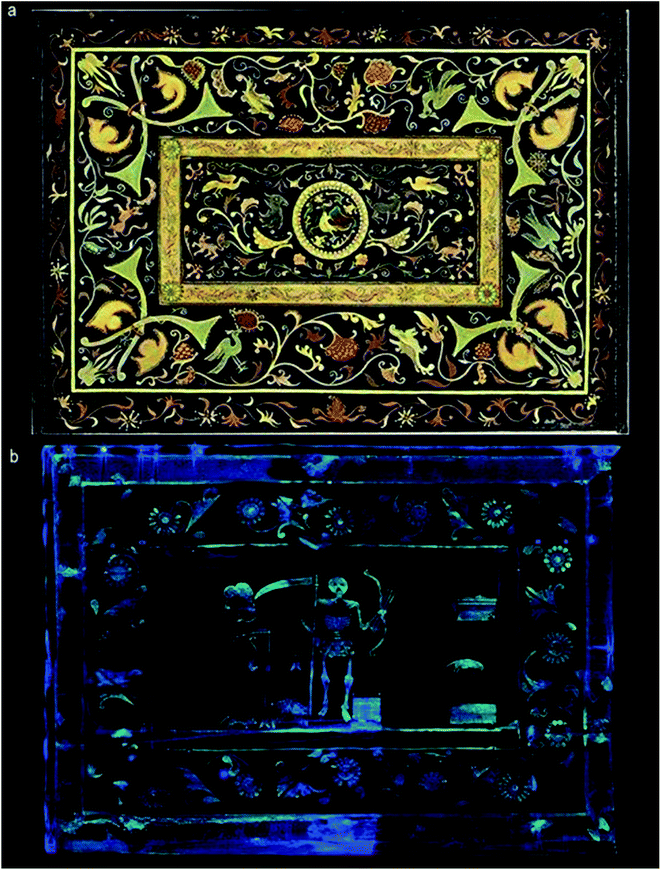 |
| Fig. 3 (a) Photograph of the inner surface of the V&A cabinet’s lid showing a 20th century paint scheme; (b) digital rendering of the original mercury-based decorative scheme, hidden below the surface. © Victoria and Albert Museum and Natural History Museum. | |
Acknowledgements
The case studies presented involved investigators from several institutes: Sebastian Foxley (Royal College of Surgeons), Lucia Burgio (the Victoria and Albert Museum), Brett Clark and Amin Garbout (Natural History Museum).
Dr Vincent Fernandez (Natural History Museum)
This Technical Brief was prepared for the Analytical Methods Committee (AMC), with contributions from members of the AMC Heritage Science Expert Working Group, and approved by the AMC on 7 July 2020.
Further Reading
- S. Foxley, Treasures from the collections, RCS Bulletin, 2019, 101(5), 206 Search PubMed.
-
L. Burgio, Hidden surprises, V&A Blog, 2018, https://www.vam.ac.uk/blog/caring-for-our-collections/hidden-surprises.
- F. Albertin, M. Bettuzzi, R. Brancaccio, M. Pia Morigi and F. Casali, X-ray computed tomography in situ: an opportunity for museums and restoration laboratories, Heritage, 2019, 2(3), 2028–2038 CrossRef.
- A. Re, F. Albertin and L. Zamprotta, X-ray tomography of large wooden artworks: the case study of “Doppio corpo” by Pietro Piffetti, Heritage Sci., 2014, 2(1), 19 CrossRef.
- A. Masson-Berghoff and D. O’Flynn, Absent, invisible or revealed ‘relics’? X-radiography and CT scanning of Egyptian bronze votive boxes from Naukratis and elsewhere, British Museum Studies in Ancient Egypt and Sudan, 2019, 24, 159–174 Search PubMed.
|
This journal is © The Royal Society of Chemistry 2020 |