DOI:
10.1039/C9AN01946D
(Paper)
Analyst, 2020,
145, 70-75
A cobalt corrole/carbon nanotube enables simultaneous electrochemical monitoring of oxygen and ascorbic acid in the rat brain
Received
30th September 2019
, Accepted 17th October 2019
First published on 22nd October 2019
Abstract
It is of interest to in vivo monitor the co-dynamics of different substances. However, the tracking of multiple species is still challenging. In this work, we demonstrate an in vivo electrochemical method by using multi-potential step amperometry to in vivo detect ascorbic acid (AA) and oxygen (O2) simultaneously. In order to achieve good selectivity and high sensitivity for both AA and O2, we design a cobalt corrole [Co(tpfc)(py)2] (tpfc = 5,10,15-tris(penta-fluorophenyl) corrole, py = pyridine, denoted as Co-TPFC) and carbon nanotube nanocomposite to modify a carbon fiber microelectrode (Co-TPFC/MWNT/CFE). This Co-TPFC/MWNT/CFE exhibits excellent electrocatalytic properties towards the reduction of O2 preceding a 4e process and facilitates the oxidation of AA at low potential in the physiological environment. Based on this, we realize simultaneous detection of AA and O2 using two-potential steps (one cathodic (−0.2 V) and the other anodic (+0.05 V)) with 1 second step time. Both in vitro and in vivo experiments proved the feasibility of this method. This demonstrated strategy is useful for us to understand various physiological and pathological processes associated with O2 and AA co-dynamics, and also provides an idea for detecting multiple substances simultaneously.
In vivo electrochemistry is playing an ever increasing role in both chemistry and neuroscience communities since it offers a high spatial and temporal resolution to track the dynamics of neurochemicals.1–5 Lots of strategies have been developed for monitoring single neurochemicals such as dopamine (DA),6–8 AA,9–15 O2,16 H2O2,17 H2S,18,19etc. in vivo. Some methods have also been reported for measurements of two or more component substances of brain tissue in vivo. For instance, differential pulse voltammetry (DPV) has been used for the simultaneous detection of multiple neurochemicals in vivo such as DA, DOPAC and 5-HT.20,21 Wightman et al. used fast scan cyclic voltammetry (FSCV) for simultaneous in vivo recording of the transient change of oxygen and dopamine during an electrical stimulus.22,23 An electrode array is also often used to record multiple neurochemicals.24–26 However, in vivo detection of multiple species is still a challenge because microelectrodes should have high selectivity and high space resolution without considering the overlap of the neighboring microelectrode.27–29
As we know, O2 is vital to our body, and the brain as one of the most oxygen consuming organs is sensitive to it. The deficiency of O2 can be the cause of many pathophysiological conditions, such as ischemia, hypoxia etc.30–33 AA is one of the most abundant neuroprotective small-molecule antioxidants and free radical scavengers endogenously which provide the first line of defense against oxidative damage in the central nervous system.34 O2 and AA are involved in many physiological and pathological processes together.35–37 Thus, exploring the co-dynamic change of O2 and AA can help us understand those processes better. Although carbon fibers and carbon paste microelectrodes have been successfully used to probe O2, they still suffer from the production of neurotoxic H2O2 during in vivo O2 detection.38–41 Although a Pt nanoparticle modified microelectrode could detect O2 with high sensitivity without the production of H2O2, it could not facilitate the oxidation of AA.16 AA has a large oxidation overpotential (ca. 300 mV) at the normal electrode, which makes the selective electrochemical measurements of O2 and AA in vivo a challenge.9
In this work, we developed a method for the simultaneous detection of O2 and AA in the rat brain using a cobalt corrole [Co(tpfc)(py)2] (tpfc = 5,10,15-tris(penta-fluorophenyl) corrole, py = pyridine, denoted as Co-TPFC) and carbon nanotube nanocomposite modified carbon fiber electrode (Co-TPFC/MWNT/CFE). Recently, we found that Co-TPFC can adsorb onto the carbon nanotube and the formed Co-TPFC/MWNT nanocomposite shows outstanding electrocatalytic properties towards the reduction of O2 preceding a 4e process in acid media, and the carbon nanotube could greatly facilitate the oxidation of ascorbate at a low potential (ca. −50 mV).9,42 In this manuscript, we explore the feasibility of Co-TPFC/MWNT/CFE to monitor O2 and AA in vivo. The result shows that the Co-TPFC and carbon nanotube nanocomposite exhibits electrocatalytic properties towards the reduction of O2 preceding a 4e process and facilitate the oxidation of AA at a low potential in a physiological solution. Based on this, we use multi-potential step amperometry by simply applying two-potential steps with 1-second step time each (one cathodic (−0.2 V) and the other anodic (+0.05 V)) and successfully detect O2 and AA simultaneously. This Co-TPFC/MWNT/CFE provides a new platform for in vivo measurements of the co-dynamic change of O2 and AA and offers a new idea for probing multiple neurochemicals.
Experimental section
Reagents and solutions
Dopamine (DA), ascorbic acid (AA), uric acid (UA), 3,4-dihydroxyphenylacetic acid (DOPAC), 5-hydroxytryptamine (5-HT), and hydrogen peroxide (H2O2) were all purchased from Sigma and used as supplied, and the solutions were prepared just before use. Artificial cerebrospinal fluid (aCSF) was prepared by mixing NaCl (126 mM), KCl (2.4 mM), KH2PO4 (0.5 mM), MgCl2 (0.85 mM), NaHCO3 (27.5 mM), Na2SO4 (0.5 mM), and CaCl2 (1.1 mM) into Milli-Q water, and the pH was adjusted to 7.4. Other chemicals were of at least analytical reagent grade and used without further purification. All aqueous solutions were prepared with Milli-Q water. Unless otherwise pointed out, all experiments were carried out at room temperature.
Preparation of the Co-TPFC/MWNT nanocomposite
Co-TPFC was synthesized as described in the literature.43 Multi-walled carbon nanotubes (MWNTs, 40–60 nm in diameter and <2 μm in length) were purchased from Shenzhen Nanotech Port Co., Ltd (Shenzhen, China), and the MWNT was purified by refluxing the as-received MWNT in 2.6 M nitric acid for 5 h, followed by centrifugation, resuspension and filtration, and dried to evaporate the solvent. To prepare the Co-TPFC/MWNT nanocomposite, 3 mg of synthetic Co-TPFC in acetonitrile was added into 15 mg of MWNT in 3 ml of acetonitrile solvent under stirring conditions. The resultant mixture was then stirred for 12 h and then ultrasonicated for 1 h to obtain a homogeneous dispersion before use.
Preparation and modification of the carbon fiber microelectrodes
The carbon fiber microelectrodes (CFEs) were fabricated as described previously.14 One drop of the Co-TPFC/MWNT dispersion was applied onto a smooth glassy plate and the CFEs were immersed and rolled into the droplet under a microscope. Attention should be paid not to break the carbon fibers while immersing and rolling the CFEs into the Co-TPFC/MWNT droplet. The electrodes (denoted as Co-TPFC/MWNT/CFEs) were then taken out from the droplet, and air-dried to evaporate the solvent.
Apparatus and measurements
Electrochemical measurements were performed with a computer-controlled electrochemical analyzer (CHI 760d, CHI Instrument). The Co-TPFC/MWNT-CFEs were used as the working electrode and a platinum wire as the counter electrode. In order to meet the requirement of in vivo measurements, we prepare a micro-sized Ag/AgCl electrode as a reference electrode that can be implanted into the brain tissue by first polarizing an Ag wire (i.d. 1 mm) at +0.6 V in 0.1 M hydrochloride acid for ca. 30 min to make an Ag/AgCl wire and then inserting the Ag/AgCl wire into a pulled glass capillary, in which aCSF was sucked from the fine end of the capillary and used as the inner solution. The larger open end of the capillary was finally sealed with epoxy using the method mentioned above. To avoid the potential difference in in vivo and in vitro electrochemical measurements, the prepared micro-sized Ag/AgCl electrode was used in all electrochemical measurements.
In vivo experiments
Adult male Sprague–Dawley rats (300–350 g) were purchased from the Health Science Center, Peking University. The animals were housed under a 12
:
12 h light–dark schedule with food and water ad libitum. All animal procedures were performed in accordance with the Guidelines for Care and Use of Laboratory Animals of National Center for Nanoscience and Technology of China and approved by the Animal Ethics Committee of National Center for Nanoscience and Technology of China. Animal experiments were performed using a method described in our earlier work.8,14 Briefly, the animals were anaesthetized with chloral hydrate (345 mg kg−1, i.p.) and positioned onto a stereotaxic frame. The Co-TPFC/MWNT/CFE was implanted into the hippocampus (AP = 2.5 mm, L = 4.4 mm from bregma, V = 2.2 mm from dura) using standard stereotaxic procedures. The prepared micro-sized Ag/AgCl reference electrode was implanted from the dura of the brain close to the working electrode and secured with tooth cement. The steel wire inserted into the brain muscle was used as the counter electrode. The surgical procedures of two-vessel occlusion (2-VO) ischemia were performed during the period of in vivo measurements. The 2-VO ischemia model was constructed by ligation of the bilateral common carotid arteries (CCAs) with a 3–0 suture to induce permanent forebrain ischemia, with the methods reported previously.
Results and discussion
Electrochemical properties of the co-TPFC/MWNT/CFEs
The structure and Co-TPFC are shown in Fig. 1A. The Co-TPFC and MWNT form the nanocomposite possibly through electrostatic or additional interactions, for instance π–π stacking and van der Waals forces. Such multiple interactions will favor the stability of the catalysts on the substrate. Fig. 1B and C display the scanning electron microscopy (SEM) images of the CFE and Co-TPFC/MWNT/CFE. Compared with the smooth surface of CFE (diameter of about 5 μm), the Co-TPFC/MWNT/CFE shows that the Co-TPFC/MWNT nanocomposite is densely packed on the carbon fiber surface (diameter of about 7 μm). To evaluate the electrocatalytic properties toward the reduction of O2, Fig. 1D and E compare the performance of O2 reduction at the CFE and Co-TPFC/MWNT/CFE. At the bare CFE, O2 reduction reaches a steady state at ca. −0.4 V with a half-peak potential of ca. −0.28 V, indicating a sluggish overall kinetic process for O2 reduction. In contrast, at the Co-TPFC/MWNT/CFE, O2 reduction reaches a well-defined steady state at −0.2 V with a half-peak potential of ca. −0.13 V. Moreover, the O2 reduction current was greatly enhanced by about 6 times at the Co-TPFC/MWNT/CFE. These results demonstrated the excellent electrocatalytic properties of the Co-TPFC/MWNT nanocomposite for the reduction of O2. These properties are due to the unique electronic and nanostructure properties of the MWNT and the synergistic effect of the MWNT and Co-TPFC, which is consistent with our earlier work.
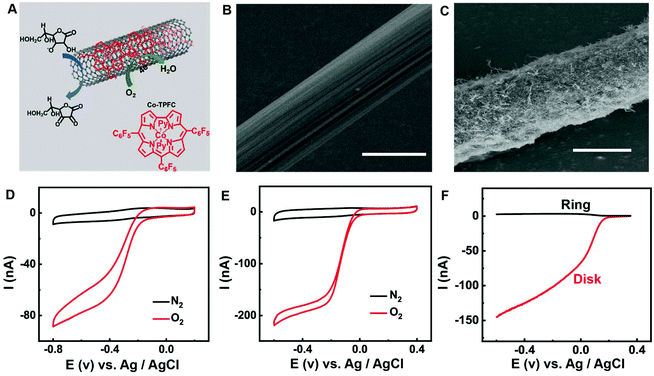 |
| Fig. 1 (A) Schematic of the Co-TPFC/MWNT nanocomposite. SEM images of (B) CFE, (C) Co-TPFC/MWNT/CFE, scale bars, 5 μm. Cyclic voltammograms (CVs) obtained at (D) CFE, (E) Co-TPFC/MWNT/CFE in aCSF (pH 7.4) saturated with N2 (black curves) or O2 (red curves). Scan rate: 50 mV s−1. (F) The rotating ring disk electrode (RRDE) voltammograms obtained for O2 reduction at the Co-TPFC/MWNT modified GC disk electrode in O2-saturated aCSF (pH 7.4) at 400 rpm. The ring Pt electrode was polarized at +1.0 V vs. Ag/AgCl. | |
O2 reduction reaction involves two competing reaction pathways in neutral media: (a) 4e reduction directly to H2O; (b) 2e reduction to H2O2. The 2e reduction process will form the H2O2 intermediate which is toxic to the brain tissue and should avoid it during in vivo O2 detection. Thus, 4e process of O2 reduction reaction is better for in vivo O2 monitor. Fig. 1F shows the rotating ring disk electrode (RRDE) measurement of the Co-TPFC/MWNT nanocomposite modified carbon electrode under physiological conditions. In the RRDE experiment, the ring potential was held at 1.0 V vs. Ag/AgCl to completely oxidize H2O2 to O2. The result shows that the current detected at the ring electrode for the Co-TPFC/MWNT is much lower. To further quantitatively characterize the Co-TPFC/MWNT modified electrode, the electron transfer number (n) per O2 molecule at the Co-TPFC/MWNT modified electrode was calculated to be 3.6, which is much closer to 4.0 and it is better than the Co-TPFC and MWNT modified electrode which was investigated in our earlier work. It means that the Co-TPFC/MWNT/CFE is suitable for in vivo monitoring of O2.
As we know, AA behaves as an inner sphere redox system on carbon surfaces with kinetic sensitivity to surface modification. Previous work shows that carbon nanotubes could greatly facilitate the oxidation of AA at low potential (ca. −50 mV), providing a new platform for selective AA detection in vivo.9–15Fig. 2 presents the AA oxidation at the CFE and Co-TPFC/MWNT/CFE, respectively. As we can see, unlike the bad performance at the CFE, AA oxidation reaches a well-defined steady state at 0.0 V for the Co-TPFC/MWNT/CFE, just as the oxidation at the MWNT/CFE. This result indicates that the Co-TPFC absorbed on the surface of the MWNT will not influence its electrocatalytic properties for AA, which is probably because Co-TPFC is absorbed on the sidewall of the MWNT, and the edge plane-like carbon at the MWNT plays the main role in facilitating the oxidation of AA.10
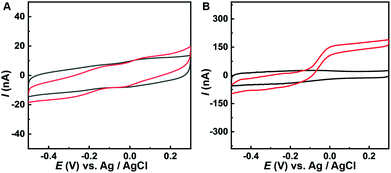 |
| Fig. 2 CV obtained at (A) CFE and (B) Co-TPFC/MWNT/CFE in aCSF removal of oxygen (pH 7.4) in the absence (black curves) and presence (red curves) of 0.50 mM AA. Scan rate, 50 mV s−1. | |
The excellent electrochemical properties of the Co-TPFC/MWNT/CFE make it possible for the selective detection of O2 and AA simultaneously even with other electroactive species coexisting in the cerebral systems. As shown in Fig. 3A, compared to 30 μM O2, the presence of other electrochemically active species at their physiological levels in the cerebral system, including 400 μM AA, 10 μM DA, 20 μM DOPAC, 50 μM UA, 10 μM 5-HT, and 10 μM H2O2 did not produce any observable current response when the electrode was poised at −0.2 V, suggesting that these species do not interfere with the monitoring of O2. In Fig. 3B, the electrode was poised at +0.05 V, and compared to 200 μM AA the presence of other electrochemically active species at their physiological levels in the cerebral system, including 10 μM DA, 20 μM DOPAC, 50 μM UA, and 10 μM 5-HT also did not produce any interference for the signal of AA.
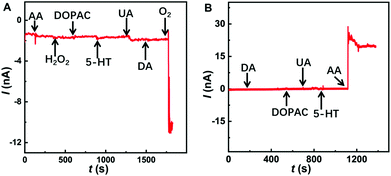 |
| Fig. 3 (A) Typical amperometric response of 400 μM AA, 10 μM DA, 20 μM DOPAC, 50 μM UA, 10 μM 5-HT, 10 μM H2O2 and 30 μM O2 at the Co-TPFC/MWNT/CFE in aCSF (pH 7.4). The electrode was poised at −0.2 V vs. Ag/AgCl. (B) Typical amperometric response of 10 μM DA, 20 μM DOPAC, 50 μM UA, 10 μM 5-HT and 200 μM AA at the Co-TPFC/MWNT/CFE in aCSF (pH 7.4). The electrode was poised at +0.05 V vs. Ag/AgCl. | |
Monitoring of oxygen and AA simultaneously
In order to monitor O2 and AA simultaneously, we use multi-potential step amperometry (one cathodic (−0.2 V) and the other anodic (+0.05 V)) to detect O2 and AA, respectively. As we know, at the beginning of each potential step, the current contains the capacitance current and Faraday current. The capacitance current decays faster than the faradaic current after applying each potential pulse, as displayed in eqn (1) (where the terms Rs is the solution resistance (70 Ω) and Cd is the differential capacitance of the double layer (3.44 × 10−7 F), i is the response current, t is time and E is the potential of an electrode versus a reference). When t = 3RsCd (about 24 μs in this system), the capacitance current decreases to 5% of the initial value. On the other hand, from eqn (2) (where the terms F is the Faraday constant, A is the electrode area, t is time, D0 is the diffusion coefficient,
is equilibrium concentrations and C0 is the initial concentration) we can know that the diffusion layer grows with t1/2 and the Faraday current decays with t−1/2.44 Therefore, we set 1 second as the duration time of each potential step and currents were measured towards the end of each step when the current reached a plateau. In addition, AA and O2 have 1 second resting test interval, which minimizes their depletion in the restricted diffusion environment of the brain tissue. The effective time resolution for O2 and AA detection was therefore 2 seconds. |  | (1) |
| 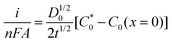 | (2) |
We have studied the stability of the prepared Co-TPFC/MWNT/CFE using this method. As shown in Fig. 4B, the Co-TPFC/MWNT/CFE used in this study exhibited good stability with only 8.0% decrease in the current after continuously measuring air-saturated O2 in aCSF at −0.2 V step potential. And the signal of AA also shows good stability with only 6.0% decrease in the current after the continuous measurement of 0.2 mM AA in aCSF at +0.05 V step potential for 60 min. This decrease might be ascribed to diffusion depletion, electrode fouling and the degradation of the electrocatalyst.9,16,42
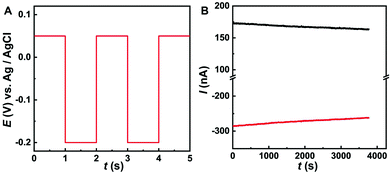 |
| Fig. 4 (A) Schematic diagram of the potential-time waveform corresponding to multi-potential step amperometry for detecting O2 reduction and AA oxidation. (B) Amperometric current response for 0.5 mM AA (black curves) and O2 (red curves) of ambient air recorded with the Co-TPFC/MWNT/CFE in aCSF (pH 7.4) by using multi-potential step amperometry, O2 at −0.2 V vs. Ag/AgCl and AA at +0.05 V vs. Ag/AgCl, step time is 1 s. | |
The Co-TPFC/MWNT/CFE also showed good linearity for the measurement of O2 and AA by this multi-potential step amperometry method. Fig. 5A shows a typical amperometric response of the Co-TPFC/MWNT/CFE upon successive injections of AA into stirring air-saturated aCSF (pH 7.4) from 0.05 to 0.25 mM at an applied alternating potential between −0.2 V and +0.05 V. As shown in Fig. 5B, the increasing current was linear with the concentration of AA [I (nA) = 203.2 CAA (mM) + 46.5, R2 = 0.9988]. Fig. 5C shows a typical amperometric response upon successive injections of O2 into stirring N2 saturated aCSF (pH 7.4) from 0.025 to 0.15 mM. The increasing current was also linear with the concentration of O2 [I (nA) = −292.3 CO2 (mM) − 70.9, R2 = 0.9996]. Moreover, from Fig. 5A and C, we can also see that even after successive injections of AA or O2 into the solution, the signal of another substance did not show any change. It means that there was no interference between O2 and AA in this method. These results suggested that the Co-TPFC/MWNT/CFE provides a new way by using multi-potential step amperometry for detecting O2 and AA simultaneously. It also shows good selectivity, stability and linearity, and should be useful for physiological and pathological investigations in general.
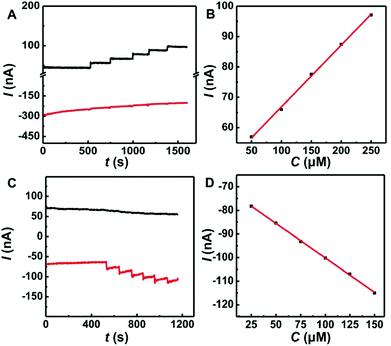 |
| Fig. 5 (A) Typical amperometric responses obtained at the Co-TPFC/MWNT/CFE towards successive additions of AA (black curves) in aCSF (pH 7.4). (B) The calibration plot of steady-state current measurement against the AA concentration. (C) Typical amperometric responses obtained at the Co-TPFC/MWNT/CFE towards successive additions of O2 (red curves) in aCSF (pH 7.4) saturated with N2 (D). The calibration plot of steady-state current measurement against the AA concentration. At an applied alternating potential between −0.2 V and +0.05 V vs. Ag/AgCl with 1 s step time each. | |
In vivo monitoring of O2 and AA simultaneously during ischemia
We demonstrate the feasibility for in vivo application of the Co-TPFC/MWNT/CFE developed in this study. The Co-TPFC/MWNT/CFE was used to investigate the O2 and AA activities simultaneously in the rat brain under ischemia. The schematic of the surgical procedures of 2-VO ischemia is shown in Fig. 6A. A typical dynamic current response is recorded with the Co-TPFC/MWNT/CFE under ischemia (Fig. 6B). When the animal was subjected to global ischemia by occluding the bilateral common carotid arteries, the O2 level was quickly decreased because the O2 supply was cut off. Meanwhile, the AA level quickly increased, which was related to a comprehensive result of a series of neuropathological processes including anoxia depolarization, glutamate release and reuptake, glutamate excitotoxicity, accumulation of oxidative free radicals, and even neural cell necrosis. The result is consistent with the earlier study that observed the dynamic change of O2 and AA, respectively, under ischemia.16,45 This result demonstrated that the developed electrochemical method with the Co-TPFC/MWNT/CFE could be used for in vivo reliable measurements of O2 and AA simultaneously in the rat brain and thus, could potentially be useful for physiological and pathological investigations.
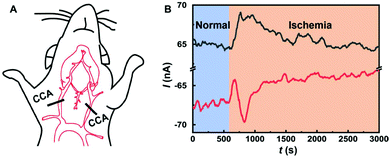 |
| Fig. 6 (A) The schematic of the surgical procedures of 2-VO ischemia. (B) Amperometric response for the hippocampus O2 (red curves) and AA (black curves) recorded in anesthetized rats during global ischemia by using multi-potential step amperometry, O2 at −0.2 V vs. Ag/AgCl and AA at +0.05 V vs. Ag/AgCl, 1 s step time each. | |
Conclusion
In summary, by taking advantage of the excellent electrochemical properties of the Co-TPFC/MWNT nanocomposite for catalysis toward O2 and facilitating the oxidation of AA, we have successfully developed a new electrochemical method by using multi-potential step amperometry for in vivo measurement of O2 and AA simultaneously with the Co-TPFC/MWNT/CFE. Compared with the existing microelectrodes for detecting brain O2 and AA, respectively, this microelectrode can not only reduce O2 according to a four-electron process without the formation of toxic H2O2 and facilitate the oxidation of AA, but can also record the dynamic at the same location in the rat brain. Both in vitro and in vivo experiments demonstrated that the Co-TPFC/MWNT/CFE possesses high selectivity and stability useful for selective and reliable measurements of O2 and AA simultaneously in the rat brain. This study offers a new analytical platform for in vivo O2 and AA measurements. It is useful for us to understand various physiological and pathological processes associated with the co-dynamic change of O2 and AA, and also expand the idea for detecting multiple substances simultaneously.
Conflicts of interest
There are no conflicts to declare.
Acknowledgements
We greatly acknowledge the financial support from the National Natural Science Foundation of China (Grant No. 21874152) and also thank Prof. Rui Cao and Dr Haitao Lei for providing cobalt corrole.
References
- M. Zhang, P. Yu and L. Mao, Acc. Chem. Res., 2012, 45, 533–543 CrossRef CAS.
- T. Xiao, F. Wu, J. Hao, M. Zhang, P. Yu and L. Mao, Anal. Chem., 2016, 89, 300–313 CrossRef.
- M. Ganesana, S. T. Lee, Y. Wang and B. J. Venton, Anal. Chem., 2017, 89, 314–341 CrossRef CAS.
- H. Dong, Q. Zhou, L. Zhang and Y. Tian, Angew. Chem., Int. Ed., 2019, 58, 13948–13953 CrossRef CAS PubMed.
- Y. Li, S. Zhang, X. Wang, X. Zhang, A. I. Oleinick, I. Svir, C. Amatore and W. Huang, Angew. Chem., Int. Ed., 2015, 54, 9313–9318 CrossRef CAS PubMed.
- R. M. Wightman, L. J. May and A. C. Michael, Anal. Chem., 1988, 60, 769A–793A CrossRef CAS.
- P. E. Phillips, G. D. Stuber, M. L. Heien, R. M. Wightman and R. M. Carelli, Nature, 2003, 422, 614 CrossRef CAS PubMed.
- X. Liu, T. Xiao, F. Wu, M. Shen, M. Zhang, H. Yu and L. Mao, Angew. Chem., Int. Ed., 2017, 56, 11802–11806 CrossRef CAS PubMed.
- M. Zhang, K. Liu, L. Xiang, Y. Lin, L. Su and L. Mao, Anal. Chem., 2007, 79, 6559–6565 CrossRef CAS PubMed.
- L. Xiang, P. Yu, J. Hao, M. Zhang, L. Zhu, L. Dai and L. Mao, Anal. Chem., 2014, 86, 3909–3914 CrossRef CAS.
- H. Cheng, L. Li, M. Zhang, Y. Jiang, P. Yu, F. Ma and L. Mao, TrAC, Trends Anal. Chem., 2018, 109, 247–259 CrossRef CAS.
- T. Xiao, Y. Wang, H. Wei, P. Yu, Y. Jiang and L. Mao, Angew. Chem., Int. Ed., 2019, 131, 6688–6691 CrossRef.
- T. Xiao, Y. Jiang, W. Ji and L. Mao, Anal. Chem., 2018, 90, 4840–4846 CrossRef CAS.
- X. Liu, M. Zhang, T. Xiao, J. Hao, R. Li and L. Mao, Anal. Chem., 2016, 88, 7238–7244 CrossRef CAS PubMed.
- L. Zhang, F. Liu, X. Sun, G. Wei, Y. Tian, Z. Liu, R. Huang, Y. Yu and H. Peng, Anal. Chem., 2017, 89, 1831–1837 CrossRef CAS.
- L. Xiang, P. Yu, M. Zhang, J. Hao, Y. Wang, L. Zhu, L. Dai and L. Mao, Anal. Chem., 2014, 86, 5017–5023 CrossRef CAS PubMed.
- R. Li, X. Liu, W. Qiu and M. Zhang, Anal. Chem., 2016, 88, 7769–7776 CrossRef CAS PubMed.
- S. Wang, X. Liu and M. Zhang, Anal. Chem., 2017, 89, 5382–5388 CrossRef CAS PubMed.
- X. Hu, Y. Liu, H. Zhang, C. Xiao, Y. Qin, H. Duo, J. Xu, S. Guo, D. Pang and W. Huang, ChemElectroChem, 2016, 3, 1998–2002 CrossRef CAS.
- M. Zhu, C. Zeng, J. Ye and Y. Sun, Appl. Surf. Sci., 2018, 455, 646–652 CrossRef CAS.
- F. Crespi, T. Sharp, N. T. Maidment and C. A. Marsden, Brain Res., 1984, 322, 135–138 CrossRef CAS.
- J. B. Zimmerman and R. M. Wightman, Anal. Chem., 1991, 63, 24–28 CrossRef CAS.
- M. K. Zachek, P. Takmakov, B. Moody, R. M. Wightman and G. S. McCarty, Anal. Chem., 2009, 81, 6258–6265 CrossRef CAS.
- S. S. Park, M. Hong, C. K. Song, G. J. Jhon, Y. Lee and M. Suh, Anal. Chem., 2010, 82, 7618–7624 CrossRef CAS PubMed.
- H. Xu, L. Wang, J. Luo, Y. Song, J. Liu, S. Zhang and X. Cai, Sensors, 2015, 15, 1008–1021 CrossRef CAS PubMed.
- G. Jobst, I. Moser, M. Varahram, P. Svasek, E. Aschauer, Z. Trajanoski, P. Wach, P. Kotanko, F. Skrabal and G. Urban, Anal. Chem., 1996, 68, 3173–3179 CrossRef CAS.
- M. E. Sandison, N. Anicet, A. Glidle and J. M. Cooper, Anal. Chem., 2002, 74, 5717–5725 CrossRef CAS PubMed.
- P. Yu and G. S. Wilson, Faraday Discuss., 2000, 116, 305–317 RSC.
- B. Ghane-Motlagh and M. Sawan, Mater. Sci. Appl., 2013, 04, 483–495 Search PubMed.
- T. Xiao, X. Li, H. Wei, W. Ji, Q. Yue, P. Yu and L. Mao, Anal. Chem., 2018, 90, 13783–13789 CrossRef CAS.
- J. K. Thompson, M. R. Peterson and R. D. Freeman, Science, 2003, 299, 1070–1072 CrossRef CAS.
- N. J. Finnerty, F. B. Bolger, E. Palsson and J. P. Lowry, ACS Chem. Neurosci., 2013, 4, 825–831 CrossRef CAS.
- H. Shi and K. Liu, Front. Biosci., 2007, 12, 1318–1328 CrossRef CAS.
- H. A. Kontos, Stroke, 2001, 11, 2712–2716 CrossRef PubMed.
- J. X. Wilson and E. M. Jaworski, Neurochem. Res., 1992, 17, 571–576 CrossRef CAS.
- K. Dabrowski, K. J. Lee, L. Guz, V. Verlhac and J. Gabaudan, Aquaculture, 2004, 233, 383–392 CrossRef CAS.
- T. Finkel and N. J. Holbrook, Nature, 2000, 408, 239–247 CrossRef CAS PubMed.
- J. B. Zimmerman and R. M. Wightman, Anal. Chem., 1991, 63, 24–28 CrossRef CAS.
- B. J. Venton, D. J. Michael and R. M. Wightman, J. Neurochem., 2003, 84, 373–381 CrossRef CAS.
- R. Trouillon, Y. Lin, L. J. Mellander, J. D. Keighron and A. G. Ewing, Anal. Chem., 2013, 85, 6421–6428 CrossRef CAS.
- F. B. Bolger, R. Bennett and J. P. Lowry, Analyst, 2011, 136, 4028–4035 RSC.
- Z. Wang, H. Lei, R. Cao and M. Zhang, Electrochim. Acta, 2015, 171, 81–88 CrossRef CAS.
- H. Lei, A. Han, F. Li, M. Zhang, Y. Han, P. Du, W. Lai and R. Cao, Chem. Chem. Phys., 2014, 16, 1883–1893 RSC.
-
A. J. Bard and L. R. Faulkner, Electrochemical Methods: Fundamentals and Applications, Wiley & Sons, New York, 2nd edn, 2001, p. 15 Search PubMed.
- K. Liu, P. Yu, Y. Lin, Y. Wang, T. Ohsaka and L. Mao, Anal. Chem., 2013, 85, 9947–9954 CrossRef CAS.
|
This journal is © The Royal Society of Chemistry 2020 |
Click here to see how this site uses Cookies. View our privacy policy here.