DOI:
10.1039/C9AN01935A
(Paper)
Analyst, 2020,
145, 132-138
A surface-enhanced electrochemiluminescence sensor based on Au-SiO2 core–shell nanocomposites doped with Ru(bpy)32+ for the ultrasensitive detection of prostate-specific antigen in human serum
Received
28th September 2019
, Accepted 5th November 2019
First published on 6th November 2019
Abstract
This study reports a surface-enhanced electrochemiluminescence (SEECL) sensor for the ultrasensitive detection of prostate-specific antigen (PSA) in human serum. First, we prepared the Au-SiO2 core–shell nanocomposites doped with Ru(bpy)32+ (Au@SiO2-Ru) as the ECL signal generation and amplification element of the SEECL sensor. Ru(bpy)32+ could emit ECL under the excitation of an electrochemical reaction, and generate the local surface plasmon resonance (LSPR) electromagnetic field from the gold nanoparticle (AuNP) core. The produced LSPR then effectively enhanced the ECL signals. This sensing strategy was utilized for the detection of PSA, which can be absorbed on the electrode surface by the reaction between PSA and monoclonal antibodies. When polyclonal antibody-modified Au@SiO2-Ru was added, the PSA-double-antibody sandwich structure was formed on the electrode surface and thus, the Au@SiO2-Ru were quantitatively immobilized on the electrode surface. The results show that this kind of SEECL sensor has good selectivity toward PSA. Notably, the minimum detection concentration reached 7 × 10−7 ng mL−1 under the optimum experimental conditions, which is much better than most of the previously reported approaches for the detection of PSA in serum.
1. Introduction
With the increasing attention of human beings to their own diseases, rapid and sensitive clinical diagnosis techniques are required. Currently, traditional electrochemiluminescence (ECL) technology is unable to detect trace amounts of biomolecules. Therefore, the development of a reliable and high-sensitivity ECL biosensor is the main research focus for the ECL technique in clinical diagnosis.1,2 At present, various ECL biosensors have been reported. Some were prepared by using biomolecules as the media for quantitatively controlling the ECL reagent or co-reactants on the electrode surface.3,4 Other ECL biosensors were achieved by the interaction between the target biomolecules and the co-reactants of the ECL reagent.5–7
Regardless of the method chosen for preparing ECL biosensors, the improvement of the sensitivity of the ECL biosensor is a key point for application. In order to improve the sensitivity of the ECL biosensor, many researchers have used metal nanomaterials as signal amplification elements. These studies mainly focused on the superior surface effect enrichment and the electrochemical activity of metallic nanomaterials,8–11 but only a few sensors were designed based on the local surface plasmon resonance (LSPR) enhancement effect of noble metal nanomaterials.12–14 In our previous study,15 we found that the LSPR of gold nanoparticles can effectively enhance the ECL emission of Ru(bpy)32+, and the concept of surface-enhanced electrochemiluminescence (SEECL) was put forward for the first time. We then successfully fabricated a SEECL biosensor for CEA detection.16 The results showed that this kind of SEECL biosensor has an ultra-sensitive response to CEA levels.
In this work, we designed a novel strategy for the ultra-sensitive detection of prostate-specific antigen (PSA) in serum based on SEECL. PSA is an acidic glycoprotein secreted by prostatic epithelial cells.17–19 Its content in serum is often used for the diagnosis of prostate cancer and its related diseases.20,21 Increased levels of PSA in serum indicate an increased risk of prostate-related diseases in men,22,23 and changes in serum PSA levels can also reflect the therapeutic effect of prostate cancer patients, which requires the high sensitivity detection of PSA.24–26
The principle of the proposed SEECL sensor is shown in Scheme 1. Firstly, we synthesized the Au-SiO2 core–shell composite nano-materials doped with Ru(bpy)32+ (Au@SiO2-Ru), and modified polyclonal antibody (Ab2) on the surface of Au@SiO2-Ru for specifically recognizing PSA. At the same time, we modified monoclonal antibodies (Ab1) on the surface of the gold electrode. In the presence of PSA, the double-antibody sandwich structure was formed by the immune reaction between PSA and the antibodies, so that the Au@SiO2-Ru could be quantitatively immobilized on the electrode surface. As we previously reported,15 the Au@SiO2-Ru composite nanomaterials not only retained the ECL activity of Ru(bpy)32+ to generate signals in ECL testing, but also had the LSPR effect of the gold nucleus to enhance the ECL signal. Therefore, the Au@SiO2-Ru composite nanomaterials combine the functions of signal generation and amplification, which greatly improve the sensitivity of the SEECL sensor. As a result, the ultra-sensitive detection of PSA is achieved.
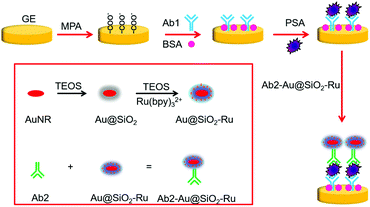 |
| Scheme 1 Schematic of the SEECL sensor for PSA detection. | |
2. Materials and methods
2.1. Apparatus and reagents
The UV-vis absorption spectra were obtained on a Shimadzu UV-3600 UV-vis-NIR photo spectrometer (PerkinElmer Co., USA). The ECL measurements were recorded by an ultra-weak luminescence analyzer (BPCL-1-TIC) at room temperature, with 1000 V voltage of the PMT. A CHI 660D electrochemical workstation (Shanghai CHI instruments Co., China) was used for recording the electrochemical measurements. All experiments were carried out with a conventional three-electrode system: a Pt wire served as the counter electrode, a Ag/AgCl electrode was used as the reference electrode, and the working electrode was the modified gold electrode (GE, ϕ = 2 mm).
PSA antigen standard samples and their antibodies are products of Abnova Biotech Co., Ltd. Prostate-specific antigen standard protein (No. KLK3 human recombinant protein) was dissolved in 0.05 M pH 8.0 Tris-HCl buffer solution containing 10 mM reduced glutathione. KLK3 mouse monoclonal antibody (Ab1) and rabbit-derived polyclonal antibody (Ab2) of KLK3 were prepared with 0.01 M pH 7.4 PBS buffer solution. The antigen and antibody reserves were stored in the refrigerator at 4 °C. Bovine serum albumin (BSA), thrombin, trypsin and lysozyme were purchased from Shanghai Bioengineering Technology Co. Ltd. Tris(2,2′-bipyridyl) dichlororuthenium(II) hexahydrate (Ru(bpy)3Cl2·6H2O) was purchased from Sigma-Aldrich. Hydrogen tetrachloroaurate tetrahydrate (HAuCl4·4H2O), and cetyltrimethyl ammonium bromide (CTAB), tri-n-propylamine (TPrA), L-ascorbic acid (VC) and other reactants were obtained from Sinopharm Chemical Reagent Co. Ltd (Shanghai, China). All reagents were of analytical grade and were used as received. Millipore ultrapure water (resistivity >18.2 MΩ cm) was used throughout the experiment.
2.2. Preparation of AuNR
2.2.1. Gold nanoparticles (AuNP).
The synthesis of AuNP was carried out using the chemical reduction method reported by Frens, outlined as follows.27 Firstly, 3 mL of 1% (w/v) tri-sodium citrate solution was injected into 50 mL of boiling 0.15 mM HAuCl4 aqueous solution. After the color of the solution changed to wine red, stirring was continued for 30 minutes, after which it was statically cooled to room temperature, centrifuged, and dissolved in an equal volume of secondary water.
2.2.2. Gold nanorods (AuNR).
AuNRs were synthesized by a seed-mediated method.28 Briefly, 0.6 mL of 0.01 M NaBH4 in fresh ice was injected into an aqueous solution containing 9.75 mL of 0.1 M CTAB and 0.25 mL of 0.01 M HAuCl4 under vigorous stirring to obtain the gold seed solution. Next, 40 mL 0.1 M CTAB, 2 mL 0.01 M HAuCl4, 0.4 mL 0.01 M AgNO3, 0.32 mL 0.1 M freshly prepared VC solution and 0.8 mL 1.0 M HCl were added, in turn, to a 50 mL flask, followed by the addition of 0.01 mL gold seed solution. The mixture solution was left undisturbed for at least 6 h at room temperature. In order to obtain AuNR with different aspect ratios, O2 was bubbled into the AuNR solution for 10 min with the injection of 0.8 mL 1.0 M HCl. The mixture solution was then incubated in a water bath at 65 °C for varied durations, ranging from 2 hours to 24 hours. Finally, the AuNR solution was centrifuged at 8500 rpm for 10 min and the precipitate was redispersed in H2O for further characterization.
2.3. Preparation of Ab2-Au@SiO2-Ru
2.3.1. Synthesis of Au@SiO2.
A mixture containing 6 mL ethanol, 0.1 mL 25% NH3·H2O and 2.0 mL AuNR or AuNP solution was prepared, then 4 μL TEOS was injected into the mixture under stirring. After stirring at 25 °C for 5 hours, the reaction solution was separated twice by centrifugation at 7500 rpm for 5 min and re-dissolved in 2.0 mL water.
2.3.2. Preparation of Au@SiO2-Ru.
To 2.0 mL of Au@SiO2 solution, 50 μL 0.1 M NaOH solution was added and mixed. This solution mixture was then injected with 2 μL of 10.0 mM Ru(bpy)3Cl2 and 20 μL 20% (v/v) TEOS-ethanol solution and allowed to react overnight at 25 °C. The final reaction solution was separated three times by centrifugation and dissolved in 2.0 mL of 0.01 M PBS at pH 7.4.
2.3.3. Preparation of Ab2-Au@SiO2-Ru.
Au@SiO2-Ru (200 μL) was reacted with 200 μL of 1
:
1000 Ab2-PBS solution at 4 °C for 24 hours in a dark place. After centrifugation (5000 rpm, 5 min), the supernatant Ab2 was removed, and the deposits were dissolved in 200 μL of 0.01 M pH 7.4 PBS buffer solution. The Ab2-Au@SiO2-Ru should be stored at 4 °C in a refrigerator.
2.4. Fabrication of the SEECL sensor
2.4.1. Electrode activation.
The gold electrode (GE) surface was polished with 0.5 μm and 0.05 μm Al2O3 polishing powder and washed in 50% (v/v) HNO3 solution, 50% (v/v) ethanol-aqueous solution and H2O for 3 minutes in turn. The cleaned electrode was immersed in 0.5 M H2SO4 solution and 10 cyclic voltammetry (CV) scans were conducted in the potential range from −0.4 V to 1.6 V with a sweep rate of 0.5 V s−1.
2.4.2. Electrode modification.
The surface of the activated GE was covered with carboxyl groups via Au–S bonds after being placed in 0.05 M mercaptopropionic acid (MPA) for 2 hours. The electrode was then placed into the 0.01 M pH 7.4 PBS with 100 ng mL−1 Ab1 at 37 °C for 2 hours to obtain the monoclonal antibody-modified electrode (GE/Ab1). After using the 0.05% BSA-PBS (pH 7.4) solution to seal the electrode surface, the GE/Ab1 was incubated in PSA protein solution to form the PSA modified electrode (GE/Ab1/PSA). Finally, the 5 μL Ab2-Au@SiO2-Ru solution was reacted with the GE/Ab1/PSA for 12 hours, and the excess reaction solution was carefully washed out with 0.01 M pH 7.4 PBS. The modified electrode (GE/Ab1/PSA/Ab2-Au@SiO2-Ru) was placed in 0.1 M pH 7.4 PBS solution and scanned 5 times to clean the surface.
2.5. ECL detection
A 5.0 mL cylinder quartz beaker was used as a reaction tank and the modified electrode was dipped into 1 mM TPA 0.1 M pH 7.4 PBS solution for ECL testing. The potential range was −0.5 V to 1.5 V with the sweep rate of 0.1 V s−1.
3. Results and discussion
3.1. LSPR of AuNR
LSPR is a unique optical physical phenomenon of precious metal nanomaterials, which can be investigated by UV-vis absorption spectra. The AuNR have transverse and longitudinal LSPR absorption peaks. In general, the transverse LSPR absorption peaks are located at about 520 nm, while the longitudinal LSPR absorption peaks vary in the wavelength range from 520 nm to 1000 nm with different length-diameter ratios of AuNR.28 With the increase in oil bath time and the oxidation degree of AuNR growth solution added, the longitudinal LSPR absorption peak gradually shifted from 750 nm to 520 nm (as shown in Fig. 1(A)). The corresponding solution color also gradually changed from brown to pink (as shown in Fig. 1(C)).
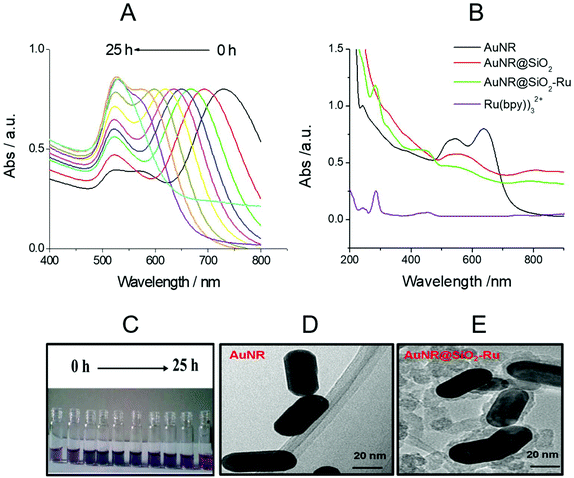 |
| Fig. 1 (A) UV-vis adsorption spectra of AuNR; (B) UV-vis adsorption spectra of different nanoparticles. (C) The variation in the color of the AuNR solution with oil bath time; TEM images of AuNR (D) and AuNR@SiO2-Ru (E). | |
As shown in Fig. 1(B), as the interface environment of the precious metal nano-materials changed, the LSRP absorption peak also changed accordingly.29 The surface refractive index of AuNR became larger and the LSPR absorption peak obviously red-shifted after coating with SiO2. The spectrum of Ru(bpy)32+ (with the concentration is 2.0 μM) is displayed in Fig. 1(B). There were two characteristic absorption peaks of Ru(bpy)32+ at 280 nm and 460 nm,30 respectively, and the same two absorption peaks also appeared in the spectrum of Au@SiO2-Ru (green curve in Fig. 1(B)), indicating the existence of Ru(bpy)32+ in Au@SiO2-Ru. Moreover, the TEM diagrams (see Fig. 1(D) and (E)) further illustrated that the surface of the AuNR was successfully coated with a 8 nm thick layer of SiO2.
3.2. Characterization of modified electrodes
Electrochemical Impedance spectroscopy (EIS) is an effective interfacial analysis technique for studying the features of electrode surfaces modified with bio-molecules.31–34 Nyquist plots of EIS with different electrode modifications are displayed in Fig. 2. The semicircle diameter obtained from the Nyquist plots of EIS represents the interfacial resistance at the electrode surface. Compared with the bare GE (curve a), the resistance obviously increased after the electrode was modified with antibody and proteins (curve b and curve c) because the antibody and proteins hindered electron transfer from the electrode. With the addition of PSA, the antigen–antibody structure was formed on the electrode surface. Thus, interfacial resistance further increased by thickening the bio-film on the electrode surface (curve d). When the electrode was modified with Ab2-Au@SiO2-Ru, maximum resistance was observed (curve e). The remarkable increase in resistance could be induced by the formation of the antibody–antigen sandwich structure and the hydrophobicity of the composite nanoparticles.
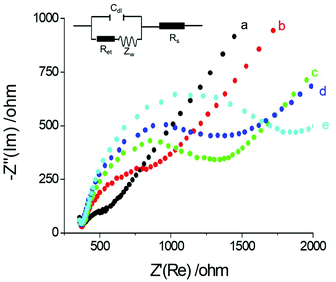 |
| Fig. 2 Nyquist plots of different modified electrodes. The Nyquist plots were recorded in PBS solution (0.1 M, pH 7.0), containing 5.0 mM K3Fe(CN)6, biasing potential 0.21 V, 5 mV alternative voltage in the frequency range of 1 Hz–100 000 Hz (curve a: GE; curve b: GE/Ab1; curve c: GE/Ab1/BSA; curve d: GE/Ab1/PSA; curve e: GE/Ab1/PSA/Ab2-Au@SiO2-Ru). | |
3.3. The effect of the gold nucleus on ECL enhancement
The LSPR of noble metal nanomaterials is closely related to their morphology and size. In order to investigate the effect of the Au nucleus on the ECL enhancement, several different gold nanoparticles were selected as the crystal cores of Au@SiO2-Ru composite nanomaterials (shown in Fig. 3). These include (1) the spherical gold nanoparticles with LSPR absorption peak at 520 nm (Au-520); (2) the elliptical gold nanoparticles with LSPR absorption peak of 567 nm (Au-567); (3) four different aspect ratios of gold nanorods with the longitudinal LSPR absorption peaks at 635 nm (Au-635), 680 nm (Au-680), 721 nm (Au-721) and 800 nm (Au-800), respectively. We also selected SiO2 nanoparticles with a particle size of 30 nm as the crystal core to prepare SiO2-Ru(bpy)32+ composite nanomaterials (SiO2-Ru) by the same method. This was used for the control experiments for SEECL testing.
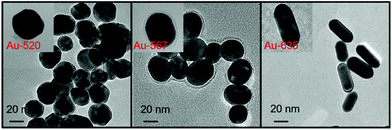 |
| Fig. 3 TEM images of different Au nuclei of Au@SiO2-Ru composite nanoparticles. | |
The ECL test results of the SEECL sensors prepared using different crystal cores are shown in Fig. 4(B). Under the same experimental conditions and similar surface state of the modified electrodes (as shown in Fig. 4(A)), the Au@SiO2-Ru modified electrode had a stronger ECL signal than the SiO2-Ru modified electrode. On changing the Au nucleus from spherical to rod-shaped, the ECL signals increased rapidly; this is due to a change in the LSPR of the gold nucleus. The AuNPs have only one LSPR peak at ∼520 nm, while the AuNRs have two LSPR absorption peaks, namely, the transverse and longitudinal peaks. The transverse absorption peak of gold nanorods is generally located at ∼520 nm, and the longitudinal absorption peaks change in the range 520 nm–800 nm with the different aspect ratios of nanorods.
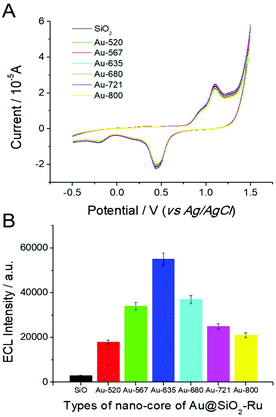 |
| Fig. 4 Simultaneous electrochemical current measurement (A) and ECL emission (B) of different SEECL sensors with different Au nuclei in 0.1 M PBS (pH 7.4) solution containing 1 mM TPA at scanning rate of 0.1 V s−1. The PSA concentration was 7.0 ng mL−1. | |
The induced electromagnetic field produced by the co-generation of two LSPR absorption bands in AuNR is more beneficial for exciting Ru(bpy)32+. In particular, when the longitudinal LSPR absorption peak of AuNR reached 635 nm, the absorption spectra overlapped with the ECL emission spectra (λem = 630 nm) of Ru(bpy)32+. In this situation, the LSPR of AuNR was more easily induced by the excited-state Ru(bpy)32+, thus the LSPR induced electromagnetic field was more efficient in enhancing the quantum yield of the ground-state Ru(bpy)32+. Moreover, Fig. 4(B) also indicates that with the increase of the longitudinal absorption peak wavelength of AuNR from 635 nm to 800 nm, the ECL signal of the sensor gradually decreased. When the LSPR absorption peak of AuNRs reached 820 nm, the ECL signal was similar to that of AuNPs.
3.4. Detection of PSA
The relationship between the ECL response of the sensor and the PSA test concentration was investigated as shown in Fig. 5. It indicated that the concentration of PSA in the system has a direct effect on the ECL response of the SEECL sensor, and the minimum response concentration of PSA can reach 7 × 10−7 ng mL−1, which is significantly lower than that of other analytical methods.9,35,36
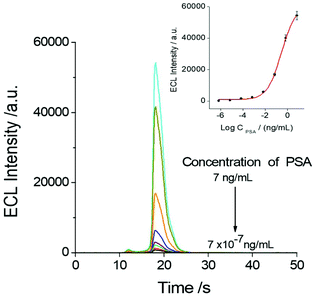 |
| Fig. 5 ECL emission of the Au@SiO2-Ru modified electrode with different PSA concentrations in 0.1 M pH 7.4 PBS containing 1.0 mM TPA with a scan rate of 100 mV s−1. The inset shows the relationship between the PSA concentration and ECL intensity. | |
3.5. The selectivity of the SEECL sensor
In order to test the selectivity of the SEECL sensor to PSA, four different proteins were detected in this paper. These four proteins were bovine serum albumin (BSA), thrombin, trypsin and lysozyme. The selected concentration of these four proteins was 70 ng mL−1, which was 100 times higher than that of PSA. Fig. 6 shows the response of SEECL sensors to various proteins. The results show that only negligible responses were observed for the four interfering proteins, while a significant response was generated in the presence of PSA. These results indicate that the SEECL sensor designed in this work has good selectivity toward PSA.
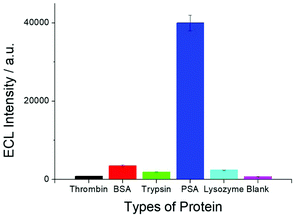 |
| Fig. 6 ECL responses of SEECL sensors for different proteins in the same 0.1 M pH 7.4 PBS containing 1.0 mM TPA; the CV scanning rate was 0.1 V s−1. The concentration of the PSA was 0.7 ng mL−1, while the concentration of the others proteins (BSA, trypsin, lysozyme and thrombin) was 70.0 ng mL−1. | |
3.6. Reproducibility and stability of the SEECL sensor
The stability and reproducibility of the SEECL sensor were also investigated. The stability of the SEECL sensor was evaluated by repeatedly measuring the ECL signal of the same SEECL sensor under the same experimental conditions. The reproducibility was obtained by collecting the ECL detection data of different SEECL sensors prepared in different batches. According to the ECL detection results in Fig. 7, the relative standard deviation (RSD) of the ECL response value of the same SEECL sensor was 4.09% (see Fig. 7(A)), while the RSD of ECL response values measured by different batches of SEECL sensors produced by the same method was 5.25% (see Fig. 7(B)).
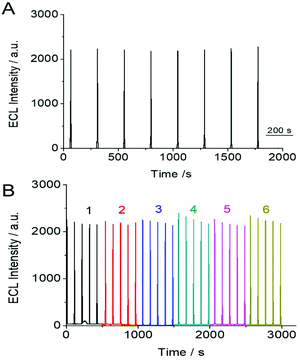 |
| Fig. 7 (A) Stability of ECL signal from one Au@SiO2-Ru modified electrode. (B) Reproducibility of the ECL signal on the Au@SiO2-Ru modified electrode collected from 6 different batches. The concentration of PSA was 7 × 10−5 ng mL−1. | |
4. Conclusions
In summary, we have demonstrated a SEECL sensor for the ultrasensitive detection of PSA in serum. With the specific reaction between antigen and antibody, the PSA in serum was captured by the Ab1 modified electrode, and then the double-antibody structure was formed in the presence of Ab2-Au@SiO2-Ru; this outlines the preparation of SEECL sensor. The more PSA is captured, the more Au@SiO2-Ru composite nanomaterials are fixed on the surface of the electrode. Therefore, the PSA content can be determined directly by measuring the ECL signal of the modified electrode. Our investigation revealed that the LSPR produced by the AuNR core of Au@SiO2-Ru greatly improved the detection sensitivity of the sensor, and the minimum concentration of PSA was 7 × 10−7 ng mL−1 under optimal conditions could be achieved. Moreover, the selective experimental results indicated the proposed SEECL sensor can be used for the selective detection of PSA. This method provides a reference for the specific detection of other proteins and antigens.
Conflicts of interest
There are no conflicts to declare.
Acknowledgements
This work was supported by the National Natural Science Foundation of China (21675028, 21507041), Nature Sciences Funding of Fujian Province (2018J01682), STS Key Project of Fujian Province (2017T3007), the Cooperative Project of Production and Study in University of Fujian Province (2018Y4007), the Natural Science Foundation of Fujian Province (2017J05020), the Science and Technology Project of Fujian Education Department (JAT160764), and the Program for New Century Excellent Talents in Fujian Province University.
References
- B. Wang, Y.-X. Dong, Y.-L. Wang, J.-T. Cao, S.-H. Ma and Y.-M. Liu, Sens. Actuators, B, 2018, 254, 159–165 CrossRef CAS.
- Y.-X. Dong, J.-T. Cao, Y.-M. Liu and S.-H. Ma, Biosens. Bioelectron., 2017, 91, 246–252 CrossRef CAS.
- Y. Nie, P. Zhang, H. Wang, Y. Zhuo, Y. Q. Chai and R. Yuan, Anal. Chem., 2017, 89(23), 12821–12827 CrossRef CAS.
- L. Zheng, C. Fang, J. Yan, H. Li and Y. Tu, Sci. Rep., 2017, 7, 11199 CrossRef.
- S. Chandra, M. Mayer and A. J. Baeumner, Talanta, 2017, 168, 126–129 CrossRef CAS.
- M.-S. Wu, N. Xu, J. Qiao, J. Chen and L.-S. Jin, Analyst, 2019, 144, 4633–4638 RSC.
- Y. Chi, Z. Chen, Y. Chen, Y. Dong and X. You, ChemElectroChem, 2017, 4, 1783–1789 CrossRef.
- Q. Li, C. Kang, K. Li, A. Chen, W. Zhang and Y.-S. Zhao, Sci. China: Chem., 2017, 60, 642–648 CrossRef CAS.
- H. W. Shi, W. Zhao, Z. Liu, X. C. Liu, M. S. Wu, J. J. Xu and H. Y. Chen, Talanta, 2016, 154, 169–174 CrossRef CAS.
- X. Li, Y. Wang, L. Shi, H. Ma, Y. Zhang, B. Du, D. Wu and Q. Wei, Biosens. Bioelectron., 2017, 96, 113 CrossRef CAS.
- J. Chen, L. Guo, B. Qiu, Z. Lin and T. Wang, Mater. Chem. Front., 2018, 2, 835–860 RSC.
- B. Wang, X. Zhong, Y. Chai and R. Yuan, Sens. Actuators, B, 2017, 245, 247–255 CrossRef CAS.
- C. Zhang, Y. Fan, H. Zhang, S. Chen and R. Yuan, Anal. Bioanal. Chem., 2018, 411, 905–913 CrossRef.
- X. Ma, S. He, B. Qiu, F. Luo, L. Guo and Z. Lin, ACS Sens., 2019, 4, 782–791 CrossRef CAS.
- D. Wang, L. Guo, H. Rong, B. Qiu, Z. Lin and G. Chen, Sci. Rep., 2015, 5, 7954 CrossRef CAS.
- D. Wang, Y. Li, Z. Lin, B. Qiu and L. Guo, Anal. Chem., 2015, 87, 5966–5972 CrossRef CAS.
- U. H. Stenman, J. Leinonen, W. M. Zhang and P. Finne, Semin. Cancer Biol., 1999, 9, 83 CrossRef CAS PubMed.
- A. M. El-Shirbiny, Adv. Clin. Chem., 1994, 31, 99–133 CAS.
- X. Jiang, S. Cheng, W. Chen, L. Wang, F. Shi and C. Zhu, Anal. Biochem., 2012, 424, 1–7 CrossRef CAS.
- M. Sasaki, S. Ishidoya, A. Ito, H. Saito, S. Yamada, K. Mitsuzuka, Y. Kaiho, D. Shibuya, T. Yamaguchi and Y. Arai, Urology, 2014, 84, 1163–1167 CrossRef.
- G. L. Andriole, B. David, O. W. Brawley, G. Leonard, M. Michael, M. Francesco, P. Curtis, T. L. J. Tammela, T. Claudio and T. Donald, J. Urol., 2011, 185, 126–131 CrossRef CAS.
- X. Ma, Y. Lin, L. Guo, B. Qiu, G. Chen, H.-h. Yang and Z. Lin, Biosens. Bioelectron., 2017, 87, 122–128 CrossRef CAS.
- X. Ma, Z. Chen, P. Kannan, Z. Lin, B. Qiu and L. Guo, Anal. Chem., 2016, 88, 3227–3234 CrossRef CAS PubMed.
- H. M. Nguyen, R. L. Vessella, C. Morrissey, L. G. Brown, I. M. Coleman, C. S. Higano, E. A. Mostaghel, X. Zhang, L. D. True and H. M. Lam, Prostate, 2017, 77, 654–671 CrossRef CAS.
- E. J. Small, R. S. Lance, T. A. Gardner, L. I. Karsh, L. Fong, C. McCoy, T. DeVries, N. A. Sheikh, D. GuhaThakurta and N. Chang, Clin. Cancer Res., 2015, 21, 3862–3869 CrossRef CAS.
- S. Negoita, E. J. Feuer, A. Mariotto, K. A. Cronin, V. I. Petkov, S. K. Hussey, V. Benard, S. J. Henley, R. N. Anderson and S. Fedewa, Cancer, 2018, 124, 2801–2814 CrossRef.
- G. Frens, Nat. Phys. Sci., 1973, 241, 20–22 CrossRef CAS.
- T. Ming, L. Zhao, Z. Yang, H. Chen, L. Sun, J. Wang and C. Yan, Nano Lett., 2009, 9, 3896–3903 CrossRef CAS.
- L. Guo, G. Chen and D. Kim, Anal. Chem., 2010, 82, 5147–5153 CrossRef CAS.
- S. Zanarini, E. Rampazzo, L. Ciana, M. Marcaccio, E. Marzocchi, M. Montalti, F. Paolucci and L. Prodi, J. Am. Chem. Soc., 2009, 131, 2260–2267 CrossRef CAS.
- S. Gupta, P. K. Kilpatrick, E. Melvin and O. D. Velev, Lab Chip, 2012, 12, 4279–4286 RSC.
- J. TANG, D. Tang, B. Su, Q. Li, B. Qiu and G. Chen, Analyst, 2011, 136, 3869–3871 RSC.
- E. Spain, S. Carrara, K. Adamson, H. Ma, R. O'Kennedy, L. De Cola and R. J. Forster, ACS Omega, 2018, 3, 17116–17124 CrossRef CAS.
- H. Huang and J. J. Zhu, Biosens. Bioelectron., 2009, 25, 927–930 CrossRef CAS.
- D. M. Rissin, C. W. Kan, T. G. Campbell, S. C. Howes, D. R. Fournier, S. Linan, P. Tomasz, P. P. Patel, C. Lei and A. J. Rivnak, Nat. Biotechnol., 2010, 28, 595–599 CrossRef CAS.
- J. Zhou, N. Gan, T. Li, F. Hu, X. Li, L. Wang and L. Zheng, Biosens. Bioelectron., 2014, 54, 199–206 CrossRef CAS.
|
This journal is © The Royal Society of Chemistry 2020 |
Click here to see how this site uses Cookies. View our privacy policy here.