DOI:
10.1039/C9TX00142E
(Paper)
Toxicol. Res., 2019,
8, 972-978
Mice with a Sertoli cell-specific knockout of the Ctr1 gene exhibit a reduced sensitivity to cisplatin-induced testicular germ cell apoptosis
Received
11th June 2019
, Accepted 14th October 2019
First published on 8th November 2019
Abstract
Exposure to the chemotherapeutic agent cis-diamminedichloroplatinum(II) (cDDP) is well known to instigate acute and prolonged testicular injury in male patients. Many investigators have hypothesized that cDDP-induced dysfunction of Sertoli cells (SCs) may, in part, account for the cDDP-induced lasting testicular injury. Nevertheless, the relative contribution of cDDP-induced SC injury versus direct effects on germ cells (GCs) to the pathogenesis of GC loss remains to be elucidated. The expression of the copper transporter 1 (CTR1) protein in cells directly corresponds with cDDP uptake and its cellular toxicity. Therefore, to discern the role of SCs in the pathogenic mechanism, mice were developed with a SC-specific disruption of the Ctr1 gene (SCΔCtr1) as a strategy to prevent their exposure to cDDP. Adult mice at postnatal day (PND) 60 were treated with 5 mg kg−1 cDDP and then testis collected at 48 hours. A two-fold increase in GC-apoptosis occurred in the testis of cDDP-treated wildtype (WT) mice as compared to saline-treated WT mice. In contrast, cDDP-treated SCΔCtr1 mice exhibited only a half-fold increase in GC-apoptosis as compared to the saline-treated SCΔCtr1 mice. This reduced incidence of GC apoptosis in the SCΔCtr1 mice corresponded to a significantly lower level of platinum within the testis. Taken together, these findings reveal that the uptake of cDDP by CTR1 in SCs accounts for the accumulation of cDDP in the testis and plays a pivotal role in the pathogenic sequence of events leading to the loss of germ cells via apoptosis.
Introduction
Cis-diamminedichloroplatinum(II) (cisplatin, cDDP) is a DNA cross-linking anti-neoplastic agent that is widely used in the treatment of various types of cancer including carcinoma, sarcoma, and lymphoma.1 Testicular carcinoma, in particular, is effectively eliminated with cDDP-based chemotherapy and curable even at advanced stages of metastasis.2,3 The main mechanism of cDDP cytotoxicity is its covalent binding to DNA that ultimately leads to the cellular apoptotic processes responsible for the elimination of the damaged cells.1,4 Despite its efficiency in treating certain tumors, cDDP therapy can be hindered by acquired tumor resistance as well as dose-limiting toxicities including long-lasting testicular dysfunction in male patients.5 Depending on the cumulative cDDP dosage, testicular cancer patients may experience several years of azoospermia or oligospermia and, in some cases, a prolonged state of infertility with no spermatogenic recovery following their successful chemotherapy treatments.6 Since patients that are undergoing cDDP based chemotherapy for testicular cancer are mostly young men in their prime reproductive age (ages 15–44), one of the major concerns is a prolonged disruption of their fertility.7,8 Therefore, the development of therapeutic strategies to minimize cDDP-induced testicular injury while still allowing for the efficient elimination of tumor cells is critical for the quality of life of men after chemotherapy.
Spermatogenesis is a complex process that takes place in the seminiferous epithelium, which is composed of germ cells (GCs) and Sertoli cells (SCs). The somatic SCs serve as “nurse” cells that are responsible for GC protection, nutrition, proliferation, differentiation, and death.9 Tight junctions between SCs (blood-testis barrier, BTB) prevents the free transport of molecules, leukocytes or harmful substances to reach the GCs, the meiotic spermatocytes, haploid spermatids, and sperm, that reside on the adluminal side of the BTB.9 Various transporters are expressed by SCs to regulate the influx and efflux of both nutrients and toxicants within the adluminal compartment and to create a suitable microenvironment that allows for functional spermatogenesis.10
Apoptosis of both tumor and normal cell types as a result of cDDP treatment have been well documented.1,11,12 However, the cellular mechanism(s) that account for the lasting dysfunction of spermatogenesis after cDDP treatment has ended is not well defined. Whether the cDDP-induced impairment of spermatogenesis and GC apoptosis is a direct result of cDDP exposure to the GCs or results from secondary effects of cDDP on SCs remains unclear. Animal models exposed to acute cDDP exposures show a loss of spermatocytes and a decrease in the secretion of factors important for functional spermatogenesis including transferrin, inhibin-B, and androgen-binding protein.13–17 Furthermore, sub-chronic cDDP exposure of mice results in prolonged spermatogenic abnormalities, disruption of the BTB, and sustained seminiferous tubule atrophy that lack of differentiating GCs even with the presence of spermatogonial cells.18–20 Altogether, these observations suggest that injury to the SC function may play a critical role in the pathogenic process underlying the observed long-lasting cDDP-induced testis dysfunction.
The copper (Cu) transporter 1 (CTR1, SLC31A1) protein is well-known to play a pivotal role in facilitating the uptake of cDDP into cells that allows for intracellular levels that ultimately result in its toxicity.21,22 CTR1 functions as a major Cu importer in eukaryotic cells and is highly conserved in all organisms from yeast to human.23,24 Autopsy of cDDP resistant tumor cells that have reduced intracellular levels of cDDP is associated with reduced expression of CTR1.22,25–28 In addition to tumor cells, the association of CTR1 and cDDP sensitivity is also evident in normal cells including hepatic and neuronal cells.29–31 Therefore, increases in CTR1 expression levels in cells of various tissues are observed to be more sensitive to cDDP toxicity as a result of the increased accumulation of intracellular cDDP.22,25–31 In addition, it should be noted that CTR2, an ortholog of CTR1, has been described and was originally proposed to function as a transporter.32In vitro studies have demonstrated that the CTR2 protein is primarily localized on the endosomal vesicles.33 Although in vitro studies have shown a close correlation between CTR2 expression and reduced cDDP sensitivity, CTR2 appears to mediate this sensitivity indirectly through the modification of CTR1 activity rather than directly facilitating the uptake of cDDP.34 The mRNA expression level of Ctr2 in the testis of mice testis have only been briefly described,35 however the physiological function of CTR2 in testis is not understood.
Previously, we have reported that CTR1 is highly expressed in testis and is localized on SCs which suggests that CTR1 may play a role in cDDP induced testicular injury.36 To test the role of CTR1 in SCs we developed mice with a SC-specific disruption of the Ctr1 gene (SCΔCtr1). Here we use the SCΔCtr1 mice to specifically assess the contribution of SCs in the uptake of cDDP into the seminiferous epithelium and its importance for the sensitivity of GCs to undergo cDDP-induced apoptosis. The SCΔCtr1 mice are indistinguishable from their WT littermates in gross morphological appearance, are fertile and sire a comparable number of pups as compared to WT male mice.36 Analysis of the testis of SCΔCtr1 mice after an acute cDDP dosing paradigm was performed and compared to their WT littermates. Results obtained in this study demonstrate that the uptake of cDDP into SCs through a CTR1 mediated mechanism is a key determinant for the sensitivity of GC to cDDP-induced GC apoptosis.
Materials & methods
Experimental animals
SC-specific knockout mice (SCΔCtr1) and their wild-type littermates (WT) were generated and maintained as previously described in Ghaffari et al.36SCΔCtr1 and WT mice were used for the experiments described below. Mice colonies were kept in a controlled temperature (23 °C ± 1 °C) and light (12 L:12 D) environment. Standard chow (5LL2, Purina Mills Lab-Diet, St Louis, MO) and tap water were supplied ad libitum. All procedures were performed in accordance with established guidelines and approval from The University of Texas at Austin's Institutional Animal Care and Use Committee (Protocol ID: AUP-2015-00198). After each experiment, mice were either euthanized by CO2 asphyxiation followed by cervical dislocation for histology and TUNEL analysis or, underwent blood perfusion as previously described in Stermer et al. for platinum measurements.37 Both testes were rapidly removed and weighed, with one testis flash-frozen in liquid N2 and stored at −80 °C and the other fixed in Bouin's solution (R1121000, RICCA Chemical Company) and embedded in paraffin for histological purposes as described below.
Treatment method
For acute cDDP treatment, WT and SCΔCtr1 mice at postnatal day (PND) 60 were treated with a single intraperitoneal (i.p.) injection of 5 mg kg−1 of cDDP dissolved in 0.9% normal saline. This dosing regimen utilized for these studies was chosen to be consistent with our, and others, previously published reports using an acute cDDP treatment paradigm.13,14,16,38 The control group (WT and SCΔCtr1) were treated with an equivalent volume of 0.9% saline for the same time period. Testes were collected 48 hours following cDDP treatment, a time period prior to the peak incident of GC apoptosis.13
Histology
Testes were fixed in Bouin's solution overnight at room temperature and were then washed in lithium-saturated 70% ethanol, and embedded in paraffin. Paraffin-embedded testes were sectioned to a thickness of 5 μm. Sections were then deparaffinized and rehydrated in a graded series of ethanol solutions in order to perform histology. Morphological analyses were performed on testis cross-sections stained with periodic acid-Schiff-hematoxylin (PAS-H) and mounted on glass slides according to standard protocols.18
TUNEL assay for apoptosis
Terminal deoxynucleotidyl transferase dUTP nick end labeling (TUNEL) staining was used to assess GC apoptosis in paraffin-embedded testis cross-sections. The TUNEL assay was performed using the ApopTag Peroxidase In Situ Apoptosis Detection Kit (S7100, EMD Millipore). The apoptotic index (AI) was calculated as the percentage of essentially round seminiferous tubules cross-sections containing > 3 TUNEL-positive GCs.39 More than 100 seminiferous tubules per cross-section were quantified per animal. A total of 8–21 animals per genotype per treatment groups were analyzed.
Total metal measurements
Testes were collected, as described above, and weighed into acid-washed vials (175-54, Savillex) and dried overnight at 85 °C. Dried tissues were then digested overnight into 1 ml of trace-analysis grade nitric acid (225711, Sigma) at 85 °C. Tissue samples were brought to room temperature and reduced nitric acid concentration to 2% nitric acid with ultra-pure water (10977-015, Life Technologies). Samples were then sent to the inductively coupled plasma-mass spectrometry (ICP-MS) core facility of the UT-Austin Jackson School of Geosciences for analysis.
Statistical analyses
Statistical analyses were performed utilizing GraphPad Prism version 7.03 for Windows. Data are presented as the means ± SD or SEM and analyzed using unpaired t-tests corrected for multiple comparisons using the Holm-Sidak method or the Mann–Whitney method for sample numbers less than 10. Statistical significance was considered when p < 0.05 unless otherwise stated.
Results
SC
ΔCtr1 mice do not exhibit enhanced GC apoptosis after acute cDDP treatment
Previous studies have shown that mice treated with a single high dose (5 mg kg−1) of cDDP resulted in an elevated GC apoptosis that peaked around 36 to 48 hours post-injection.13 Thus, to examine the role of CTR1 expression by SCs in cDDP induced GC apoptosis, adult SCΔCtr1 and WT mice at PND 60 were treated with single i.p. injection of 5 mg kg−1 cDDP or an equivalent volume of 0.9% normal saline as a control treatment. At 48 hours post-treatment, testes were collected from all treatment groups from both WT and SCΔCtr1 mice and analyzed for histology, tissue weights and GC apoptosis using TUNEL assay.
Testis weight, testis to bodyweight ratios and histological changes between all experimental groups, WT and SCΔCtr1 treated and untreated mice were examined. The average testis weight and testis to body weight remained unchanged in the cDDP treated mice from untreated mice following 48 hours of both WT and SCΔCtr1 mice, as shown in Fig. 1A and B. Two days following acute exposure of cDDP, both WT and SCΔCtr1 mice did not display obvious morphological abnormalities in the seminiferous tubules from saline-treated mice, as shown in Fig. 1C–F. However, multiple incidences of condensed GC nuclei characteristic of cells undergoing apoptosis could be observed within the seminiferous epithelium and prompted us to specifically quantify GC apoptosis using the TUNEL assay. The incidence of TUNEL-positive apoptotic GCs is presented as the apoptotic index (AI) as described in the method section. The cDDP treated WT mice displayed a two-fold significant increase in AI as compared to the saline-treated (control) WT mice (average AI of 2.4 in saline versus cDDP treated AI of 5.7 in WT mice), shown in Fig. 2. Interestingly, cDDP treated SCΔCtr1 mice only displayed a half-fold increase in AI from control SCΔCtr1 mice (average AI of 2.9 in saline-treated mice compared to AI of 4.5 in cDDP treated SCΔCtr1 mice), as illustrated in Fig. 2. Therefore, loss of CTR1 in SCs reduces GC apoptosis following cDDP exposure. TUNEL-positive apoptotic GCs localization was evaluated within seminiferous cross-sections. Apoptotic GCs in both cDDP treated WT and SCΔCtr1 mice testes were found at both the along the basal and the adluminal compartment of the seminiferous epithelium as shown in Fig. 3C and D.
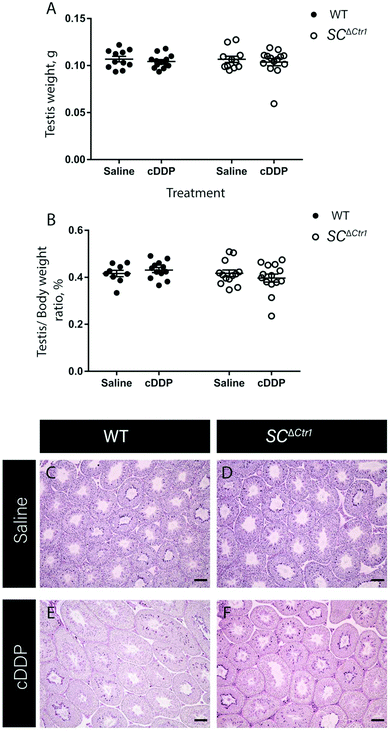 |
| Fig. 1
SC
ΔCtr1 mice do not exhibit enhanced germ cell loss after acute cDDP treatment. Testis weight (A) and testis to body weight ratio (B) from cDDP treated and saline-treated (control) groups of both WT and SCΔCtr1 mice were analyzed (average ± SD for each genotype N = 8–23). Histological analysis was performed using PAS&H staining on control WT (C) and SCΔCtr1 (D) and cDDP treated WT (E) and SCΔCtr1 (F) mice testis. | |
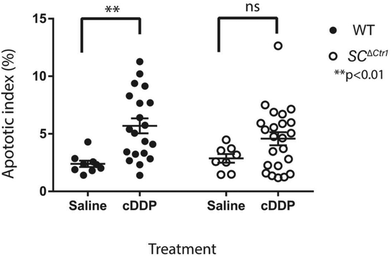 |
| Fig. 2
SC
ΔCtr1 mice do not exhibit a significant increase in germ cell loss after acute cDDP treatment. The graph represents the average apoptotic index (AI) of all treatment groups; each point represents individual animals. A two-fold increase in AI of cDDP treated WT mice compared to saline-treated control WT. Only half fold increase in AI was displayed in cDDP treated SCΔCtr1 mice when compared to saline-treated control SCΔCtr1 mice (average ± SEM for each genotype N = 8–23, **p < 0.01). | |
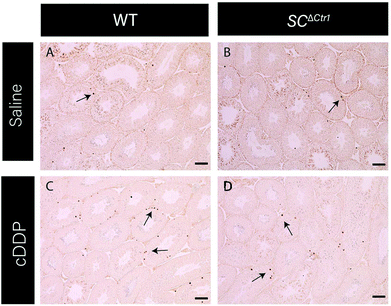 |
| Fig. 3
SC
ΔCtr1 mice do not exhibit enhanced germ cell loss after acute cDDP treatment. Testis cross-sections stained for TUNEL analysis representing each treatment groups (A–D). Arrows indicating TUNEL-positive apoptotic germ cells. Scale bar = 100 μm. | |
SC
ΔCtr1 mice testis displayed lower tissue platinum level than WT mice with no changes in tissue Cu level
To determine whether there were changes in cDDP accumulation in testis, the levels of platinum in the testis of treated mice were measured. Testes from both cDDP treated WT and SCΔCtr1 mice were randomly selected and prepared to examine the total platinum concentration using ICP-MS which corresponds to total tissue cDDP level. The average platinum level in the perfused testis of treated WT mice was significantly higher when compared to SCΔCtr1 treated testis, with WT testis having an average of 2.7 μg g−1 of dry tissue weight compared to SCΔCtr1 mice with an average 0.9 μg g−1 (Fig. 4A).
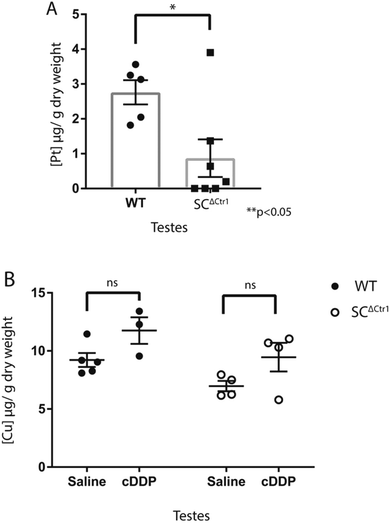 |
| Fig. 4
SC
ΔCtr1 mice testis display lower tissue platinum levels than WT mice with no changes in steady state tissue copper levels. Both WT and SCΔCtr1 cDDP-treated mice were randomly selected and analyzed for tissue platinum levels. The graph (A) represents total tissue platinum concentration in perfused mice. An increase of platinum accumulation was observed in WT testis as compared to SCΔCtr1 mice testis (average ± SEM, for each genotype N = 3–7, *p < 0.05). (B) Cu was measured in cDDP and saline-treated control mice testis of each genotype (average ± SEM, N = 3–5). | |
Given that both Cu and cDDP utilize CTR1 to enter the cells, we examined whether acute cDDP treatment would alter total testicular Cu level or whether toxicity observed in these mice is due to altered Cu levels. Thus, cDDP treated and untreated mice from WT and SCΔCtr1 mice were analyzed to determine testicular Cu level. Saline-treated SCΔCtr1 mice testes had a 30% reduced steady-state Cu level than WT mice testes (Fig. 4B), consistent with previously reported observations.36 However, following cDDP treatment, the steady-state Cu level of both WT and SCΔCtr1 mice did not exhibit significant changes (Fig. 4B). This observation suggests that acute cDDP treatment does not disrupt testis Cu homeostasis, which is in agreement with previous reports demonstrating that Cu and cDDP have district transport mechanism by CTR1.40,41
Discussion
Despite the growing body of knowledge on the cytotoxic mechanisms of cDDP in tumor cells, the cellular sequence of events that account for the lasting testicular injury after cDDP treatment remains unclear.1,6 An increased accumulation of cDDP in both normal and cancer cells has been implicated as one of the mechanisms underlying the cell's sensitivity to cDDP toxicity.42 Accordingly, cells with elevated levels of Ctr1 expression show an enhanced sensitivity to cDDP whereas cDDP resistant cell types often show low levels of Ctr1 expression.25,26,43 Although in vivo studies have demonstrated a positive correlation of Ctr1 expression levels to cellular cDDP sensitivity,25,26,43 there is a lack of sufficient in vivo studies that adequately examine cDDP uptake and sensitivity in relation to the Ctr1 expression levels.
Previously, we hypothesized that the pathogenic mechanism leading to cDDP-induced GC apoptosis involves, in part, the SC rather exclusively being dependent on the direct effects of cDDP on the GCs themselves.13,18,20 However, the extensive interactions amongst the cells of the testis have made it challenging to dissect the in vivo contribution of the SC in the sequence of events leading to cDDP-induced GC apoptosis.18,19,44,45 To address this challenge, mice with a SC-specific knockout of the Ctr1 gene were generated as a strategy to the delineate the relative contribution of the SCs on cDDP-induced GC apoptosis. Collectively, the results reported here support the notion that cDDP-induced GC apoptosis is dependent upon the expression of the CTR1 protein by SCs.
The tight correlation between the reduced incidence of cDDP-induced GC apoptosis (Fig. 2 & 3) and decreased levels of platinum in the testis of in SCΔCtr1 mice (Fig. 4) strongly implicates the CTR1 protein in the transport of cDDP into the seminiferous epithelium through the SCs. However, from these data, it cannot be determined if the reduced cDDP-induced GC apoptosis is due soley to the inability of cDDP to transport through the SC and into the adluminal compartment where the meiotic GC subtypes reside or whether the protection of SC function from cDDP-induced disruption also accounts for some of the observed protection against GC apoptosis. Nevertheless, although alterations in SC function have been noted others investigators,13–17 whether these changes instigate GC apoptosis directly are not known. On-going studies are now targeted to characterize the differential role in the testis of the SCΔCtr1 mice between the prevention of the accumulation of cDDP in the adluminal compartment versus the possible contribution from the protection against SC injury.
Although there was a significant reduction in average platinum levels in the testis of SCΔCtr1 mice (Fig. 4), a low amount of platinum was measured within the testis of these mice. It is possible that this low level of cDDP in the testis could exist in the interstitial compartment and affect cells such as the Leydig cells, or that a low levels of cDDP can make it into the SC via a different transporter. Since CTR1 is specifically knocked out in SCs (and not other cells such as Leydig cells), it is possible that uptake of cDDP into Leydig cells could possibly alter the extent of GC apoptosis in both WT and SCΔCtr1 mice by modifying testosterone levels. However, studies have shown that testosterone levels following cDDP treatments of both male rats and human patients did not display significant changes in testosterone levels14,46 making it unlikely that this is the case. The organic cation transporter 2 (OCT2) is has been associated with cDDP transport across the cell and could be considered as a mechanism to account for the observed low level of cDDP in the testis of the SCΔCtr1 mice. However, the expression OCT2 in SCs has not yet been established.47,48 Nevertheless, the marked reduction of platinum levels in the testis of the SCΔCtr1 mice does indicate that CTR1 is predominantly responsible for the mechanism for the uptake of cDDP into the seminiferous epithelium and that alternative transporters only play a minor role after an acute exposure to cDDP.
Both Cu and cDDP utilize the same transporter protein, CTR1, to enter the cell. Unlike Cu which gets transported through the CTR1 pore, cDDP is believed to bind to the CTR1 protein and enter the cell through endocytosis of CTR1.26,49 In this way, CTR1 acts as a receptor for cDDP uptake.41 In addition, it is believed that CTR1 is utilized by both Cu and cDDP in a non-competitive manner.26,49,50Testicular Cu levels of all treatment groups and genotypes were examined as to whether cDDP exposure alters testicular Cu levels. Consistent with this notion, our observations revealed that steady state Cu level in testis of both genotypes (WT and SCΔCtr1) remains unchanged followed by acute cDDP exposure in mice. This suggests that acute cDDP exposure does not affect testicular Cu levels in vivo.
Conclusion
Collectively, the findings of the present study reveal that the uptake of cDDP via CTR1 expressed by SCs is an important determinant for cDDP-induced GC loss by apoptosis. These observations provide foundational knowledge that could be utilized for in the development of novel therapeutic strategies to allow for the protection against the lasting testicular injury that occurs as a result of cDDP-based chemotherapy.
Funding
This work was supported, in part, by the National Institute of Health (2 R01ES016591; J. H. R.). Funding in the form of graduate research assistantship or teaching assistantship from the University of Texas at Austin was provided to R. G. The Funders have no role in study design, data collection, and analysis, the decision to publish or preparation of the manuscript. No commercial funding supported this work.
Conflicts of interest
There are no conflicts of interest to declare.
Acknowledgements
We thank Dr Nathaniel Miller (Jackson School of Geosciences, University of Texas, Austin) for performing all inductively coupled plasma-mass spectrometry assays reported in this manuscript.
References
- L. Kelland, The resurgence of platinum-based cancer chemotherapy, Nat. Rev. Cancer, 2007, 7, 573–584, DOI:10.1038/nrc2167.
- N. H. Hanna and L. H. Einhorn, Testicular Cancer—Discoveries and Updates, N. Engl. J. Med., 2014, 371(21), 2005–2016, DOI:10.1056/NEJMra1407550.
- D. C. Spierings, E. G. de Vries, E. Vellenga and S. de Jong, The attractive Achilles heel of germ cell tumours: an inherent sensitivity to apoptosis-inducing stimuli, J. Pathol., 2003, 200, 137–148, DOI:10.1002/path.1373.
- Y. Jung and S. J. Lippard, Direct Cellular Responses to Platinum-Induced DNA Damage, Chem. Rev., 2007, 107, 1387–1407, DOI:10.1021/cr068207j.
- S. J. Howell, Spermatogenesis After Cancer Treatment: Damage and Recovery, J. Natl. Cancer Inst. Monogr., 2005, 2005, 12–17, DOI:10.1093/jncimonographs/lgi003.
- S. J. Howell and S. M. Shalet, Testicular function following chemotherapy, Hum. Reprod. Update, 2001, 7, 363–369 CrossRef CAS PubMed.
- A. B. Thomson, H. O. D. Critchley, C. J. H. Kelnar and W. H. B. Wallace, Late reproductive sequelae following treatment of childhood cancer andoptions for fertility preservation, Best Pract. Res., Clin. Endocrinol. Metab., 2002, 16, 311–334, DOI:10.1053/beem.2002.0200.
- W. H. B. Wallace, Oncofertility and preservation of reproductive capacity in children and young adults, Cancer, 2011, 117, 2301–2310, DOI:10.1002/cncr.26045.
- C. Y. Cheng and D. D. Mruk, The Blood-Testis Barrier and Its Implications for Male Contraception, Pharmacol. Rev., 2011, 64, 16–64, DOI:10.1124/pr.110.002790.
- L. Su, D. D. Mruk and C. Y. Cheng, Drug transporters, the blood-testis barrier and spermatogenesis, J. Endocrinol., 2010, 1–17, DOI:10.1677/JOE-10-0363.
- R. Oun, Y. E. Moussa and N. J. Wheate, The side effects of platinum-based chemotherapy drugs: a review for chemists, Dalton Trans., 2018, 47(19), 6645–6653, 10.1039/C8DT00838H.
- V. M. Gonzalez, M. A. Fuertes, C. Alonso and J. M. Perez, Is cisplatin-induced cell death always produced by apoptosis?, Mol. Pharmacol., 2001, 59, 657–663 CrossRef CAS PubMed.
- F. Seaman, P. Sawhney, C. J. Giammona and J. H. Richburg, Cisplatin-induced pulse of germ cell apoptosis precedes long-term elevated apoptotic rates in C57/BL/6 mouse testis, Apoptosis, 2003, 8, 101–108 CrossRef CAS PubMed.
- H. F. Huang, L. M. Pogach, E. Nathan and W. Giglio, Acute and chronic effects of cisplatinum upon testicular function in the rat, J. Androl., 1990, 11, 436–445 CAS.
- P. Köpf-Maier, Effects of carboplatin on the testis. A histological study, Cancer Chemother. Pharmacol., 1992, 29, 227–235 CrossRef PubMed.
- X. Zhang, N. Yamamoto, S. Soramoto and I. Takenaka, Cisplatin-induced germ cell apoptosis in mouse testes, Arch. Androl., 2001, 46, 43–49 CrossRef CAS PubMed.
- K. Yamaguchi, T. Ishikawa, Y. Kondo and M. Fujisawa, Cisplatin regulates Sertoli cell expression of transferrin and interleukins, Mol. Cell. Endocrinol., 2008, 283, 68–75, DOI:10.1016/j.mce.2007.11.021.
- P. Sawhney, C. J. Giammona, M. L. Meistrich and J. H. Richburg, Cisplatin-induced long-term failure of spermatogenesis in adult C57/Bl/6J mice, J. Androl., 2005, 26, 136–145 CAS.
- D. Mohammadnejad, A. Abedelahi, J. Soleimani-Rad, A. Mohammadi-Roshandeh, M. Rashtbar and A. Azami, Degenerative effect of Cisplatin on testicular germinal epithelium, Adv. Pharm. Bull., 2012, 2, 173–177, DOI:10.5681/apb.2012.026.
- J. G. Harman and J. H. Richburg, Cisplatin-induced alterations in the functional spermatogonial stem cell pool and niche in C57/BL/6J mice following a clinically relevant multi-cycle exposure, Toxicol. Lett., 2014, 227(2), 99–112, DOI:10.1016/j.toxlet.2014.03.019.
- S. Ishida, J. Lee, D. J. Thiele and I. Herskowitz, Uptake of the anticancer drug cisplatin mediated by the copper transporter Ctr1 in yeast and mammals, Proc. Natl. Acad. Sci. U. S. A., 2002, 99(22), 14298–14302, DOI:10.1073/pnas.162491399.
- E. S. Kim, X. Tang, D. R. Peterson, D. Kilari, C.-W. Chow and J. Fujimoto,
et al., Copper transporter CTR1 expression and tissue platinum concentration in non-small cell lung cancer, Lung Cancer, 2014, 85(1), 88–93, DOI:10.1016/j.lungcan.2014.04.005.
- J. Lee, J. R. Prohaska and D. J. Thiele, Essential role for mammalian copper transporter Ctr1 in copper homeostasis and embryonic development, Proc. Natl. Acad. Sci. U. S. A., 2001, 98(12), 6842–6847, DOI:10.1073/pnas.111058698.
- S. Puig, J. Lee, M. Lau and D. J. Thiele, Biochemical and Genetic Analyses of Yeast and Human High Affinity Copper Transporters Suggest a Conserved Mechanism for Copper Uptake, J. Biol. Chem., 2002, 277, 26021–26030, DOI:10.1074/jbc.M202547200.
- M. C. Akerfeldt, C. M.-N. Tran, C. Shen, T. W. Hambley and E. J. New, Interactions of cisplatin and the copper transporter CTR1 in human colon cancer cells, J. Biol. Inorg. Chem., 2017, 22, 765–774, DOI:10.1007/s00775-017-1467-y.
- C. A. Larson, P. L. Adams, D. D. Jandial, B. G. Blair, R. Safaei and S. B. Howell, The role of the N-terminus of mammalian copper transporter 1 in the cellular accumulation of cisplatin, Biochem. Pharmacol., 2010, 80(4), 448–454, DOI:10.1016/j.bcp.2010.04.030.
- C. A. Larson, B. G. Blair, R. Safaei and S. B. Howell, The Role of the Mammalian Copper Transporter 1 in the Cellular Accumulation of Platinum-Based Drugs, Mol. Pharmacol., 2009, 75, 324–330, DOI:10.1124/mol.108.052381.
- X. Xu, H. Ren, B. Zhou, Y. Zhao, R. Yuan and R. Ma,
et al., Prediction of copper transport protein 1 (CTR1) genotype on severe cisplatin induced toxicity in non-small cell lung cancer (NSCLC) patients, Lung Cancer, 2012, 77(2), 438–442, DOI:10.1016/j.lungcan.2012.03.023.
- N. Pabla, R. F. Murphy, K. Liu and Z. Dong, The copper transporter Ctr1 contributes to cisplatin uptake by renal tubular cells during cisplatin nephrotoxicity, Am. J. Physiol. Renal. Physiol., 2009, 296, F505–F511, DOI:10.1152/ajprenal.90545.2008.
- J. J. Liu, S. M. F. Jamieson, J. Subramaniam, V. Ip, N. N. Jong and J. F. B. Mercer,
et al., Neuronal expression of copper transporter 1 in rat dorsal root ganglia: association with platinum neurotoxicity, Cancer Chemother. Pharmacol., 2009, 64, 847–856, DOI:10.1007/s00280-009-1017-6.
- S. Waissbluth, J. Pitaro and S. J. Daniel, Gene therapy for cisplatin-induced ototoxicity: a systematic review of in vitro and experimental animal studies, Otol Neurotol., 2012, 33, 302–310, DOI:10.1097/MAO.0b013e318248ee66.
- B. L. Logeman, L. K. Wood, J. Lee and D. J. Thiele, Gene duplication and neo-functionalization in the evolutionary and functional divergence of the metazoan copper transporters Ctr1 and Ctr2, J. Biol. Chem., 2017, 292, 11531–11546, DOI:10.1074/jbc.M117.793356.
- P. V. E. van den Berghe, D. E. Folmer, H. E. M. Malingré, E. van Beurden, A. E. M. Klomp and B. van de Sluis,
et al., Human copper transporter 2 is localized in late endosomes and lysosomes and facilitates cellular copper uptake, Biochem. J., 2007, 407, 49–59, DOI:10.1042/BJ20070705.
- H. Öhrvik, Y. Nose, L. K. Wood, B.-E. Kim, S.-C. Gleber and M. Ralle,
et al., Ctr2 regulates biogenesis of a cleaved form of mammalian Ctr1 metal transporter lacking the copper- and cisplatin-binding ecto-domain, Proc. Natl. Acad. Sci. U. S. A., 2013, 110(46), E4279–E4288, DOI:10.1073/pnas.1311749110.
- M. Ogórek, M. Lenartowicz, R. Starzyński, A. Jończy, R. Staroń and A. Doniec,
et al., Atp7a and Atp7b regulate copper homeostasis in developing male germ cells in mice, Metallomics, 2017, 9(9), 1288–1303, 10.1039/c7mt00134g.
- R. Ghaffari, K. R. Di Bona, C. L. Riley and J. H. Richburg, Copper transporter 1 (CTR1) expression by mouse testicular germ cells, but not Sertoli cells, is essential for functional spermatogenesis, PLoS One, 2019, 14(4), e0215522–e0215522, DOI:10.1371/journal.pone.0215522.
- A. R. Stermer, C. J. Murphy, R. Ghaffari, K. R. Di Bona, J. J. Voss and J. H. Richburg, Mono-(2-ethylhexyl) phthalate-induced Sertoli cell injury stimulates the production of pro-inflammatory cytokines in Fischer 344 rats, Reprod. Toxicol., 2017, 69, 150–158, DOI:10.1016/j.reprotox.2017.02.013.
- M. L. Meistrich, M. Finch, M. F. da Cunha, U. Hacker and W. W. Au, Damaging Effects of Fourteen Chemotherapeutic Drugs on Mouse Testis Cells, Cancer Res., 1982, 42, 122–131 CAS.
- J. Lee, J. H. Richburg, S. C. Younkin and K. Boekelheide, The Fas system is a key regulator of germ cell apoptosis in the testis, Endocrinology, 1997, 138, 2081–2088, DOI:10.1210/endo.138.5.5110.
- Y. Guo, K. Smith and M. J. Petris, Cisplatin Stabilizes a Multimeric Complex of the Human Ctr1 Copper Transporter, J. Biol. Chem., 2004, 279, 46393–46399, DOI:10.1074/jbc.M407777200.
- D. Sinani, D. J. Adle, H. Kim and J. Lee, Distinct Mechanisms for Ctr1-mediated Copper and Cisplatin Transport, J. Biol. Chem., 2007, 282, 26775–26785, DOI:10.1074/jbc.M703973200.
- D. Kilari, Role of copper transporters in platinum resistance, World J. Clin. Oncol., 2016, 7, 106–109, DOI:10.5306/wjco.v7.i1.106.
- K. Katano, A. Kondo, R. Safaei, A. Holzer, G. Samimi and M. Mishima,
et al., Acquisition of resistance to cisplatin is accompanied by changes in the cellular pharmacology of copper, Cancer Res., 2002, 62, 6559–6565 CAS.
- L. M. Pogach, Y. Lee, S. Gould and W. Giglio, Characterization of cis-platinum-induced Sertoli cell dysfunction in rodents, Toxicol. Appl. Pharmacol., 1989, 98, 350–361, DOI:10.1016/0041-008X(89)90239-1.
- K. Boekelheide, Mechanisms of Toxic Damage to Spermatogenesis, J. Natl. Cancer Inst. Monogr., 2005, 2005, 6–8, DOI:10.1093/jncimonographs/lgi006.
- S. W. Hansen, J. G. Berthelsen and H. Maase von der, Long-term fertility and Leydig cell function in patients treated for germ cell cancer with cisplatin, vinblastine, and bleomycin versus surveillance, J. Clin. Oncol., 1990, 8, 1695–1698, DOI:10.1200/JCO.1990.8.10.1695.
- G. Ciarimboli, D. Deuster, A. Knief, M. Sperling, M. Holtkamp and B. Edemir,
et al., Organic Cation Transporter 2 Mediates Cisplatin-Induced Oto- and Nephrotoxicity and Is a Target for Protective Interventions, Am. J. Pathol., 2010, 176, 1169–1180, DOI:10.2353/ajpath.2010.090610.
- K. K. Filipski, R. H. Mathijssen, T. S. Mikkelsen, A. H. Schinkel and A. Sparreboom, Contribution of Organic Cation Transporter 2 (OCT2) to Cisplatin-Induced Nephrotoxicity, Clin. Pharmacol. Ther., 2009, 86(4), 396–402, DOI:10.1038/clpt.2009.139.
- H. Öhrvik and D. J. Thiele, How copper traverses cellular membranes through the mammalian copper transporter 1, Ctr1, Ann. N. Y. Acad. Sci., 2014, 1314, 32–41, DOI:10.1111/nyas.12371.
- S. B. Howell, R. Safaei, C. A. Larson and M. J. Sailor, Copper Transporters and the Cellular Pharmacology of the Platinum-Containing Cancer Drugs, Mol. Pharmacol., 2010, 77, 887–894, DOI:10.1124/mol.109.063172.
|
This journal is © The Royal Society of Chemistry 2019 |