DOI:
10.1039/C9TC03066B
(Paper)
J. Mater. Chem. C, 2019,
7, 10379-10388
Effect of structural engineering of π-spacers on anti-aggregation of D–A–π–A dyes†
Received
8th June 2019
, Accepted 26th July 2019
First published on 26th July 2019
Abstract
In this paper, the encapsulated insulated molecular wire (EIMW) and 2′,7′-bis(hexyloxy)spiro[cyclopenta[2,1-b:3,4-b′]dithiophene-4,9′-fluorene] (SPDF) were specifically designed as efficient anti-aggregation π-bridges for two novel D–A–π–A metal-free sensitizers (IBT1 and IBT2). Compared with the reference dye IBT3 with 3,3′-dihexyl-2,2′-bithiophene (DHBT) as an anti-aggregation π-bridge, both the dyes IBT1 with EIMW and IBT2 with SPDF as π-bridges can suppress intermolecular aggregation more efficiently. The π-bridge of SPDF shows the strongest anti-aggregation ability due to the rigid ‘T’ configuration among these dyes. Meanwhile, the π-bridge of EIMW shows better anti-aggregation ability than DHBT because of the larger steric hindrance of the former. Finally, when the dyes IBT1 and IBT2 are co-sensitized with IBT3, respectively, the new devices exhibit high efficiencies of 7.85% and 7.64%, respectively, which are comparable to that of N719 (7.85%). The results demonstrate that the dyes with EIMW and SPDF as anti-aggregation π-bridges are promising candidates for efficient DSSCs and dye aggregation can be effectively inhibited by structural engineering of the π-spacer.
1. Introduction
Dye-sensitized solar cells (DSSCs) have attracted increasing interest over the last few decades, owing to their unique merits in terms of low-cost production, easy fabrication and good stability.1–8 DSSCs have been developed to achieve high power conversion efficiency since the work by O’Regan and Grätzel in 1991.9 The exploration of new-style photosensitizers with broad absorption spectra to achieve high power conversation efficiency (PCE) is a crucial mission for the development of energy technologies. Metal complex dyes and metal-free dyes have been developing rapidly, which significantly improve the performance of the devices,10–12 especially for metal-free sensitizers, due to the advantages of accessible raw materials, their environmentally friendly nature and highly adjustable structure.13,14 In recent years, extensive research has been directed toward the design of novel metal-free sensitizers that achieve high efficiencies.15–19 It is worth noting that a new record efficiency of 14.5% was reported in 2015 by using a cobalt-based liquid electrolyte.20
In general, donor (D)–π spacer (π)–acceptor (A) type functional dyes have been extensively used in DSSCs, owing to the strong intramolecular charge transfer (ICT) effect, the electron transfer from donor to acceptor flowing through the π-bridge smoothly.21–25 Afterwards, a new donor–auxiliary acceptor–π spacer–acceptor (D–A–π–A) configuration was developed, which incorporates an electron withdrawing auxiliary acceptor such as benzothiadiazole (BTD),26,27 benzotriazole (BTZ),28,29 quinoxaline,30–32 diketopyrrolopyrrole (DPP),33,34 isoindigo35 and 4,4-difluoro-4-bora-3a,4a-diaza-s-indacene (Bodipy).36,37 Because the new architecture (D–A–π–A) can not only narrow the band gap but also broaden the light absorption in the visible and near infrared regions, these dyes are considered as candidate sensitizers for achieving more efficient DSSCs.38–41 Cyclopenta[2,1-b:3,4-b′]dithiophene (CPDT) is extensively used as a π-bridge in the D–A–π–A architecture,42–45 because of its excellent light-harvesting performance. However, the characteristic rigid planar conformation of this architecture might form a rigid rod shape that facilitates dye aggregation after these dyes are adsorbed onto the surface of TiO2. All of the reported CPDT sensitizers adopt a conventional alkyl chain as an anti-aggregation group to inhibit dye aggregation, and unfortunately the aggregation behavior still exists,46–48 which limits further improvement of the device performance.
Dye aggregation is adverse for DSSCs because it lowers the photoelectric performance.49 Therefore, so far, there have been enormous efforts in designing anti-aggregation dyes.50–53 In our previous work, we have designed and synthesized a series of novel phenothiazine sensitizers PH1–3, where the encapsulated insulated molecular wire (EIMW) unit as an auxiliary donor was introduced to the phenothiazine-based organic dyes.54 The result indicates that the EIMW unit as an auxiliary donor can inhibit dye aggregation dramatically and result in a much higher photovoltage. However, there is still a small amount of aggregation in the dyes. When a coplanar aromatic polycycle was introduced, the new dye aggregation was aggravated significantly.
In this work, we attempted to suppress dye aggregation by π-spacer structural engineering with a ‘T’ configuration and large steric hindrance. Firstly, we introduced the EIMW unit to the D–A–π–A dye IBT1 as an anti-aggregation π-spacer with large steric hindrance (Fig. 1). Secondly, we introduced the 2′,7′-bis(hexyloxy)spiro[cyclopenta[2,1-b:3,4-b′]dithiophene-4,9′-fluorene] (SPDF) unit with a ‘T’ configuration to suppress dye aggregation in the dye IBT2. To the best of our knowledge, SPDF is applied as an anti-aggregation π-spacer for the first time. In addition, 3,3′-dihexyl-2,2′-bithiophene was introduced to achieve IBT3 as the reference dye because it is well known that alkyl chains show good anti-aggregation effects.55–58
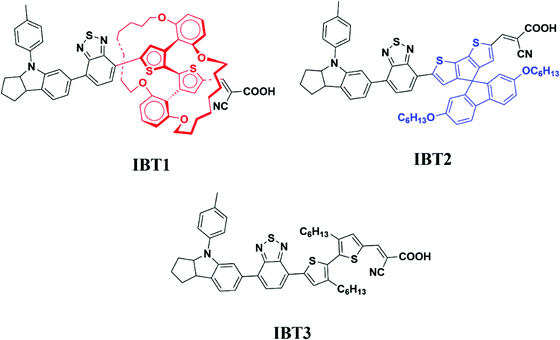 |
| Fig. 1 Chemical structures of indoline based D–A–π–A sensitizers IBT1–3. | |
2. Experimental
2.1 Materials and reagents
The raw materials were purchased from Aladdin, J&K, Energy Chemical and Adamas and were of analytical grade. Toluene, THF, 1,2-dichloroethane and 1,4-dioxane were distilled over sodium under an argon atmosphere. DMF was extra dried and stored in EnergySeal with molecular sieves. Other solvents and reagents were of analytical grade and used without further purification. The reactions were monitored by thin-layer chromatography (TLC) until the reaction was completed. Chromatographic separations were carried out on silica gel (200–300 mesh).
2.2 Instruments and characterization
Characterization 1H and 13C NMR spectra were recorded on a Bruker advance III 400 MHz spectrometer in CDCl3 and THF-d8 with tetramethylsilane as the internal standard. High-resolution mass spectrometry (HRMS) spectra were recorded on an Agilent Technologies 1290 Infinity mass spectrophotometer. Melting point was measured on an SGW X-4B microscopic melting point apparatus. The absorption spectra of dyes in solution were measured on a Shimadzu UV-2450 spectrophotometer. The absorption spectra of dyes adsorbed on the surface of TiO2 were recorded on a UV-3010 spectrophotometer. The PL spectra were recorded on a Fluorolog III photoluminescence spectrometer. The cyclic voltammetry (CV) data were measured with a CHI 660d electrochemical workstation (Chenhua Co. Ltd, Shanghai, China), using a three electrode cell at a scan rate of 50 mV s−1. The dye loaded TiO2 film was used as a working electrode, a Pt wire as auxiliary electrode, and Ag/AgCl (0.01 M) as reference electrode. The supporting electrolyte was 0.1 M tetrabutylammonium hexafluorophosphate (n-Bu4NPF6) in CH3CN solution. A ferrocenium/ferrocene (Fc/Fc+) redox couple was employed to act as an internal potential reference. The incident monochromatic photon-to-current conversion efficiency (IPCE) spectra were measured on a Spectral products DK240 monochromator in the region from 400 to 800 nm. The current–voltage characteristics were measured on a Keithley 2400 source meter under simulated AM 1.5G (100 mW cm−2) illumination with a standard solar light simulator with a mask. The electrochemical impedance spectra (EIS) were recorded on a Zahner Zennium electrochemical workstation under dark conditions.
2.3 Fabrication of dye-sensitized solar cells
The manufacturing processes of these cells were carried out according to the previous procedures.59–61 Fluorine-doped tin oxide (FTO) glass was washed with detergent, water, ethanol and acetone in an ultrasonic bath to wipe off the impurity. The thickness of the TiO2 photoanode films was controlled to about 16 μm (12 μm thick transparent layer and 4 μm thick scattering layer). The films were immersed into a 3.0 × 10−4 M solution of the dyes for 24 h (the solution is a mixture solvent of CHCl3 and ethanol (volume ratio of 1
:
1)) at 25 °C. The composition of the electrolyte is 0.6 M 1-metyl-3-propylimidazoliumiodide (PMII), 0.05 M LiI, 0.10 M guanidiniumthiocyanate, 0.03 M I2 and 0.5 M tert-butylpridine in acetonitrile/valeronitrile (85/15). For the co-adsorption, chenodeoxycholic acid (CDCA) was added into the dye solution at a concentration of 1 mM. The dye-loaded TiO2/FTO glass films were assembled into a sandwiched type configuration together with a Pt/FTO counter electrode. The active area of the dye coated TiO2 film was 0.16 cm2. For the co-sensitization experiment, the photoanodes was first immersed into a 0.3 mM solution of dye IBT1 (or IBT2) in CHCl3/CH3CH2OH (v/v, 1/1) for 24 h, rinsed with ethanol, and then immersed into a 0.3 mM solution of dye IBT3 in CHCl3/CH3CH2OH (v/v, 1/1) for 3 h.
3. Results and discussion
3.1 Synthesis
The synthetic procedures of IBT1–3 are depicted in Scheme 1. Compound 1,62 5′-bromo-3,3′-dihexyl-[2,2′-bithiophene]-5-carbaldehyde (7),63 4-(4,4,5,5-tetramethyl-1,3,2-dioxaborolan-2-yl)-7-(4-(p-tolyl)-1,2,3,3a,4,8b-hexahydrocyclopenta[b]indol-6-yl)benzo[c][1,2,5]thiadiazole (8)64 and 2′,7′-bis(hexyloxy)spiro[cyclopenta[2,1-b:3,4-b′]dithiophene-4,9′-fluorene] (SPDF) (4)65 were synthesized according to the references. Intermediates 3, 6 and 9 were synthesized via the Suzuki coupling reaction. Intermediates 5 and 8 were synthesized according to the Vilsmeier–Haack reaction and then through bromination with N-bromosuccinimide (NBS). The target products IBT1, IBT2 and IBT3 were obtained from 3, 6 and 9via the Knoevenagel condensation reaction. All of the new compounds were certified by 1H NMR, 13C NMR and HRMS or MALDI-TOF-MS.
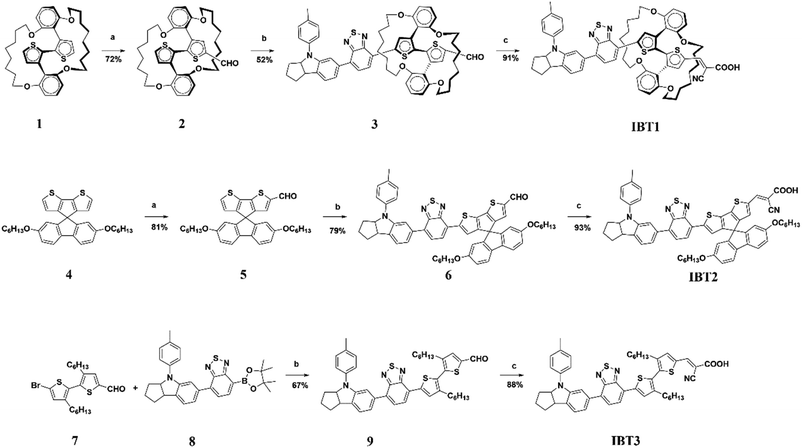 |
| Scheme 1 Synthetic routes of the dyes IBT1–3. (a) POCl3, DMF, and 1,2-dibromoethane, at 80 °C; (b) Pd(PPh3)4, Na2CO3, ethanol, H2O, and toluene, at 100 °C; (c) piperidine, cyanoacetic acid, and CHCl3, at 75 °C. | |
3.2 Photophysical properties
The UV-Vis absorption spectra of the three dyes IBT1, IBT2 and IBT3 were measured in CH2Cl2 solution (Fig. 2) and relevant characteristic data are tabulated in Table 1. Generally, the three dyes show two obvious broad absorption bands in the range of 300–750 nm. The high-energy bands located at 300–380 nm are generated by a local aromatic π–π* electronic transition of the chromophores. The low-energy bands located at 380–750 nm are produced by intramolecular charge transfer (ICT) from the indoline donor to the electron acceptor of cyanoacetic acid. The maximum absorption wavelengths of IBT1, IBT2 and IBT3 are 544, 564 and 513 nm, respectively. Compared with IBT3, IBT1 and IBT2 display a distinct red shift of 31 nm and 51 nm in the ICT absorption band, respectively; this phenomenon may be attributed to the better and better planarity obtained when EIMW and SPDF are introduced in turn. Besides, additional bands are found in the range of 400–500 nm for IBT1 and IBT2. The origin of these bands has been researched in a previously reported reference.66 Special mention should be made that dye IBT3 achieved a higher absorption in the range of 300–500 nm than IBT1 and IBT2, and this phenomenon suggests that IBT3 might be a co-sensitizer for dyes IBT1 and IBT2 to make up the absorption valley in this region.
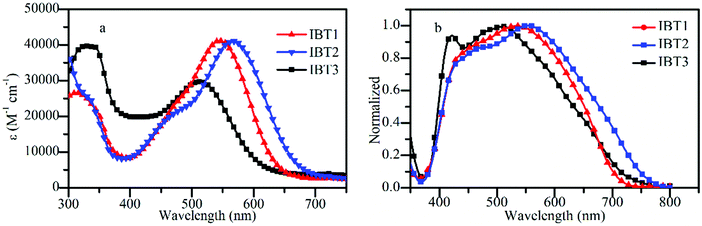 |
| Fig. 2 The UV-Vis absorption spectra of the three dyes in CH2Cl2 solution (a) and the absorption spectra on 12 μm TiO2 films (b). | |
Table 1 Optical and electrochemical data of the dyes IBT1–3
Dye |
λ
max
(nm) |
ε
(104 M−1 cm−1) |
λ
max
(nm) |
HOMOc (V) |
E
0–0
(eV) |
LUMOe (V) |
Maximum absorption peak (λmax) and molar extinction coefficient (ε) of the dyes were measured in CH2Cl2 solution.
λ
max of the dyes was measured on 12 μm TiO2 films.
Oxidation potential was measured in CH3CN containing 0.1 M (n-C4H9)4NPF6 with a scan rate of 50 mV s−1, referenced against Fc/Fc+.
E
0−0 was estimated from the normalized fluorescence and absorption curves by 1240/λ in CH2Cl2 solution.
The LUMO was calculated according to LUMO = HOMO − E0–0.
|
IBT1
|
544 |
4.11 |
532 |
0.87 |
2.13 |
−1.26 |
IBT2
|
564 |
4.10 |
554 |
0.85 |
1.96 |
−1.11 |
IBT3
|
513 |
2.97 |
505 |
0.85 |
2.18 |
−1.33 |
In addition, the three dyes achieve a high molar extinction coefficient, especially dyes IBT1 and IBT2. For dye IBT3, two alkyl chains were introduced to suppress dye aggregation and increase solubility, but a smaller molar extinction coefficient (2.97 × 104 M−1 cm−1) was obtained due to the interrupted conjugation resulting from two alkyl chains, which can be verified later by the DFT calculation. Compared to dye IBT3, IBT1 showed a higher molar extinction coefficient (4.11 × 104 M−1 cm−1) after EIMW was introduced. IBT2 with a novel π bridge SPDF shows the same high molar extinction coefficient (4.10 × 104 M−1 cm−1) as IBT1. These results suggest that both IBT1 and IBT2 possess stronger light-harvesting capability.67
The absorption spectra of IBT1–3 on 16 μm thick TiO2 films are shown in Fig. 2b and the corresponding data are listed in Table 1. The absorption spectra of these dyes are broadened in comparison with those in solution, which is beneficial for improving the light-harvesting ability. In contrast with those in solution, the maximum absorption peak (λmax) of IBT1, IBT2 and IBT3 exhibits a blue shift of 12, 10 and 8 nm, respectively. This phenomenon may be due to a deprotonation effect and dye aggregation. It is surprising that IBT2 shows the widest absorption spectrum, which demonstrates that SPDF might be a potential light-harvesting segment in the field of DSSCs.
3.3 Electrochemical properties
The thermodynamic possibility of electron injection, dye regeneration and electrochemical properties of the three dye sensitized films were determined by cyclic voltammetry in MeCN solution with 0.1 M tetrabutyl-ammonium hexafluorophosphate as the supporting electrolyte. The first oxidation potential corresponds to the highest occupied molecular orbital (HOMO) level. The cyclic voltammetry curves and schematic diagram of energy levels of all IBT sensitizers are shown in Fig. 3, and the corresponding electrochemical data are listed in Table 1. The potential was externally calibrated by the ferrocenium/ferrocene (Fc/Fc+) couple and then converted to NHE by addition of 0.63 V. The HOMO levels of the dyes IBT1, IBT2 and IBT3 are 0.87 V, 0.85 V and 0.85 V, respectively. These nearly identical values are mainly ascribed to the same donor of the dyes, because the HOMO level depends on the donor segment.68 These values are more positive than the I−/I3− redox potential value (0.4 V vs. NHE), which indicates that the oxidized dyes can be efficiently regenerated by the electrolyte. Estimated from the intersection of the normalized absorption and emission spectra, E0–0 values of IBT1, IBT2 and IBT3 are 2.13, 1.96 and 2.18 eV, respectively. The LUMO levels of dyes IBT1, IBT2 and IBT3 calculated from HOMO–E0–0 are −1.26, −1.11 and −1.33 V, respectively. The values are more negative than the conduction band (CB) level of TiO2 (−0.5 V vs. NHE), indicating that an efficient electron injection process from the excited dye into the conduction band of TiO2 can occur for the three dyes.69
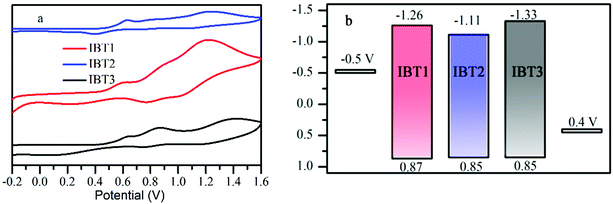 |
| Fig. 3 (a) Cyclic voltammograms of the three dye sensitized films in acetonitrile containing 0.1 M (n-C4H9)4NPF6 as the supporting electrolyte and (b) the HOMO and LUMO of the three dyes. | |
3.4 Theoretical calculations
To investigate the electron-density distributions of the three dyes, we used the hybrid B3LYP functional and the 6-31G(d) basis set to optimize the ground-state geometries of the sensitizers in the gas phase by density functional theory (DFT) simulation with the Gaussian 09 package. The optimized molecular configurations and distributions of the HOMO and LUMO orbitals of the three dyes are shown in Table 2. The optimized molecular configuration of IBT3 shows a lengthy rodlike structure, which tends to aggravate dye aggregation, therefore, leading to self-quenching and inefficient electron injection into the TiO2. It is worth noting that these deleterious processes can be avoided or suppressed in IBT1 and IBT2, because the two dyes possess bulky structural features that impede aggregation.70 For IBT1, the rod-shaped configuration is surrounded by a circle alkyl chain, so the dye–dye interaction on the surface of TiO2 is controlled. A peculiar cross-shaped rigid structure is found in IBT2, which reduces the tendency to form aggregations due to the inhibition of intermolecular interactions.71 These results are consistent with the conclusions of the anti-aggregation ability.
Table 2 Optimized structures and electron distributions in the HOMO and LUMO levels of the dyes IBT1–3
For the dyes IBT1 and IBT2, the HOMO orbitals are delocalized over the whole D–A–π portion, but mainly delocalized over the D–A portion for IBT3. The difference is caused by the fact that the two alkyl chains between the two thiophene units break the planarity of the skeleton. This result is confirmed by the following dihedral angle calculation. The LUMO orbitals of the three dyes are distributed along the A–π–A section. Besides, the HOMO and LUMO orbitals of IBT1–3 have obvious overlap on the additional acceptor BTD group, which can promote electron transfer from the indoline donor to the cyanoacetic acid acceptor.
The dihedral angles of each part were achieved through the optimized ground-state geometries of these sensitizers. The dihedral angles between the extra-acceptor and the π-spacer of IBT1, IBT2 and IBT3 are 0.9°, 1.5° and 1.5°, respectively. This result shows no obvious effect on the planarity of the D–A part when introducing different π-spacers into the skeleton of the three dyes. The dihedral angles between the bithiophene units are 0.6°, 0° and 49.8°, respectively. It can be found that IBT1 and IBT2 possess excellent planarity of the π-spacer, which is beneficial to charge transfer from the donor to the acceptor. On the contrary, dye IBT3 shows the biggest twist angle between the two thiophene units, leading to a blue-shifted maximum absorption wavelength and lower molar extinction coefficient. The twist angles between π-spacer and acceptor of IBT1, IBT2 and IBT3 are 0.2°, 0.1° and 3.0°, respectively. The superior planarity of the π–A part is beneficial to electron injection into the conduction band of TiO2.
3.5 Photovoltaic performance of the DSSCs
The cells based on IBT1–3 were fabricated with iodine electrolyte and the photovoltaic properties of the dyes were determined (Fig. 4). The relevant parameters are listed in Table 3. The photocurrent–voltage (J–V) measurements are exhibited in Fig. 4a. The devices based on IBT1, IBT2 and IBT3 show a PCE of 6.92%, 6.67% and 7.21%, respectively. The dye IBT3 achieves the highest efficiency, producing a short-circuit photocurrent (Jsc) of 15.17 mA cm−2, an open-circuit photovoltage (Voc) of 689 mV and a fill factor (FF) of 0.69. As listed in Table 3, the loading amounts of dyes IBT1, IBT2 and IBT3 are 1.09 × 10−7 mol cm−2, 1.66 × 10−7 mol cm−2 and 2.50 × 10−7 mol cm−2, respectively. Compared with IBT3, IBT1 and IBT2 show a relatively lower efficiency, which might be due to the lower loading amount on the surface of TiO2.69 The device based on IBT1 presents the highest Voc because EIMW is introduced as a π-spacer. EIMW has a rotaxane-like configuration, the circle chain plays a role as a compact layer on the surface of TiO2. The compact layer produces an efficient shielding effect to retard the I3− ion from approaching the TiO2 surface and reduces the charge recombination rate. Therefore, the DSSC based on IBT1 achieved the highest Voc.54,72 The reason that the alkyl chain can inhibit charge recombination is similar to that of EIMW,43,68 but the circle chain weakens charge recombination more effectively than the linear alkyl chain. Consequently, the Voc of the device based on IBT3 is lower than IBT1. However, for IBT2 with SPDF as π-spacer, a large steric hindrance is obtained, the gap between the two molecular frames is oversized, the charge recombination is easier, and so the Voc of the DSSC based on IBT2 drops significantly.
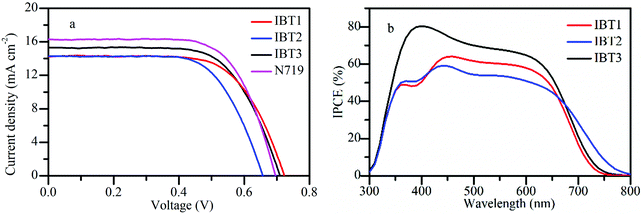 |
| Fig. 4
J–V curves (a) and IPCE spectra (b) of DSSCs based on IBT1–3. | |
Table 3 Photovoltaic parameters of the devices sensitized with IBT1–3
Dye |
J
sc (mA cm−2) |
V
oc (mV) |
FF (%) |
PCE (%) |
Dye loading amount (×10−7 mol cm−2) |
The photoanodes were immersed in a 0.3 mM solution of the three dyes in CHCl3/CH3CH2OH (v/v, 1/1) for 24 h.
CDCA was added into the dye solution at a concentration of 1 mM.
The photoanodes were first immersed in a 0.3 mM solution of IBT1 (or IBT2) in CHCl3/CH3CH2OH (v/v, 1/1) for 24 h, rinsed with ethanol, and then immersed into a 0.3 mM solution of IBT3 in CHCl3/CH3CH2OH (v/v, 1/1) for 3 h.
|
IBT1
|
14.30 |
722 |
0.67 |
6.92 |
1.09 |
IBT2
|
14.55 |
665 |
0.69 |
6.67 |
1.66 |
IBT3
|
15.17 |
689 |
0.69 |
7.21 |
2.50 |
IBT1 + CDCAb |
14.05 |
728 |
0.68 |
6.96 |
— |
IBT2 + CDCAb |
14.01 |
669 |
0.69 |
6.47 |
— |
IBT3 + CDCAb |
15.43 |
721 |
0.68 |
7.56 |
— |
IBT1 + IBT3c |
15.83 |
729 |
0.68 |
7.85 |
1.04 (IBT1) |
0.648 (IBT3) |
IBT2 + IBT3c |
16.21 |
693 |
0.68 |
7.64 |
1.49 (IBT2) |
0.948 (IBT3) |
N719
|
16.53 |
698 |
0.68 |
7.85 |
|
In order to investigate the relationship between the structure and performance, the theoretical efficiencies of the three dyes are calculated based on the same adsorption amount as the dye IBT2 (1.66 × 10−7 mol cm−2). The calculated efficiencies of the dyes IBT1, IBT2 and IBT3 are 10.50%, 6.67% and 4.79%, respectively, if the same adsorption amount is 1.66 × 10−7 mol cm−2. This result manifests that the anti-aggregation characteristic of the two featured π-spacers (EIMW and SPDF) is favorable for harvesting the sunlight and obtaining excellent photoelectric performance.
In order to explain the tendency of Jsc reasonably, the incident photo-to-current conversion efficiency (IPCE) spectra were measured and the results are shown in Fig. 4b. In the range of 300–670 nm, the plateaus of the IPCE spectrum become higher and higher in the order of IBT2 < IBT1 < IBT3, so these behaviors are consistent with the variation in Jsc. Meanwhile, the device based on dye IBT2 shows the widest IPCE response. This phenomenon is consistent with the previous UV-Vis absorption spectra (Fig. 2). For dye IBT2, a lower plateau results from the decreased loading amount, but it exhibits a wider light-harvesting region, along with no distinct valley over the whole visible wavelength. This phenomenon indicates that the SPDF as a π-bridge might have great potential in the photoelectronic field.
3.6 Anti-aggregation ability
Dye aggregation is a negative factor in DSSCs. Unfortunately, it is a common phenomenon, especially for planar dyes. We have reported an organic dye PH2 (Fig. S17, ESI†) with EIMW as an auxiliary donor to suppress dye aggregation in DSSCs considering that EIMW is a big ring with large steric hindrance. The results show that EIMW is a good auxiliary donor to suppress dye aggregation. In this study, we focused on the effect of structural engineering of the π-spacer with EIMW and DHBT to suppress dye aggregation in DSSCs. The dye IBT3 with 3,3′-dihexyl-2,2′-bithiophene as π-bridge was chosen as the reference dye because two alkyl chains in the π-bridge show a good anti-aggregation effect.
It is well known that chenodexycholic acid (CDCA) is usually used as a co-adsorbent to inhibit dye aggregation. But for an anti-aggregation dye, the Jsc would decrease because of competitive adsorption, which would not improve the photoelectric performance after addition of CDCA.73 In this work, an appropriate amount (1 mM) of CDCA was added to verify whether the two π-bridges can suppress dye aggregation. The J–V characteristics of the DSSCs are exhibited in Fig. 5 and relevant photovoltaic data are listed in Table 3. When CDCA was added, the efficiency of the device based on IBT3 was improved from 7.21% to 7.53%, which means that there is still aggregation even though the two alkyl chains in the π-bridge act as good anti-aggregation groups. However, the Jsc values of the two devices based on IBT1 and IBT2 show a slight decrease because of competitive adsorption. The efficiency shows only a minor variation for IBT1, which demonstrates that EIMW exhibits better anti-aggregation ability than alkyl chains. The higher anti-aggregation of EIMW might be rationalized by its larger steric hindrance. More interestingly, the PCE of the cell based on IBT2 slightly declined, which demonstrates that SPDF exhibits the strongest anti-aggregation ability due to the rigid ‘T’ configuration among these dyes. SPDF has a peculiar rigid ‘T’ structure with two perpendicularly oriented molecular halves. The 3-dimensional structure can inhibit intermolecular interactions efficaciously. However, EIMW and the linear alkyl chain can also inhibit dye aggregation, but these are stretchable architectures; when the dyes are adsorbed on the surface of TiO2, the alkyl chain of the two π-spacers can move freely within a certain range, so the π–π stacking is eliminated incompletely. Therefore, the flexible structure leads to a weaker anti-aggregation ability than the rigid structure. In comparison with the dye PH1 with EIMW as an auxiliary donor, IBT1 shows more effective anti-aggregation ability when EIMW is introduced as a π-bridge, which suggests that the anti-aggregation effect of the EIMW unit as a π-bridge is more efficacious than as an auxiliary donor.
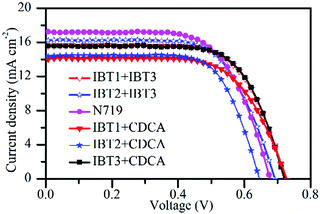 |
| Fig. 5
J–V characteristics of DSSCs based on six devices. | |
3.7 Co-sensitization
In order to reduce the deficiencies that result from the low loading amount and the limit of the light-harvesting region, co-sensitization tactics were applied to improve the photovoltaic performance. As mentioned above, IBT1 and IBT2 show an obvious absorption valley in the range of 300–500 nm, but on the contrary, IBT3 shows a notable absorption peak in this region (Fig. S18 and S19, ESI†). The absorption spectra of the photoanodes co-sensitized by IBT3 with IBT1 and IBT2 are shown in Fig. S20 (ESI†). Compared with the photoanodes without co-sensitization, the absorption spectra of the two new photoanodes show significant differences in the range of 400–500 nm, and the valleys of IBT1 and IBT2 are filled up by an absorption peak that appears for IBT3 in the same region. Thus, we utilize IBT3 as a co-sensitizer to make up the weak absorption between 300 and 500 nm of IBT1 and IBT2. The corresponding photovoltaic parameters are listed in Table 3 and Fig. 5. For the devices co-sensitized by IBT3 with IBT1 and IBT2, the PCEs increase to 7.85% and 7.64%, respectively, which reach that of the reference dye N719 (7.85%) under the same conditions. After co-sensitization, the loading amount of each dye was measured (Table 3). The results manifest that using a co-sensitizer can minimize the loss of low loading amount resulting from a bulky anti-aggregation π-bridge and improve the available photovoltaic performance.
3.8 Electrochemical impedance spectroscopy
The charge recombination rate of a device has an important influence on Voc. At the same photocarrier generation flux, the charge recombination is primarily determined by the interfacial electron recombination rate based on the surface of TiO2. To study the charge recombination dynamics, electrochemical impedance spectra (EIS) of DSSCs were obtained under 0.68 V bias voltage in the dark. The results are shown in Fig. 6 and the relevant parameters are listed in Table 4. The small semicircle represents the charge transfer resistance (Rce) at the interface of the counter electrode and electrolyte. The second semicircle represents the charge transfer resistance (Rrec) at the interface of TiO2, dye and electrolyte. The Rce values of the three dyes are similar, which may be due to the same batch of counter electrode and electrolyte. However, the radius of the large semicircle increased in the order: IBT2 < IBT3 < IBT1, which is in agreement with the variation of Voc. The results also indicate that the circle alkyl chain can weaken charge recombination more effectively than the linear alkyl chain because a tight compact layer is formed on the surface of TiO2. A large steric hindrance is obtained by SPDF, but charge recombination is easier because the gap between the two molecular frames is oversized. This observation can also be verified in the Bode phase plots (Fig. 6b). The peaks with the frequency lying in the range of 10−1–102 Hz represent the electron transfer frequency at the interface of TiO2/dye/electrolyte. Moreover, the electron lifetime (τ) can be calculated by τct = Rrec × CCPE.74,75 The corresponding electron lifetime values of DSSCs based on IBT1, IBT2 and IBT3 are 61.5 ms, 21.8 ms and 50.7 ms, respectively. The shorter electron lifetime stands for a higher dark current.
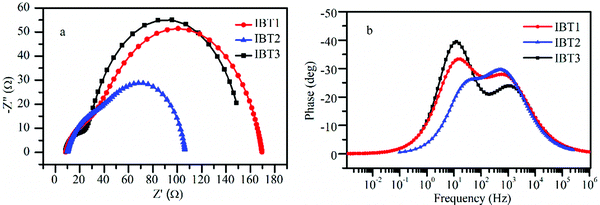 |
| Fig. 6 Nyquist (a) and Bode phase plots (b) of electrochemical impedance spectra (EIS) for DSSCs based on IBT1–3. | |
Table 4 Parameters of the electrochemical impedance spectra for DSSCs
Dye |
R
ce (Ω cm−2) |
R
rec (Ω cm−2) |
CPE2 (μF) |
τ (ms) |
IBT1
|
27.34 |
133.7 |
460 |
61.5 |
IBT2
|
33.67 |
63.1 |
346 |
21.8 |
IBT3
|
19.21 |
128.1 |
396 |
50.7 |
The electrochemical impedance spectra (EIS) were also recorded under different applied voltages in the dark.76,77 The results are shown in Fig. S21 (ESI†). At a fixed potential, the Rrec values increased in the order of IBT2 < IBT3 < IBT1, consistent with the variation of Voc. This result indicates that the introduction of EIMW is beneficial to the suppression of charge recombination. After co-sensitization, the two new devices present higher Rrec values than the DSSCs without co-sensitization, respectively (Fig. S22, ESI†). This result is consistent with the variation of the Voc.
4. Conclusion
Two novel D–A–π–A metal-free organic dyes IBT1 and IBT2 with EIMW and SPDF as anti-aggregation π-bridges were designed, synthesized and applied in DSSCs. Due to the excellent photoelectric performance of EIMW and SPDF, IBT1 achieves a higher Voc and IBT2 shows a wider IPCE response, respectively. In addition, the co-adsorption experiment with CDCA demonstrates that EIMW and SPDF as π-bridges inhibit dye aggregation more significantly than DHBT. After dyes IBT1 and IBT2 are co-sensitized with IBT3, the new devices exhibit the same high efficiencies as that of N719. This work not only provides an anti-aggregation strategy by structural engineering of the π-spacer, but also two novel dyes as promising candidates for efficient DSSCs.
Conflicts of interest
The authors declare no competing financial interest.
Acknowledgements
We are grateful to the National Natural Science Foundation of China (21572069, 21772045), the National Key Research and Development Program of China (2016YFA0602900), and the Natural Science Foundation of Guangdong Province, China (2018B030311008).
References
- A. Hagfeldt, G. Boschloo, L. Sun, L. Kloo and H. Pettersson, Chem. Rev., 2010, 110, 6595–6663 CrossRef CAS PubMed
.
- L. L. Li and E. W. Diau, Chem. Soc. Rev., 2013, 42, 291–304 RSC
.
- J. Gong, K. Sumathy, Q. Qiao and Z. Zhou, Renewable Sustainable Energy Rev., 2017, 68, 234–246 CrossRef CAS
.
- H. Meier, Z.-S. Huang and D. Cao, J. Mater. Chem. C, 2017, 5, 9828–9837 RSC
.
- A. Mishra, M. K. R. Fischer and P. Bäuerle, Angew. Chem., Int. Ed., 2009, 48, 2474–2499 CrossRef CAS PubMed
.
- M. Freitag, J. Teuscher, Y. Saygili, X. Zhang, F. Giordano, P. Liska, J. Hua, S. M. Zakeeruddin, J.-E. Moser, M. Grätzel and A. Hagfeldt, Nat. Photonics, 2017, 11, 372–378 CrossRef CAS
.
- X. Li, X. Zhang, J. Hua and H. Tian, Mol. Syst. Des. Eng., 2017, 2, 98–122 RSC
.
- S. Chaurasia, C.-J. Liang, Y.-S. Yen and J. T. Lin, J. Mater. Chem. C, 2015, 3, 9765–9780 RSC
.
- B. O'Regan and M. Grätzel, Nature, 1991, 353, 737 CrossRef
.
- C. Li, L. Luo, D. Wu, R. Jiang, J. Lan, R. Wang, L. Huang, S. Yang and J. You, J. Mater. Chem. A, 2016, 4, 11829–11834 RSC
.
- Y. Huang, W.-C. Chen, R. Ghadari, X.-P. Liu, X.-Q. Fang, T. Yu and F.-T. Kong, J. Power Sources, 2018, 396, 559–565 CrossRef CAS
.
- G. Saritha, R. V. Mangalaraja and S. Anandan, Sol. Energy, 2017, 146, 150–160 CrossRef CAS
.
- J. S. Ni, W. S. Kao, H. J. Chou and J. T. Lin, ChemSusChem, 2015, 8, 2932–2939 CrossRef CAS PubMed
.
- Z.-S. Huang, H. Meier and D. Cao, J. Mater. Chem. C, 2016, 4, 2404–2426 RSC
.
- J. H. Yum, E. Baranoff, F. Kessler, T. Moehl, S. Ahmad, T. Bessho, A. Marchioro, E. Ghadiri, J. E. Moser, C. Yi, M. K. Nazeeruddin and M. Gratzel, Nat. Commun., 2012, 3, 631 CrossRef PubMed
.
- G. Wu, F. Kong, Y. Zhang, X. Zhang, J. Li, W. Chen, W. Liu, Y. Ding, C. Zhang, B. Zhang, J. Yao and S. Dai, J. Phys. Chem. C, 2014, 118, 8756–8765 CrossRef CAS
.
- P. Dai, L. Yang, M. Liang, H. Dong, P. Wang, C. Zhang, Z. Sun and S. Xue, ACS Appl. Mater. Interfaces, 2015, 7, 22436–22447 CrossRef CAS PubMed
.
- L. Zhang, X. Yang, W. Wang, G. G. Gurzadyan, J. Li, X. Li, J. An, Z. Yu, H. Wang, B. Cai, A. Hagfeldt and L. Sun, ACS Energy Lett., 2019, 4, 943–951 CrossRef CAS
.
- X. Xie, D. Sun, Y. Wei, Y. Yuan, J. Zhang, Y. Ren and P. Wang, J. Mater. Chem. A, 2019, 7, 11338–11346 RSC
.
- K. Kakiage, Y. Aoyama, T. Yano, K. Oya, J. Fujisawa and M. Hanaya, Chem. Commun., 2015, 51, 15894–15897 RSC
.
- Z. Ning and H. Tian, Chem. Commun., 2009, 5483–5495 RSC
.
- X. Ren, S. Jiang, M. Cha, G. Zhou and Z.-S. Wang, Chem. Mater., 2012, 24, 3493–3499 CrossRef CAS
.
- C.-Y. Lo, D. Kumar, S.-H. Chou, C.-H. Chen, C.-H. Tsai, S.-H. Liu, P.-T. Chou and K.-T. Wong, ACS Appl. Mater. Interfaces, 2016, 8, 27832–27842 CrossRef CAS PubMed
.
- H.-H. Gao, X. Qian, W.-Y. Chang, S.-S. Wang, Y.-Z. Zhu and J.-Y. Zheng, J. Power Sources, 2016, 307, 866–874 CrossRef CAS
.
- G. S. Selopal, H.-P. Wu, J. Lu, Y.-C. Chang, M. Wang, A. Vomiero, I. Concina and E. W.-G. Diau, Sci. Rep., 2016, 6, 18756 CrossRef CAS PubMed
.
- D. Joly, M. Godfroy, L. Pellejà, Y. Kervella, P. Maldivi, S. Narbey, F. Oswald, E. Palomares and R. Demadrille, J. Mater. Chem. A, 2017, 5, 6122–6130 RSC
.
- Y. K. Eom, S. H. Kang, I. T. Choi, Y. Yoo, J. Kim and H. K. Kim, J. Mater. Chem. A, 2017, 5, 2297–2308 RSC
.
- F. Lu, G. Yang, Q. Xu, J. Zhang, B. Zhang and Y. Feng, Dyes Pigm., 2018, 158, 195–203 CrossRef CAS
.
- J. S. Ni, Y. C. Yen and J. T. Lin, Chem. Commun., 2015, 51, 17080–17083 RSC
.
- L.-W. Ma, Z.-S. Huang, S. Wang, H. Meier and D. Cao, Dyes Pigm., 2017, 145, 126–135 CrossRef CAS
.
- X. Lu, Q. Feng, T. Lan, G. Zhou and Z.-S. Wang, Chem. Mater., 2012, 24, 3179–3187 CrossRef CAS
.
- J. Yang, P. Ganesan, J. Teuscher, T. Moehl, Y. J. Kim, C. Yi, P. Comte, K. Pei, T. W. Holcombe, M. K. Nazeeruddin, J. Hua, S. M. Zakeeruddin, H. Tian and M. Grätzel, J. Am. Chem. Soc., 2014, 136, 5722–5730 CrossRef CAS PubMed
.
- G. Wang, Z. Liu, Y. Deng, L. Xie and S. Tan, Dyes Pigm., 2017, 145, 427–435 CrossRef CAS
.
- Y. Hao, Y. Saygili, J. Cong, A. Eriksson, W. Yang, J. Zhang, E. Polanski, K. Nonomura, S. M. Zakeeruddin, M. Grätzel, A. Hagfeldt and G. Boschloo, ACS Appl. Mater. Interfaces, 2016, 8, 32797–32804 CrossRef CAS PubMed
.
- C. Aumaitre, C. Rodriguez-Seco, J. Jover, O. Bardagot, F. Caffy, Y. Kervella, N. López, E. Palomares and R. Demadrille, J. Mater. Chem. A, 2018, 6, 10074–10084 RSC
.
- M. Mao, X.-L. Zhang, X.-Q. Fang, G.-H. Wu, Y. Ding, X.-L. Liu, S.-Y. Dai and Q.-H. Song, Org. Electron., 2014, 15, 2079–2090 CrossRef CAS
.
- J.-B. Wang, X.-Q. Fang, X. Pan, S.-Y. Dai and Q.-H. Song, Chem. – Asian J., 2012, 7, 696–700 CrossRef CAS PubMed
.
- S. Nagane, A. Ichake, I. Agrawal, A. Sadhanala, R. H. Friend, S. Ogale and P. P. Wadgaonkar, ChemPlusChem, 2017, 82, 280–286 CrossRef CAS
.
- S. Qu, C. Qin, A. Islam, Y. Wu, W. Zhu, J. Hua, H. Tian and L. Han, Chem. Commun., 2012, 48, 6972 RSC
.
- Y. Wu, X. Zhang, W. Li, Z.-S. Wang, H. Tian and W. Zhu, Adv. Energy Mater., 2012, 2, 149–156 CrossRef CAS
.
- Z. Chai, M. Wu, M. Fang, S. Wan, T. Xu, R. Tang, Y. Xie, A. Mei, H. Han, Q. Li and Z. Li, Adv. Energy Mater., 2015, 5, 1500846 CrossRef
.
- J. Luo, X. Wang, L. Fan, G. Li, Q. Qi, K.-W. Huang, T. L. Dexter Tam, J. Zhang, Q. Wang and J. Wu, J. Mater. Chem. C, 2016, 4, 3709–3714 RSC
.
- Q. Chai, W. Li, Y. Wu, K. Pei, J. Liu, Z. Geng, H. Tian and W. Zhu, ACS Appl. Mater. Interfaces, 2014, 6, 14621–14630 CrossRef CAS PubMed
.
- K. Chaitanya, B. M. Heron and X.-H. Ju, Dyes Pigm., 2017, 141, 501–511 CrossRef CAS
.
- W. Zhang, Y. Wu, X. Li, E. Li, X. Song, H. Jiang, C. Shen, H. Zhang, H. Tian and W.-H. Zhu, Chem. Sci., 2017, 8, 2115–2124 RSC
.
- X. Li, B. Xu, P. Liu, Y. Hu, L. Kloo, J. Hua, L. Sun and H. Tian, J. Mater. Chem. A, 2017, 5, 3157–3166 RSC
.
- X. Li, Y. Hu, I. Sanchez-Molina, Y. Zhou, F. Yu, S. A. Haque, W. Wu, J. Hua, H. Tian and N. Robertson, J. Mater. Chem. A, 2015, 3, 21733–21743 RSC
.
- X. Wang, J. Yang, H. Yu, F. Li, L. Fan, W. Sun, Y. Liu, Z. Y. Koh, J. Pan, W.-L. Yim, L. Yan and Q. Wang, Chem. Commun., 2014, 50, 3965–3968 RSC
.
- M. Xu, X. Hu, Y. Zhang, X. Bao, A. Pang and J.-K. Fang, ACS Appl. Energy Mater., 2018, 1, 2200–2207 CrossRef CAS
.
- R. Milan, G. S. Selopal, M. Cavazzini, S. Orlandi, R. Boaretto, S. Caramori, I. Concina and G. Pozzi, Sci. Rep., 2017, 7, 15675 CrossRef PubMed
.
- E. Colom, J. M. Andrés-Castán, D. Barrios, I. Duerto, S. Franco, J. Garín, J. Orduna, B. Villacampa and M. J. Blesa, Dyes Pigm., 2019, 164, 43–53 CrossRef CAS
.
- J. Liu, Y. Numata, C. Qin, A. Islam, X. Yang and L. Han, Chem. Commun., 2013, 49, 7587 RSC
.
- K. Zeng, Y. Lu, W. Tang, S. Zhao, Q. Liu, W. Zhu, H. Tian and Y. Xie, Chem. Sci., 2019, 10, 2186–2192 RSC
.
- T. Hua, Z.-S. Huang, K. Cai, L. Wang, H. Tang, H. Meier and D. Cao, Electrochim. Acta, 2019, 302, 225–233 CrossRef CAS
.
- Y. Tang, Y. Wang, X. Li, H. Agren, W. H. Zhu and Y. Xie, ACS Appl. Mater. Interfaces, 2015, 7, 27976–27985 CrossRef CAS PubMed
.
- R. Bisht, S. Singh, K. Krishnamoorthy and J. Nithyanandhan, J. Org. Chem., 2017, 82, 1920–1930 CrossRef CAS PubMed
.
- H. Dong, M. Liang, C. Zhang, Y. Wu, Z. Sun and S. Xue, J. Phys. Chem. C, 2016, 120, 22822–22830 CrossRef CAS
.
- W. Zhang, Y. Wu, H. Zhu, Q. Chai, J. Liu, H. Li, X. Song and W.-H. Zhu, ACS Appl. Mater. Interfaces, 2015, 7, 26802–26810 CrossRef CAS PubMed
.
- C. Chen, J.-Y. Liao, Z. Chi, B. Xu, X. Zhang, D.-B. Kuang, Y. Zhang, S. Liu and J. Xu, J. Mater. Chem., 2012, 22, 8994 RSC
.
- Z.-S. Huang, H.-L. Feng, X.-F. Zang, Z. Iqbal, H. Zeng, D.-B. Kuang, L. Wang, H. Meier and D. Cao, J. Mater. Chem. A, 2014, 2, 15365–15376 RSC
.
- Z. Iqbal, W.-Q. Wu, H. Zhang, L. Han, X. Fang, L. Wang, D.-B. Kuang, H. Meier and D. Cao, Org. Electron., 2013, 14, 2662–2672 CrossRef CAS
.
- K. Sugiyasu, Y. Honsho, R. M. Harrison, A. Sato, T. Yasuda, S. Seki and M. Takeuchi, J. Am. Chem. Soc., 2010, 132, 14754–14756 CrossRef CAS PubMed
.
- H. Naoto, N. Takashi, M. Takuya, N. Hiroki, M. Keiko, M. Mikio and H. Hiroyuki, Bull. Chem. Soc. Jpn., 2007, 80, 371–386 CrossRef
.
- C. Zhao, T. Sakurai, S. Yoneda, S. Seki, M. Sugimoto, C. Oki, M. Takeuchi and K. Sugiyasu, Chem. – Asian J., 2016, 11, 2284–2290 CrossRef CAS PubMed
.
- H. Hanamura and N. Nemoto, Polymer, 2014, 55, 6672–6679 CrossRef CAS
.
- Y. Wu, M. Marszalek, S. M. Zakeeruddin, Q. Zhang, H. Tian, M. Grätzel and W. Zhu, Energy Environ. Sci., 2012, 5, 8261 RSC
.
- S. Cai, X. Hu, Z. Zhang, J. Su, X. Li, A. Islam, L. Han and H. Tian, J. Mater. Chem. A, 2013, 1, 4763 RSC
.
- J. He, W. Wu, J. Hua, Y. Jiang, S. Qu, J. Li, Y. Long and H. Tian, J. Mater. Chem., 2011, 21, 6054 RSC
.
- H.-L. Jia, Y.-C. Chen, L. Ji, L.-X. Lin, M.-Y. Guan and Y. Yang, Dyes Pigm., 2019, 163, 589–593 CrossRef CAS
.
- D. Heredia, J. Natera, M. Gervaldo, L. Otero, F. Fungo, C. Y. Lin and K. T. Wong, Org. Lett., 2010, 12, 12–15 CrossRef CAS PubMed
.
- G. Pozzi, S. Orlandi, M. Cavazzini, D. Minudri, L. Macor, L. Otero and F. Fungo, Org. Lett., 2013, 15, 4642–4645 CrossRef CAS PubMed
.
- C. H. Huang and Y. J. Chang, Tetrahedron Lett., 2014, 55, 4938–4942 CrossRef CAS
.
- H. Chen, G. Lyu, Y. Yue, T. Wang, D.-P. Li, H. Shi, J. Xing, J. Shao, R. Zhang and J. Liu, J. Mater. Chem. C, 2019, 7, 7249–7258 RSC
.
- Z. Shen, J. Chen, X. Li, X. Li, Y. Zhou, Y. Yu, H. Ding, J. Li, L. Zhu and J. Hua, ACS Sustainable Chem. Eng., 2016, 4, 3518–3525 CrossRef CAS
.
- J.-H. Park, D. G. Nam, B.-M. Kim, M. Y. Jin, D.-H. Roh, H. S. Jung, D. H. Ryu and T.-H. Kwon, ACS Energy Lett., 2017, 2, 1810–1817 CrossRef CAS
.
- K. T. Dembele, G. S. Selopal, C. Soldano, R. Nechache, J. C. Rimada, I. Concina, G. Sberveglieri, F. Rosei and A. Vomiero, J. Phys. Chem. C, 2013, 117, 14510–14517 CrossRef CAS
.
- H. Li, M. Fang, R. Tang, Y. Hou, Q. Liao, A. Mei, H. Han, Q. Li and Z. Li, J. Mater. Chem. A, 2016, 4, 16403–16409 RSC
.
Footnote |
† Electronic supplementary information (ESI) available: Complete experimental details, 1H NMR and 13C NMR spectra of the new compounds, spectroscopic data. See DOI: 10.1039/c9tc03066b |
|
This journal is © The Royal Society of Chemistry 2019 |