The preparation of oxidized methylcellulose crosslinked by adipic acid dihydrazide loaded with vitamin C for traumatic brain injury†
Received
26th April 2019
, Accepted 21st June 2019
First published on 12th July 2019
Abstract
Traumatic brain injury (TBI) is defined as the destruction or degeneration of brain cells due to external force. Free radical generation from the damage site is the major factor that causes post-therapeutic failure in TBI patient. In this study, an oxi-methylcellulose-adipic acid dihydrazide (oxi-MC-ADH) hydrogel was prepared as a vitamin C carrier for TBI in emergency that continuously releases vitamin C at the injured site in the brain. The results show that the oxi-MC-ADH hydrogel exhibits adequate gelation time and good biocompatibility in vitro. Vitamin C incorporated in the oxi-MC-ADH hydrogel (oxi-MC-ADH-VC) initially burst released in the first 6 h to serve as a primary treatment and then extendedly released for the secondary treatment. The analysis of gene expression and reactive oxygen species production was done to demonstrate the neuroprotective effects of the hydrogel. In vivo neurological function assays and behavioral tests show a significant improvement in the outcome in rats with TBI 3 weeks after the oxi-MC-ADH-VC hydrogel injection. This study demonstrates that the oxi-MC-ADH-VC hydrogel has a great potential in the management of TBI.
Introduction
Traumatic brain injury (TBI) is defined as brain damage caused by an external mechanical force.1 It is one of the major factors that cause disability and mortality.2 Commonly, traffic accidents, excessive drinking, falls, sports injuries, and criminal violence are the major causes of TBI.
The consequences of TBI and the following medical care account for US$400 billion in medical expenses annually worldwide. Considering an estimated gross world product of about US$80 trillion,3 approximately US$1 for every US$200 is spent on TBI-related consequences.4 TBI affects a large number of people globally5 and leads to an inconvenient lifestyle and imposes a large economic burden.
TBI can be divided into three stages: mild, moderate, and severe.6 If a patient is not treated adequately and immediately after the injury, a mild TBI may progress to a moderate or even severe stage, leading to adverse sequelae such as impaired cognition, behavior, and communication.
Once TBI has happened, a significant increase in the reactive oxygen species (ROS) produced at the injury site can leads to neuronal and vasculature damage that causes secondary brain injury. The subsequent results may cause major deterioration symptoms such as hypoxia, edema, hypotension, or hematomas.7 Moreover, the high level of ROS could result in severe cerebral ischemia, edema, intracranial hypertension, and cerebral hypoxia.8,9
Adequate treatment for TBI patients in the first few hours after the injury, such as to stabilize the patient by using drugs offering neuroprotection and thereby minimizing the secondary damages caused by ROS, are of utmost importance to prevent neurological deterioration later on.10 One approach is to inject phosphate buffered saline (PBS) containing a high concentration of vitamin C into the peritoneal cavity to mitigate the oxidative stress at the damaged site. However, the results of the approach are not so promising because the injected vitamin C loses bioactivity after a long circulation period. Therefore, the local delivery of an antioxidant to the damaged site is more reasonable than injecting it into a distant site. The aim of this study is to prepare a methyl-cellulose hydrogel as a carrier for vitamin C to serve as a real-time local delivery formulation for the diffusion of ROS from the injury site so as to mitigate the secondary damage following TBI.
Methylcellulose (MC) is a water-soluble polysaccharide derived from cellulose by the partial substitution of the hydroxyl groups with the methoxy groups.11 MC has been widely used in many medical applications such as drug delivery, cosmetic surgery, and bio-ink due to its good biocompatibility and adequate supportive strength.12–14 Vitamin C is a powerful antioxidant and one of the important co-enzymes in the human body. It has been reported to have neuroprotective effects against ROS-related ailments in animals such as Alzheimer's disease, seizures, stroke, and brain injury.15–18
In this study, the oxi-MC hydrogel was prepared as a carrier of vitamin C and directly applied to the damage area once TBI happened. The vitamin C could be locally delivered to the damaged site as a ROS scavenger to diffuse the ROS from the injured area immediately. MC was oxidized by sodium meta-periodate (NaIO4) and cross-linked with adipic acid dihydrazide (ADH) (oxi-MC-ADH). Vitamin C was mixed within the hydrogel (oxi-MC-ADH-VC). In this work, a trinitrobenzene sulfonate (TNBS) assay was used to detect the oxidation degree of MC and the functional groups of oxi-MC were characterized via Fourier transform infrared spectrometry (FT-IR) and nuclear magnetic resonance (NMR) spectroscopy. Elastic (G′) and viscous (G′′) modules of the hydrogel were measured by a rheometer to determine the gelation time. The release profile of vitamin C from oxi-MC-ADH was studied by UV-vis spectrophotometry. The in vitro biocompatibility was evaluated by water-soluble tetrazolium salts (WST-1), lactate dehydrogenase (LDH), and Live/Dead staining. The related gene expression was detected by the quantitative polymerase chain reaction (qPCR). Finally, a rat TBI model was used to prove the concept and to evaluate the safety of the developed hydrogel in vivo. We believe that the oxi-MC-ADH-VC hydrogel does not only have the property of burst release of vitamin C to diffuse the ROS in the first few hours after TBI happened but can also sustain the release for almost 1 week to scavenge the ROS from the TBI area to avoid consecutive neuronal degeneration.
Results
Characterization of the oxi-MC preparation
The recovery yield of the oxi-MC preparation was determined by the weight of freeze-dried oxi-MC and the value obtained was 91.53 ± 1.38%. The absorption bond in the FT-IR spectra at 1730 cm−1 was due to the stretching of the C
O bonds in oxi-MC (Fig. 1A). The determined chemical shift based on the NMR spectrum in the aldehyde spectral region of oxi-MC was in the range of 8–10 ppm (Fig. 1B). The oxidation degree of oxi-MC was 21 ± 4.49%, as determined by the TNBS assay for aldehyde content.
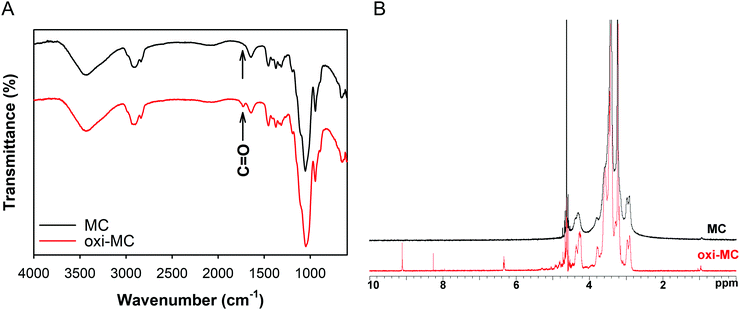 |
| Fig. 1 Characterization of MC and oxi-MC. (A) The FT-IR spectrum of oxi-MC; the arrow indicates the vibrational peak for the aldehyde functional groups of oxi-MC at 1730 cm−1, and (B) the 1H NMR spectrum of oxi-MC; the aldehyde spectral region was in the range of 8–10 ppm. | |
Viscoelastic properties of the oxi-MC preparation
The average gelation time of oxi-MC-ADH and oxi-MC-ADH-VC at 37 °C was 371.34 ± 35.56 s and 54.07 ± 5.10 s, respectively (Table 1 and Fig. S1, ESI†). This indicated that oxi-MC-ADH-VC has a rapid gelation time of <5 min at normal human body temperature and can be used in the form of a local injection.
Table 1 Gelation time determination of the specimen at 37 °C by a rheometer
|
Gelation time (s) |
G′ = G′′ (Pa) |
Oxi-MC |
N.D. |
N.D. |
Oxi-MC-ADH |
371.34 ± 35.56 |
60.78 ± 33.72 |
Oxi-MC-ADH-VC |
54.07 ± 5.10 |
9.39 ± 2.09 |
Degradation of the oxi-MC-ADH-VC hydrogel
The degradation of the oxi-MC-ADH-VC hydrogel was evaluated from 1 to 14 days. The degradation percentage of the oxi-MC-ADH hydrogel at 1, 4, 7, 10, and 14 days was 22.86 ± 1.72%, 39.50 ± 1.02%, 43.25 ± 4.89%, 45.40 ± 7.01%, and 48.87 ± 4.75%, respectively (Fig. 2). In Fig. S2 (ESI†), the data indicates that there was no significant difference between the degradation percentages of oxi-MC-ADH and oxi-MC-ADH-VC. Consequently, oxi-MC-ADH-VC hydrogel can be considered as a degradable and sustained-release drug delivery system.
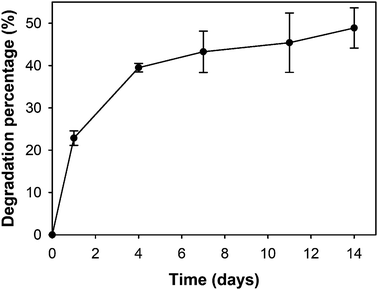 |
| Fig. 2 Degradation percentage of the oxi-MC-ADH-VC hydrogel. | |
Release profile of vitamin C from the oxi-MC-ADH-VC system
The release profile of vitamin C from the oxi-MC-ADH-VC hydrogel system is illustrated in Fig. 3. The percentage of cumulative release at 1, 3, 6, 12, 24, 48, 72, 96, 120, and 144 h was 53.68 ± 6.27%, 77.06 ± 2.79%, 82.13 ± 2.19%, 84.03 ± 2.09%, 87.73 ± 1.39%, 92.83 ± 1.29%, 96.01 ± 0.87%, 97.95 ± 0.39%, 99.16 ± 0.22%, and 100 ± 0.01%, respectively. A rapid burst-release (80%) was detected in the first 6 h, followed by a sustained release (total of 100%) up to 144 h. Hence, the oxi-MC-ADH-VC hydrogel can serve as an emergency ROS scavenger in acute TBI and can prevent subsequent secondary damage.
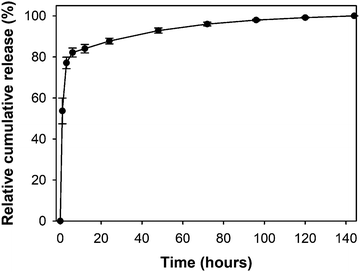 |
| Fig. 3 Release profile of the oxi-MC-ADH-VC hydrogel. | |
Evaluation of biocompatibility
Following the ISO 10993 standards, the biocompatibility of the control, oxi-MC-ADH, oxi-MC-ADH-VC, and total lysis was found to be 100 ± 5.00%, 101.38 ± 6.74%, 104.37 ± 6.49%, and 0.72 ± 0.51%, respectively (Fig. 4A). The OD values of LDH activity of the control group, oxi-MC/ADH hydrogel, oxi-MC-ADH-VitC, and total lysis were 0.83 ± 0.08, 0.76 ± 0.17, 0.61 ± 0.06, and 2.32 ± 0.10, respectively (Fig. 4B). The Live/Dead staining images represented the same trend (Fig. 4C). These results indicated that the oxi-MC-ADH-VC hydrogel system has excellent biocompatibility as an antioxidant carrier.
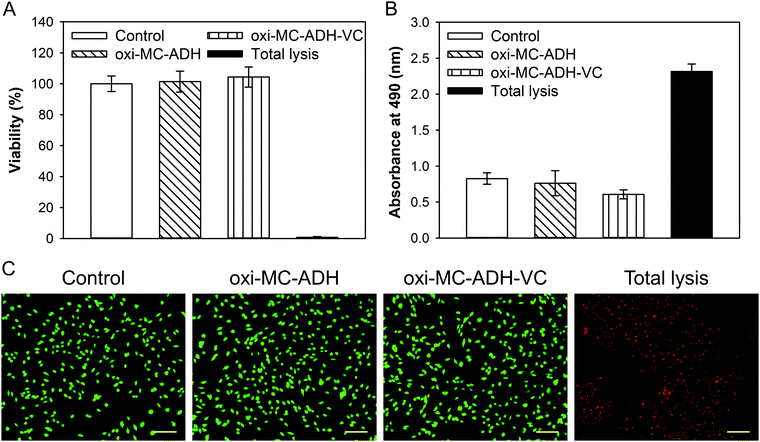 |
| Fig. 4 Evaluation of in vitro biocompatibility. (A) WST-1 assay, (B) LDH assay, and (C) Live/Dead staining of B35 cells (scale bar: 100 μm). | |
Determination of ROS
Intracellular ROS were induced by H2O2 and determined by the CM-H2DCFDA dye (Fig. 5). ROS-induced fluorescence levels in B35 cells exposed to H2O2 and H2O2 + oxi-MC-ADH were observed. The H2O2-treated B35 cells substantially recovered after treatment with oxi-MC-ADH-VC, confirming the neuroprotective effects of MC-ADH-VC.
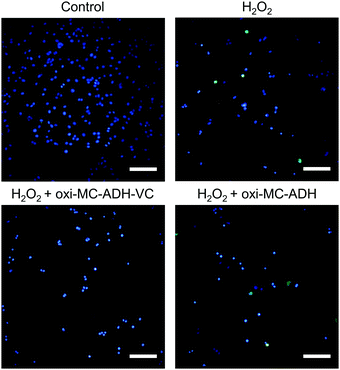 |
| Fig. 5 CM-H2DCFDA staining for ROS detection (scale bar: 100 μm). | |
Gene expression
The gene expression profiles of the oxidative stress, inflammatory response, and apoptosis-related genes were all significantly up-regulated in the H2O2-treated group (Fig. 6). For the oxidative stress-related gene evaluation, the iNOS (inducible nitric oxide synthase) signal was significantly down-regulated in the oxi-MC-ADH-VC group (p < 0.05). No significant difference was, however, noted in the nNOS (neural nitric oxide synthase) and eNOS (endothelial nitric oxide synthase) signals (Fig. 6A). In the inflammatory response-related genes, the mRNA levels of COX-2 (cyclooxygenase-2), IL-1β (interleukin-1 beta), and TNF-α (tumor necrosis factor-alpha) were all significantly decreased in the oxi-MC-ADH-VC group (Fig. 6B). All the apoptotic genes triggered by H2O2 were substantially repressed in the oxi-MC-ADH-VC group (Fig. 6C).
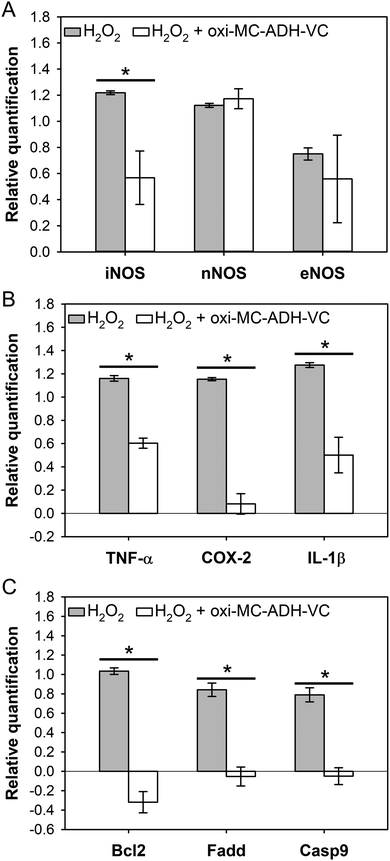 |
| Fig. 6 mRNA expression of the B35 cells. (A) ROS-related gene, (B) inflammation-related gene, and (C) apoptosis-related gene (*p < 0.05 compare with H2O2). | |
In vivo study
A cortical injury was induced in the right cerebral hemispheres of the experimental rats, which resulted in left-sided functional deficits. The functional deficits were evaluated by a mNSS test and scored as moderate (score 7–12) for three post-operative days. The mNSS score in the oxi-MC-ADH-VC group was, however, significantly lower than that in the untreated group after 3 and 4 weeks (p < 0.05) (Fig. 7A).
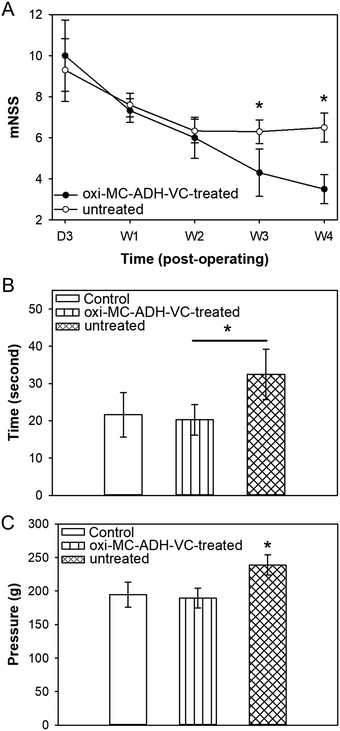 |
| Fig. 7 Evaluation of functional recovery of the oxi-MC-ADH-VC hydrogel for TBI rats. (A) mNSS, (B) hot plate test, and (C) paw pressure test (*p < 0.05). | |
Paw licking is a rapid response to a painful thermal stimulus and is a direct indicator of the nociceptive threshold. In the hot-plate heat test, the latency withdrawal time in the control group was 21.60 ± 5.99 s, while in the untreated group it increased to 32.47 ± 6.78 s because of the functional neurological deficits. In contrast, the licking latency time in the oxi-MC-ADH-VC-treated group significantly decreased to 20.26 ± 4.10 s (Fig. 7B) without a significant difference in comparison to the control group. In addition, the paw pressure test was used to quantify the nociceptive flexion reflex and the threshold was measured by an increased force. The force of paw withdrawal threshold of the control, oxi-MC-ADH-VC-treated, and untreated groups were 189.92 ± 19.97 g, 175.75 ± 233.08 g, and 251.96 ± 41.23 g, respectively (Fig. 7C). These results indicated that oxi-MC-ADH-VC treatment could decrease the withdrawal response and can improve the sensory function recovery in TBI.
Histopathological findings
Hematoxylin and eosin (H&E) staining was used to evaluate the characteristic features of the TBI. An extensive cortical hemorrhage at the injured site on the day following the surgery was noted in the untreated group but the hemorrhagic area was found to be significantly healed in the oxi-MC-ADH-VC-treated group (Fig. 8A). The results of cresyl violet staining displayed the disappearance of some Nissl substances in the untreated group as compared to the control group. In contrast, the oxi-MC-ADH-VC-treated group retained a large amount of Nissl substances (Fig. 8B). Fluoro-Jade B (FJB) is a sensitive stain to detect the degenerated neurons. As shown in Fig. 8C, the FJB-positive signals in the untreated group were conspicuously higher than those in the control and the oxi-MC-ADH-VC-treated groups. This signified that the oxi-MC-ADH-VC hydrogel could effectively reduce the neuronal degeneration. Moreover, there was a sharp increment in the activated microglia numbers in the untreated group (Fig. 8D) compared to the oxi-MC-ADH-VC-treated group. In summary, the oxi-MC-ADH-VC hydrogel efficiently and effectively prevented neuronal degeneration after the occurrence of a TBI.
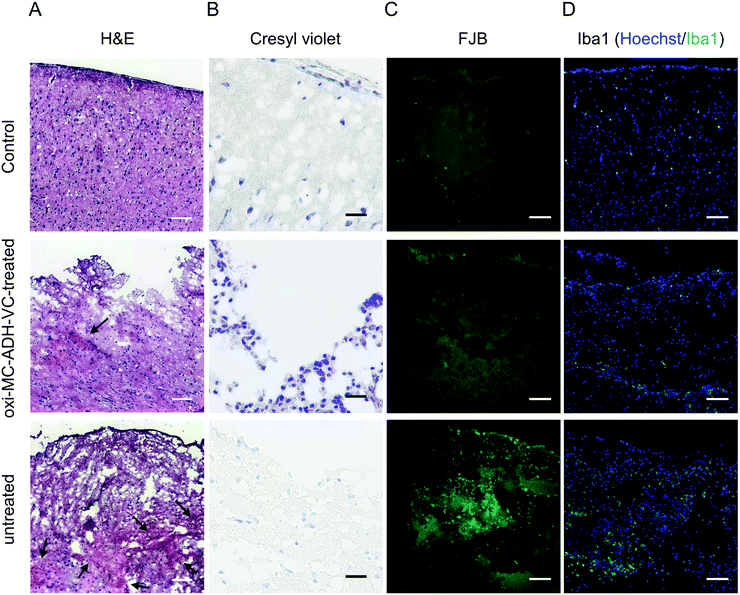 |
| Fig. 8 Histological evaluation. (A) H&E stain, the black arrow indicates the hemorrhagic area, (B) cresyl violet stain, (C) FJB, and (D) Iba1 (scale bar: 100 μm). | |
Discussion
TBI is primarily caused by external mechanical force. It initiates a biochemical cascade leading to secondary injury and generation of free radicals, which is the key influencing factor during the rehabilitation process after TBI.19,20 Various mechanisms have been described to explain the generation of free radicals causing brain damage, including glutamate release, intracellular calcium overload, increase in arachidonic acid and its metabolites, hemoglobin denaturation, and ferric ion release.21,22 The current clinical treatment of TBI primarily focuses on the maintenance of intracranial pressure by surgical procedures, followed by administration of hyperosmotic drugs to prevent further brain damage. However, the free radicals are continuously present at the injured site and harm the brain tissues, resulting in a poor prognosis.
The alternative treatment strategies for TBI treatment could be concerning the dosage and more efficient administration of vitamin C. Hydrogels are high-water content materials prepared by cross-linked polymers that are great candidates as drug carriers. Hydrogels can not only be formed in practically any shape and size according to the requirement but also are able to provide sustained local delivery of a variety of therapeutic agents.23,24 The objective of this study is development of the oxi-MC-ADH hydrogel as an antioxidant carrier for the treatment of traumatic brain injury.
MC has been previously reported as a potential therapeutic agent for the treatment of TBI as well as peripheral nerve injury.25 It remains stable within a broad range of pH from 3 to 11.26 Its acid-resistant properties make it suitable to deliver acidic antioxidants such as vitamin C. Polysaccharide oxidation by NaIO4 has been extensively applied in biomaterial research to produce active di-aldehyde groups in the C2 and C3 units of glucose.27–29 The oxi-MC contains di-aldehyde groups that react with ADH and form the hydrogel in situ by a Schiff-base reaction (Fig. S3, ESI†). The FT-IR spectrum of oxi-MC-ADH shows that the absorption band for C
O stretching disappeared due to the consumption of aldehyde to form the imine bond between oxi-MC and ADH. Moreover, the appearance of a new absorption band at 1560 cm−1 is associated with the NH functional group (Fig. S4, ESI†). The Schiff-base reaction contributes to rapid gelation time (about 6 min at 37 °C) of oxi-MC-ADH (Table 1). The results of gelation time suggest that the oxi-MC-ADH hydrogel is suitable for surgical procedures (over 10 min at 4 °C). However, the average gelation time of oxi-MC-ADH-VC at 37 °C was within 1 min, which is dependent on the reaction being catalyzed by acids.30 The oxi-MC-ADH hydrogel is a kind of high water-content material and serves as an ideal hydrophilic antioxidant carrier. In addition, the gelation property of oxi-MC-ADH is such that it can take any shape to fit in the injured site and can provide sustained-release of the antioxidant. The degradation property of the oxi-MC-ADH-VC hydrogel was utilized to mimic the in vivo physiological lysozyme digestion. The data indicated that the oxi-MC-ADH-VC hydrogel can be considered as a degradable delivery system. Further, half of the oxi-MC-ADH-VC hydrogel can degrade within 2 weeks in vitro and it is expected to degrade much faster in a dynamic physiological environment. The aim of this study was to establish a novel antioxidant carrier for the treatment of TBI, and hence, the oxi-MC-ADH-VC hydrogel was selected to directly deliver vitamin C to the TBI lesion.
Vitamin C is a powerful antioxidant; the beneficial effect of vitamin C for humans was discovered in 1932.31 Vitamin C is a well-known antioxidant used for neuroprotection. The neuroprotective actions of vitamin C were demonstrated for oxidative stress-related syndrome in the animal model such as Alzheimer's disease (AD), seizures, stroke, and brain injury.15,32–34 Vitamin C can inhibit nuclear factor (NF)-κB during inflammation and apoptosis.35 In a clinical trial with patients suffering from severe head injury, a low dose of vitamin C did not exhibit any recognizable neuroprotective effect but in high doses, it blunted the progression of perilesional edema without resulting in a better neurological outcome.15 The reason might be related to an insufficient concentration of the antioxidant being delivered to the injured site. It is noteworthy to mention here that a high dose of vitamin C can cause mortality and lead to a vegetative state.15 Therefore, drug administration requires an optimal dose to be administered by the correct route to achieve the desired therapeutic concentration.24 In this study, the dose of vitamin C was determined to achieve a target concentration of 250 μg mL−1 for the brain cells (Fig. S5, ESI†); therefore, 2.5 mg mL−1 vitamin C was incorporated within the oxi-MC-ADH-VC hydrogel (oxi-MC-ADH-VC/cell culture medium, 1/9 (v/v) for the extraction medium in the in vitro biocompatibility testing). According to Fig. 3, the cumulative release of vitamin C was >80% within 6 h and maintained a contusive release for almost one week. The initial burst-release of vitamin C served as an initial ROS scavenger after TBI and the extended-release worked to prevent secondary damages.
TBI occurrence results in inflammation, leading to mitochondrial ROS generation.36 If the ROS production exceeds the scavenging capacity of the antioxidant, it leads to irreversible cell function or cell damage.37 ROS-induced damage to DNA is an early event following TBI and occurs within minutes.38 In this study, we fixed the concentration of H2O2 at 0.6 mM to induce damage in the B35 cell line based on a preliminary concentration screening data (not shown). The B35 cells are neuroblastoma cell lines and were deemed suitable for this experiment. The gene expression profiles revealed that the oxi-MC-ADH-VC hydrogel could down-regulate the inflammation-related (COX-2, IL-1β, and TNF-α), apoptosis-related (Bcl-2 (B-cell lymphoma)), Fadd (fas-associated protein with death domain), Casp-9 (caspase-9), and ROS-related (iNOS) genes (Fig. 6). At the beginning of inflammation, the downstream transcription factor NF-κB gets activated to release TNF-α and IL-1β.39 The oxi-MC-ADH-VC hydrogel can efficiently inhibit the NF-κB-mediated inflammatory pathway, as demonstrated by the CM-H2DCFDA staining assay (Fig. 5). Our data indicate that the oxi-MC-ADH-VC hydrogel can efficiently protect the B35 cells from H2O2-induced damage. Based on these findings, we can infer that the oxi-MC-ADH-VC hydrogel exhibited good neuroprotective effects after TBI.
In order to evaluate the biocompatibility and safety of the oxi-MC-ADH hydrogel, we injected the hydrogel subcutaneously in Sprague-Dawley rats for one month. Biochemical and hematological tests were performed for safety assessment (Table S3, ESI†). For developing the in vivo animal model, Feeney's weight-dropping TBI model was used to create a reproducible lesion of 50 mm3 volume. In order to prevent an increase in the intracranial pressure by hydrogel injection, we injected only 50 μL of each hydrogel per lesion. The doses of the oxi-MC-ADH-VC hydrogel and vitamin C were calculated as per the body surface area (BSA)-based formula for converting animal doses to human equivalent dose.40 The dietary reference intake of vitamin C is 100 mg per day for adults.41 The dose of vitamin C in the rats was determined as 10 mg kg−1 per day and, therefore, the antioxidant concentration of the oxi-MC-ADH-VC hydrogel was fixed at 200 mg mL−1.
Based on the severity, TBI can lead to immediate brain damage causing several neuro-pathological dysfunctions.42 Accordingly, we used neurobehavioral function tests such as the rota-rod performance test, VersaMax animal activity monitoring system, paw pressure test, and hot-plate test to evaluate the degree of recovery from TBI. The results of the paw pressure and hot-plate tests revealed that the oxi-MC-ADH-VC hydrogel could help in the restoration of neuropathological functions. Repeating a behavioral test in the same animal can lead to bias43 and this explains the similar trend observed in all the groups in the rota-rod performance test (Fig. S6, ESI†). In addition, we investigated the neurological deficits using an mNSS scale,22 which is a severity scoring tool to assess the neurological function. The pain-related quantitative test showed a significant difference between the oxi-MC-ADH-VC-treated and untreated groups. Our histopathological data indicate that the use of oxi-MC-ADH-VC hydrogel can improve recovery from TBI, including a reduction in hemorrhage, down-regulation of microglia, and a decrease in neuronal degeneration. These findings might be attributable to the ability of vitamin C to control the inflammatory response after a TBI.44,45 The oxi-MC-ADH-VC hydrogel served as a safe biomaterial for the treatment of TBI as well as for the improvement of neurological functions.
Experimental section
Oxidized methylcellulose (oxi-MC) polymer preparation
Methylcellulose (MC) (1% w/v) (average molecular weight: ∼40
000, degree of substitution: 1.6–1.9 mol methoxy per mol cellulose, viscosity: 400 cP) (274429, Sigma, USA) was dissolved in 400 mL double-distilled water (ddH2O) at room temperature and then 40 mL of 6.47% NaIO4 (S1878, Sigma) was added gently under constant stirring for oxidation in dark for 24 h. The molar ratio between MC and NaIO4 was 1
:
1. The oxidation was stopped by ethylene glycol (0.67 mL) (324588, Sigma). The oxidized MC polymers underwent a dialysis process for 3 days to remove the incomplete reaction residues and silver nitrate (3426, J.T.Baker, USA) was used to determine the amount of unreacted NaIO4 in the dialysis buffer. The final oxi-MC product was obtained by freeze drying.
Characterization of oxi-MC
Fourier-transform infrared spectroscopy (FT-IR) and nuclear magnetic resonance spectroscopy (NMR) were used for detecting the functional groups of oxi-MC. For FT-IR, the spectra were recorded in the wavelength range between 4000 to 600 cm−1 with a resolution of 8 cm−1; for the NMR spectral assignment of protons, 5 mg of oxi-MC was dissolved in 0.7 mL of D2O (151882, Sigma) and then analyzed by an NMR instrument (400MR, Varian, USA).
The degree of oxidation was determined by 2,4,6-trinitrobenzene sulfonic acid (TNBS) assay.46 The absorbance was obtained by using a micro-plate reader (VersaMaxTM, Molecular Devices, Canada) at 340 nm.
Establishment of oxi-MC antioxidant carrier system
The oxi-MC-ADH and oxi-MC-ADH-VC hydrogel delivery system are defined in Table S1 (ESI†). Briefly, 8% oxi-MC (w/v, in PBS) was cross-linked with 4% ADH (A0638, Sigma) solution (w/v, in PBS, pH = 7.2) in a 4
:
1 volume ratio. For oxi-MC-ADH-VC preparation in the in vitro study, 4% ADH solution was mixed with 12.5 mg vitamin C (A4544, Sigma) (ADH-VC) first. For the oxi-MC-ADH-VC hydrogel fabrication in the in vivo study, 250 mg vitamin C was dissolved in 8% oxi-MC solution (oxi-MC-VC) and was cross-linked with 4% ADH solution (w/v, in PBS, pH = 7.2) in a 4
:
1 volume ratio for obtaining 200 mg mL−1 of vitamin C in the hydrogel.
Determination of gelation time
A rheometer (Discovery HR-1 hybrid, TA instruments, USA) with a 40 mm parallel plate was used to evaluate the viscoelastic properties of the hydrogels. Also, the gelation time was analyzed via an oscillation time sweep model. The storage modulus (G’) and loss modulus (G”) were recorded at 37 °C over 600 s.
Examination of degradation properties
The evaluation of degradation properties of oxi-MC-ADH-VC hydrogel was performed with digestion at 37 °C. Briefly, the oxi-MC-ADH-VC sample was soaked in PBS containing 2000 U mL−1 lysozyme (L6876, Sigma) (pH = 6.0). The volume of PBS was maintained to be 10 times greater than that of the hydrogel. The sample was collected to be freeze-dried (Wdry) at a specific time intervals for the degradation percentage calculation. The degradation percentage was calculated by the following formula: degradation percentage (%) = 100 × (Winitial − Wdry)/Winitial, where Winitial is the dry weight of the hydrogel on day 0.
The release profile
The evaluation of the release profile of oxi-MC-ADH-VC was performed in PBS in a 1
:
9 volume ratio at 37 °C. 500 μL of liquid-state oxi-MC-ADH-VC was transferred into a scintillation vial and allowed to form the hydrogel for 10 min. PBS was added to each tube and incubated at 37 °C. The incubated PBS were collected at specific time intervals and mixed with 0.136 M glycine solution (composed of 30 mM potassium dihydrogenphosphate and 0.89 mM disodium hydrogenphosphate) for determining the cumulative vitamin C release by the measurement of absorbance at 266 nm (Nano-100, Clubio, Taiwan).
In vitro biocompatibility testing
The biocompatibility assay following the ISO 10993 guidelines was carried out to evaluate the biocompatibility of the hydrogel with a rat neuroblastoma cell line (B35, CRL-2754, ATCC). Briefly, B35 cells were cultured in 10% FBS (Hyclone, USA) supplemented with high-glucose DMEM (Gibco, USA) and seeded with a density of 4 × 103 cells per well in a 96-well tissue culture plate at 37 °C under 5% CO2 for 24 h. The culture medium was replaced with fresh culture medium (control group) or extraction medium and incubated for 72 h. The biocompatibility assay was performed by the Cell Proliferation Reagent WST-1 (11644807001, Roche, USA) and the Cytotoxicity Detection KitPLUS (LDH, 04744934001, Roche), following the manufacturer's instructions. The data was determined by an ELISA reader. The percentage of cytotoxicity was calculated by the following formula: cytotoxicity (%) = 100 × (ODexperiment − ODcontrol)/(ODtotal-lysis − ODcontrol).
The cell viability assay was evaluated via the Live/Dead staining kit (L3324, ThermoFisher Scientific, USA) by staining the cells with calcein AM (Ex/Em: 494/517 nm) and ethidium homodimer (Ex/Em: 528/617 nm) for 10 min at room temperature. The labeled cells were observed by a fluorescence microscope (TS100, Nikon, Japan).
ROS determination
The ROS determination in the B35 cells was performed by CM-H2DCFDA (chloromethyl-2′,7′-dichlorofluorescein diacetate) (C6827, ThermoFisher Scientific). In brief, B35 cells were seeded with a density of 1 × 104 cells per well in a 12-well tissue culture plate for 24 h and then treated with 0.6 mM H2O2 for 1 h to induce ROS damage. After ROS induction, oxi-MC-ADH-VC hydrogel was co-cultured with the damaged cells via the Transwell® culture plate insert (3460, Corning, USA) for 24 h. After 24 h treatment, the cells were stained with 10 μM CM-H2DCFDA (Ex/Em: 502/523 nm) and 0.5 μg mL−1 Hoechst 33342 (Ex/Em: 350/461 nm, ThermoFisher Scientific) at room temperature for 20 min and observed by a fluorescence microscope (TS100, Nikon, Japan). The groups were defined as the control group, H2O2 group (ROS-induced), H2O2 + oxi-MC-ADH group (ROS-induced and treated with oxi-MC-ADH hydrogel), and H2O2 + oxi-MC-ADH-VC group (ROS-induced and treated with oxi-MC-ADH-VC hydrogel).
Gene expression
The B35 cells sustained ROS damage and were treated with the oxi-MC-ADH-VC hydrogel for 48 h, as described previously. Total RNA extracted via a Direct-zol™ RNA MiniPrep Kit (R2052, Zymo Research, USA), according to the manufacturer's instructions, was quantified by the concentration corresponding to the absorbance at 260 nm by a micro-spectrophotometer (Nano-100). The RNA was reverse-transcribed into cDNA by using the High capacity cDNA reverse transcription kit (Life Technologies Corporation, USA). The real-time PCR assays were performed by the TaqMan® method on an ABI-7900 System (Life Technologies Corporation, USA) to evaluate the gene expression, including the oxidation stress related gene (iNos, nNos, and eNos), the inflammatory response related gene (COX-2, IL-1β, and TNF-α), and the apoptosis related gene (Bcl-2, Fadd, and Casp-9). According to the ΔΔCT method, the relative mRNA quantity was normalized by GAPDH.
In vivo TBI model
The animal study was approved by the Institutional Animal Care and Use Committee of National Health Research Institutes (NHRI-IACUC-104124). Male Sprague–Dawley rats (200–300 g) were anaesthetized with isoflurane and a 5 mm diameter craniotomy was performed over the right parietal cortex. Briefly, the cortical defect was accomplished by a 300 g metal object falling down from 25 cm height and hitting with a rounded metal tip (2.5 mm diameter), resulting in a deformation under the dura of 4 mm depth. The whole procedure is shown in Fig. S7 (ESI†). For the oxi-MC-ADH-VC-treated TBI-rats’ (oxi-MC-ADH-VC-treated) group, 40 μL oxi-MC-VC and 10 μL 4% ADH were mixed and injected onto the injury site immediately for gelation. In addition, the untreated TBI-rats’ (untreated) group received 50 μL PBS only; on the contrary, the control group (control) did not receive any surgical operation.
Behavioral recovery was evaluated using mNSS test on days 3, 7, 14, 21, and 28 post-TBI-surgery (shown in Table S2, ESI†).47 The hot plate test measured the thermal pain reflexes with a constant-temperature rise up to 50 °C by using an Incremental Hot/Cold Plate Analgesia Meter (IITC Life Sciences, CA) and the elicited responses were recorded on day 21. The Randall–Selitto test (Paw pressure test) and Digital Paw Pressure Randall Selitto Meter (IITC Life Science, CA) were also evaluated on day 21. An increasing pressure was applied via the probe on the dorsal surface up to a maximum of 300 g.
Histological evaluation
All experimental animals were sacrificed by transcardial perfusion with PBS, the brain tissues were collected, and post-fixed overnight by 4% paraformaldehyde at 4 °C. After dehydration with 30% sucrose solution, the brain tissues were cut into 5 μm thick tissue sections using a cryostat (CM3050S, Leica, Germany) and received the H&E stain,48 cresyl violet stain, FJB (AG310, Merck, Germany) (Ex/Em: 480/525 nm) stain, and immune-fluorescent stain. The H&E and FJB staining were done following the routine histology process. For cresyl violet staining, the slides were stained by 0.012% cresyl violet (C5042, Sigma) dye solution at 60 °C for 20 min. For immune-fluorescent stain, all the sections were rehydrated in PBS and fixed in 4% paraformaldehyde for 20 min. The sections were washed 3 times in Tris-buffered saline (TBS) for 5 min each time, and blocked for 60 min in TBS containing 0.2% Triton X-100 (TBS-TX) and 3% horse serum (HS). The sections were blocked at 25 °C for 1 h with primary antibody (Iba1, 1
:
100) (019-19741, Wako) in TBS-TX containing 1% HS and washed 3 times by TBS. The sections were incubated with a secondary antibody (Alexa Fluor® 488 Donkey anti-rabbit IgG) (406416, Biolegend) at 1
:
200 dilution in TBS containing 1% HS at 25 °C for 1 h and washed 3 times in TBS (5 min each time). The sections were coverslipped with the mounting media and observed under a fluorescence microscope (TS100, Nikon, Japan).
Statistical analysis
All the results were conducted at least in triplicate and reported as mean ± standard deviation. Statistical analysis was performed by using one-way ANOVA. The p-value < 0.05 was considered statistically significant.
Conclusions
We successfully prepared oxi-MC-ADH-VC as a vitamin C carrier for TBI treatment. The vitamin C was delivered to the brain lesion site with a release profile adequate to match the clinical needs. The results can provide an alternative therapeutic strategy for TBI treatment during emergency. The initial burst of vitamin C from oxi-MC-ADH-VC hydrogel could quickly remove ROS from the injured site after an acute TBI. The later 3-day sustained release could prevent secondary damage to damaged brain sites that might lead to more serious sequelae. This oxi-MC-ADH-VC hydrogel can open up a new avenue for the clinical management of TBI.
Conflicts of interest
There are no conflicts to declare.
Acknowledgements
Che-Yung Kuan carried out his thesis research under the auspices of the PhD Program in Tissue Engineering and Regenerative Medicine, National Chung Hsing University and National Health Research Institutes. This work was supported by the National Health Research Institutes (grant numbers: BN-106-PP-01 and BN-108-PP-01).
Notes and references
- S. Barker-Collo, A. Theadom, K. Jones, N. Starkey, M. Kahan and V. Feigin, Brain Inj., 2018, 32, 1651–1658 CrossRef PubMed
.
- H. H. Dash and S. Chavali, Korean J. Anesthesiol., 2018, 71, 12–21 CrossRef PubMed
.
-
H. Krawczyk and A. Targowski, Issues in Informing Science & Information Technology, 2018, vol. 15, pp. 97–108 Search PubMed
.
- X. Ge, J. Yu, S. Huang, Z. Yin, Z. Han, F. Chen, Z. Wang, J. Zhang and P. Lei, J. Neurosci. Methods, 2018, 308, 162–172 CrossRef PubMed
.
- K. Zhang, Z. Shi, J. Zhou, Q. Xing, S. Ma, Q. Li, Y. Zhang, M. Yao, X. Wang and Q. Li, J. Mater. Chem. B, 2018, 6, 2982–2992 RSC
.
- R. P. Anada, K. T. Wong, J. J. Jayapalan, O. H. Hashim and D. Ganesan, Electrophoresis, 2018, 39, 2308–2315 CrossRef CAS PubMed
.
- T. Murthy, P. Bhatia, K. Sandhu, T. Prabhakar and R. L. Gogna, Indian J. Neurotrauma, 2005, 2, 7–12 CrossRef
.
- K. N. Corps, T. L. Roth and D. B. McGavern, JAMA Neurol., 2015, 72, 355–362 CrossRef PubMed
.
- V. Lobo, A. Patil, A. Phatak and N. Chandra, Pharmacogn. Rev., 2010, 4, 118–126 CrossRef CAS PubMed
.
- Q. Shen, J. B. Hiebert, J. Hartwell, A. R. Thimmesch and J. D. Pierce, Worldviews Evid. Based Nurs., 2016, 13, 380–389 CrossRef PubMed
.
- C. C. Huang, Z. X. Liao, D. Y. Chen, C. W. Hsiao, Y. Chang and H. W. Sung, Adv. Healthcare Mater., 2014, 3, 1133–1148 CrossRef CAS PubMed
.
- M. K. Bain, D. Maity, B. Bhowmick, D. Mondal, M. R. Mollick, G. Sarkar, M. Bhowmik, D. Rana and D. Chattopadhyay, Carbohydr. Polym., 2013, 91, 529–536 CrossRef CAS PubMed
.
- G. T. Gold, D. M. Varma, P. J. Taub and S. B. Nicoll, Carbohydr. Polym., 2015, 134, 497–507 CrossRef CAS PubMed
.
- N. Law, B. Doney, H. Glover, Y. Qin, Z. M. Aman, T. B. Sercombe, L. J. Liew, R. J. Dilley and B. J. Doyle, J. Mech. Behav. Biomed. Mater., 2018, 77, 389–399 CrossRef CAS PubMed
.
- A. Razmkon, A. Sadidi, E. Sherafat-Kazemzadeh, A. Mehrafshan, M. Jamali, B. Malekpour and M. Saghafinia, Clin. Neurosurg., 2011, 58, 133–137 CrossRef
.
- M. Allahtavakoli, F. Amin, A. Esmaeeli-Nadimi, A. Shamsizadeh, M. Kazemi-Arababadi and D. Kennedy, Basic Clin. Pharmacol. Toxicol., 2015, 117, 335–339 CrossRef CAS PubMed
.
- S. Sil, T. Ghosh, P. Gupta, R. Ghosh, S. N. Kabir and A. Roy, J. Mol. Neurosci., 2016, 60, 421–435 CrossRef CAS PubMed
.
- B. Kamali manesh, E. Mohebi, H. Azhdari Zarmehri, A. Shamsizadeh and M. Mohammad-Zadeh, Physiol. Pharmacol., 2018, 22, 103–108 Search PubMed
.
- K. M. O’Connell and M. T. Littleton-Kearney, Biol. Res. Nurs., 2013, 15, 253–263 CrossRef
.
- J. Y. Lee, S. Acosta, J. P. Tuazon, K. Xu, H. Nguyen, T. Lippert, M. G. Liska, A. Semechkin, I. Garitaonandia, R. Gonzalez, R. Kern and C. V. Borlongan, Theranostics, 2019, 9, 1029–1046 CrossRef PubMed
.
- E. Moor, E. Shohami, E. Kanevsky, N. Grigoriadis, C. Symeonidou and R. Kohen, Exp. Gerontol., 2006, 41, 303–311 CrossRef CAS PubMed
.
- Y. Xiong, A. Mahmood and M. Chopp, Nat. Rev. Neurosci., 2013, 14, 128–142 CrossRef CAS PubMed
.
- N. Bhattarai, J. Gunn and M. Zhang, Adv. Drug Delivery Rev., 2010, 62, 83–99 CrossRef CAS PubMed
.
- J. Li and D. J. Mooney, Nat. Rev. Mater., 2016, 1, 16071 CrossRef CAS PubMed
.
- D. Gupta, C. H. Tator and M. S. Shoichet, Biomaterials, 2006, 27, 2370–2379 CrossRef CAS PubMed
.
- P. Nasatto, F. Pignon, J. Silveira, M. Duarte, M. Noseda and M. Rinaudo, Polymers, 2015, 7, 777 CrossRef CAS
.
- M. H. Hu, K. C. Yang, Y. H. Sun, Y. C. Chen, S. H. Yang and F. H. Lin, Eur. Cells Mater., 2017, 34, 307–320 CrossRef CAS PubMed
.
- L. Li, C. Wang, Q. Huang, J. Xiao, Q. Zhang and Y. Cheng, J. Mater. Chem. B, 2018, 6, 2474–2480 RSC
.
- M. K. Khan, J. Luo, Z. Wang, R. Khan, X. Chen and Y. Wan, J. Mater. Chem. B, 2018, 6, 1640–1649 RSC
.
- S. Afrin Dalia, F. Afsan, S. Hossain, N. Khan, C. Zakaria, K. Zahan and M. Ali, Int. J. Chem. Stud., 2018, 6, 2859–2866 Search PubMed
.
-
A. Dasgupta and K. Klein, Antioxidants in Food, Vitamins and Supplements, Elsevier, San Diego, 2014, pp. 277–294 Search PubMed
.
- J. Huang, D. B. Agus, C. J. Winfree, S. Kiss, W. J. Mack, R. A. McTaggart, T. F. Choudhri, L. J. Kim, J. Mocco, D. J. Pinsky, W. D. Fox, R. J. Israel, T. A. Boyd, D. W. Golde and E. S. Connolly, Jr., Proc. Natl. Acad. Sci. U. S. A., 2001, 98, 11720–11724 CrossRef CAS PubMed
.
- C. Behl and B. Moosmann, Free Radicals Biol. Med., 2002, 33, 182–191 CrossRef CAS PubMed
.
- L. F. Santos, R. L. Freitas, S. M. Xavier, G. B. Saldanha and R. M. Freitas, Pharmacol., Biochem. Behav., 2008, 89, 1–5 CrossRef CAS PubMed
.
- X. Y. Zhang, Z. P. Xu, W. Wang, J. B. Cao, Q. Fu, W. X. Zhao, Y. Li, X. L. Huo, L. M. Zhang, Y. F. Li and W. D. Mi, Int. Immunopharmacol., 2018, 65, 438–447 CrossRef CAS PubMed
.
- A. Sharma, K. Liaw, R. Sharma, Z. Zhang, S. Kannan and R. M. Kannan, Theranostics, 2018, 8, 5529–5547 CrossRef CAS PubMed
.
- M. Bains and E. D. Hall, Biochim. Biophys. Acta, 2012, 1822, 675–684 CrossRef CAS PubMed
.
- A. Mendes Arent, L. F. d. Souza, R. Walz and A. L. Dafre, BioMed Res. Int., 2014, 2014, 723060 Search PubMed
.
- D. Brites, Front. Pharmacol., 2012, 3, 88 Search PubMed
.
- S. Reagan-Shaw, M. Nihal and N. Ahmad, FASEB J., 2008, 22, 659–661 CrossRef CAS PubMed
.
- K. A. Naidu, Nutr. J., 2003, 2, 7 CrossRef PubMed
.
- E. D. Bigler, Front. Hum. Neurosci., 2013, 7, 395 Search PubMed
.
- A. Hanell and N. Marklund, Front. Behav. Neurosci., 2014, 8, 252 Search PubMed
.
- C. C. Portugal, R. Socodato, T. Canedo, C. M. Silva, T. Martins, V. S. Coreixas, E. C. Loiola, B. Gess, D. Rohr, A. R. Santiago, P. Young, R. D. Minshall, R. Paes-de-Carvalho, A. F. Ambrosio and J. B. Relvas, Sci. Signaling, 2017, 10, eaal2005 CrossRef PubMed
.
- J. Wang and S. Dore, J. Cereb. Blood Flow Metab., 2007, 27, 894–908 CrossRef CAS PubMed
.
- Y. C. Chen, W. Y. Su, S. H. Yang, A. Gefen and F. H. Lin, Acta Biomater., 2013, 9, 5181–5193 CrossRef CAS PubMed
.
- S. F. Chen, C. W. Hsu, W. H. Huang and J. Y. Wang, Br. J. Pharmacol., 2008, 155, 1279–1296 CrossRef CAS PubMed
.
- B. Zihayat, A. Khodadadi, M. Torabi, M. Mehdipour, M. Basiri and M. Asadi-Shekarri, Biomed. Eng., 2018, 30, 1850015 Search PubMed
.
Footnote |
† Electronic supplementary information (ESI) available. See DOI: 10.1039/c9tb00816k |
|
This journal is © The Royal Society of Chemistry 2019 |