Cationized Bombyx mori silk fibroin as a delivery carrier of the VEGF165–Ang-1 coexpression plasmid for dermal tissue regeneration
Received
31st May 2018
, Accepted 18th November 2018
First published on 19th November 2018
Abstract
The angiogenesis of an implanted construct is among the most important issues in tissue engineering. In this study, spermine was used to modify Bombyx mori silk fibroin (BSF) to synthesize cationized BSF (CBSF). BSF and CBSF were coated in sequence on the surface of polyethyleneimine (PEI)/vascular endothelial growth factor 165/angiopoietin-1 coexpression plasmid DNA (pDNA) complexes to form CBSF/BSF/PEI/pDNA quaternary complexes. BSF scaffolds loaded with carrier/pDNA complexes were prepared as dermal regeneration scaffolds by freeze-drying. In one set of experiments, scaffolds were used to cover a chick embryo chorioallantoic membrane (CAM) to investigate the influence of carrier/pDNA complexes on angiogenesis; in another set of experiments, scaffolds were implanted into dorsal full-thickness wounds in Sprague-Dawley rats to evaluate the effect of carrier/pDNA complex-loaded BSF scaffolds on neovascularization and dermal tissue regeneration. After modification with spermine, the surface zeta potential value of BSF rose to +11 mV from an initial value of −9 mV, and the isoelectric point of BSF increased from 4.20 to 9.04. The in vitro transfection of human umbilical vein endothelial cells (EA.hy926) with quaternary complexes revealed that the CBSF/BSF/PEI/pDNA complexes clearly exhibited lower cytotoxicity and higher transfection efficiency than the PEI/pDNA complexes. The CAM assay showed a more abundant branching pattern of blood vessels in BSF scaffolds loaded with CBSF/BSF/PEI/pDNA complexes than in BSF scaffolds without complexes or loaded with PEI/pDNA complexes. The in vivo experimental results demonstrated that the incorporation of CBSF/BSF/PEI/pDNA complexes could effectively enhance angiogenesis in the implanted BSF scaffolds, thereby promoting the regeneration of dermal tissue, providing a new scaffold for the regeneration of dermal tissue and other tissues containing blood vessels.
1. Introduction
The fate of scaffold-based tissue constructs is crucially dependent on vascularization after implantation, which determines their survival and function at defect sites.1 To supply cells with oxygen and nutrients, rapid generation of vascular networks in scaffolds is needed. Unfortunately, it usually takes several weeks for a construct to become fully vascularized.2 In recent years, various vascularization strategies have been introduced in the field of tissue engineering, such as modification of the structural and physicochemical properties of scaffolds, the activation of biological scaffolds with growth factor-releasing systems or gene carriers, and the in vitro or in vivo prevascularization of scaffolds.3,4 Among these strategies, scaffolds loaded with angiogenic factors such as the vascular endothelial growth factor (VEGF),5 angiopoietin-1 (Ang-1),6 platelet-derived growth factor (PDGF),7 basic fibroblast growth factor (bFGF)8 and transforming growth factor (TGF)9 can promote vascularization and tissue regeneration to different degrees. VEGF and Ang-1 are the most effective angiogenic growth factors that act directly on endothelial cells.10 VEGF induces the formation of new blood vessels by promoting cell proliferation and vascular permeability, and Ang-1 promotes the maturation of new vessel networks by sustaining the survival of endothelial cells, preventing VEGF-induced permeability and recruiting pericytes to the vessel wall.11 However, a major limitation of the direct application of exogenous growth factors is their short active half-life due to extensive degradation and inactivation.12
Therapies with genes encoding angiogenic growth factors, rather than the protein itself, enable the in situ expression of growth factors at defect sites. This approach provides an alternative to exogenous growth factors, which are vulnerable to degradation, inactivation and elimination by the immune system after entering the body. A bilayer porous collagen–chitosan/silicone membrane dermal scaffold loaded with pDNA encoding VEGF-165 and N,N,N-trimethyl chitosan chloride complexes was transplanted into full-thickness burn wounds in miniature pigs, and the scaffolds loaded with pDNA complexes had a significantly higher number of newly formed and mature blood vessels and faster regeneration of the dermis.13 When collagen–chitosan laser drilling acellular dermal matrix composite scaffolds containing microencapsulated VEGF-165 gene-modified human umbilical cord mesenchymal stromal cells were implanted into skin defect wounds in miniature pigs, the VEGF expression increased, and these scaffolds achieved a heightened degree of vascularization.14 Heparin-modified pluronic nanogels encapsulated with bFGF and loaded with pDNA encoding VEGF165 were delivered into endothelial progenitor cells, which were then able to promote endothelial cell differentiation and neovascularization in an ischemic limb model system.15
Gene delivery systems have attracted a great deal of attention in recent years and include both viral and nonviral delivery systems.16,17 Due to the limitations of viral vectors, such as immunogenicity, oncogenicity, high cost and restrictions on the size of the DNA, nonviral carriers are considered preferable for gene delivery.18 Several cationic polymers such as cationic lipids, dextran, chitosan, poly(amino ester), poly(L-lysine) and polyamidoamine have been investigated.19 Among them, polyethyleneimine (PEI) has attracted significant interest because of its high transfection efficiency and its intrinsic endosomolytic activity.20 The strong buffering capacity of PEI can facilitate the endosomal escape of pDNA by rupturing the endosome membrane via the “proton sponge” effect.21,22 However, the gene transfection efficiency and cytotoxicity of PEI are strongly correlated with its chain length and topology.23,24 High-molecular-weight PEIs (25 kDa) are effective in condensing nucleic acids but exhibit pronounced cytotoxicity and induce membrane damage in the initial stage of treatment25 followed by mitochondrially mediated apoptosis in the later stages. Meanwhile, low-molecular-weight PEIs, which have buffering capacity equivalent to that of their longer chain counterparts, are nearly nontoxic but display low transfection efficiency owing to inefficient pDNA condensation and low cellular uptake caused by limited charge-mediated interactions.26 Therefore, a key challenge for nonviral gene delivery systems is to develop an appropriate delivery carrier that not only effectively transfers therapeutic DNA into the targeted cells to achieve efficient and stable expression of the target gene but also exhibits biodegradability and a lack of cytotoxicity.
Bombyx mori silk fibroin (BSF) is a natural protein produced by the silkworm that has been widely used in tissue engineering scaffolds27,28 and as a delivery carrier for bioactive molecules29,30 due to its excellent biocompatibility and biodegradability.31,32 In our previous studies, we used Antheraea pernyi silk fibroin (ASF) to coat PEI/pDNA complexes and transfected these ASF/PEI/pDNA complexes into L929, HEK293, and HCT 116 cells.33,34 The results revealed that ASF could effectively reduce the cytotoxicity of PEI/pDNA complexes by shielding the excess positive charge of PEI and could also improve transfection efficiency. Silk fibroin microcapsules loaded with pDNA could transfect NIH/3T3 fibroblasts with lower cytotoxicity than that of pDNA/PEI complexes, as reported by Li et al.35 Compared with that of ASF, the molecular conformation of BSF is less affected by ambient temperature and ion concentration, and the structure and properties of BSF could be more stable in the physiological environment if BSF can be used as a gene delivery carrier, providing great potential for practical applications.
We hypothesized that spermine could be covalently grafted onto the side chain of BSF in the presence of carbodiimide to obtained cationized BSF (CBSF), changing the surface charge of BSF from negative to positive. The successive coating of BSF and CBSF on the surface of complexes of PEI with pDNA encoding VEGF165 and Ang-1 could shield the excess positive charge on the PEI/pDNA complex surface and endow the resulting CBSF/BSF/PEI/pDNA complexes with an appropriate positive charge, not only reducing the cytotoxicity of the PEI/pDNA complexes but also improving their transfection efficiency when the outermost layer of the complexes was Bombyx mori silk fibroin. BSF scaffolds loaded with CBSF/BSF/PEI/pDNA quaternary complexes could accelerate the formation of blood vessels in vivo and thereby promote the regeneration of dermal tissue. Based on this hypothesis, in this study, CBSF was synthesized by grafting spermine onto the side chain of BSF with the mediation of carbodiimide via an amidation reaction between the amino groups in the spermine and the carboxyl groups in the BSF. BSF and CBSF were coated in sequence on the surface of PEI/pDNA binary complexes to form CBSF/BSF/PEI/pDNA quaternary complexes. These complexes were characterized in terms of particle size, zeta potential, surface morphology, cell viability and in vitro transfection efficiency in human umbilical vein endothelial cells (EA.hy926). Then, porous BSF scaffolds loaded with CBSF/BSF/PEI/pDNA complexes were prepared as dermal regeneration scaffolds by freeze-drying. In one set of experiments, the scaffolds were used to cover a chick embryo chorioallantoic membrane (CAM) to investigate the influence of carrier/pDNA complexes on angiogenesis; in another set, the scaffolds were implanted into dorsal full-thickness wounds in Sprague-Dawley rats to evaluate the effect of the carrier/pDNA complex loaded-BSF scaffolds on neovascularization and dermal tissue regeneration.
2. Materials and methods
2.1 Preparation of BSF solution
Regenerated BSF solution was prepared following the procedure described previously.27Bombyx mori silk fibers (Huzhou, China) were degummed three times in 0.05% Na2CO3 solution at 98–100 °C for 30 min and dried at 60 °C after thorough rinsing. The extracted BSF was dissolved in a ternary solvent of CaCl2
:
CH3CH2OH
:
H2O (1
:
2
:
8 M ratio) at 72 ± 2 °C for 1 h. The BSF solution was obtained after dialysis with cellulose membranes (MWCO 9–12 kDa) in deionized water for 4 days.
2.2 Synthesis of CBSF
First, 50 mL of BSF solution (10 mg mL−1) was stirred in an ice bath. Spermine (Sigma-Aldrich) was then added to the solution at 0.1, 0.5, 2.0, 5.0, 10.0 and 15.0% of the BSF weight in solution. The pH of the mixture was adjusted to approximately 5.6 by the addition of 2-morpholinoethanesulfonic acid (MES, Sigma-Aldrich). 1-Ethyl-3-(3-dimethyl-aminopropyl)carbodiimide hydrochloride (EDC, Sigma-Aldrich) and N-hydroxysuccinimide (NHS, Sigma-Aldrich) were added to the mixture at 20% and 10% of the weight of BSF in solution, respectively. The mixture was then stirred for 24 h at 4 °C. Finally, the CBSF solution was obtained by dialysis against deionized water at 4 °C for 72 h to remove excess reagents.
2.3 Characterization of CBSF
The zeta potential of CBSF at different weight ratios of spermine with respect to BSF was measured in triplicate using a zeta potential analyzer (Malvern Zetasizer Nano ZS90; Malvern Instruments, Malvern, UK) at 25 °C. The zeta potential of BSF and CBSF was measured at pH values ranging from 3 to 10. The pH of the solutions was adjusted using 0.1 M hydrochloric acid and 0.1 M sodium hydroxide.
BSF, CBSF (modified with 5% spermine as a proportion of BSF) and spermine solutions were dried at 25 °C and cut into particles 40 μm wide. Approximately 1 mg of particles was pressed into a pellet with 200 mg of potassium bromide. Fourier transform infrared (FTIR) analysis was performed with a Nicolet 5700 spectrometer (Nicolet Company, USA). The measurements were taken in the range of 500–4000 cm−1.
2.4 Plasmid DNA production
Plasmid DNA encoding VEGF165, Ang-1 and green fluorescent protein (GFP) were obtained according to previously described procedures.33 Primers were designed based on the conserved sequences of VEGF165 and Ang-1. The primers for the ends of VEGF165 introduced Bgl II and Sal I restriction enzyme sites. The primers for the ends of Ang-1 introduced Not I and Xho I sites. The VEGF165 and Ang-1 fragments were amplified by PCR from pAdTrackCMV-Ang-1-IRES-VEGF165, which contains the VEGF165 and Ang-1 fragments. The products were identified by agarose electrophoresis, and the PCR product was double digested with restriction enzymes. The no-load pAdTrack-CMV-PolyA-promoter plasmid that was created before the experiment was reused after gel extraction. The products were joined by incubation with T4 DNA ligase overnight at 4 °C, thus creating pAdTrack CMV-VEGF165-PolyA-promoter-Ang-1 dual genetic recombination pDNA. The pDNA was amplified in Escherichia coli DH5α cells and purified using a Qiagen Plasmid Mega Kit (Qiagen, Chatsworth, CA, USA). The concentration of pDNA was determined by measuring the ultraviolet (UV) absorbance at 260 nm with a Nanodrop 2000 UV spectrophotometer (Thermo Fisher Scientific, Waltham, MA, USA).
2.5 Preparation of CBSF/BSF/PEI/pDNA complexes
The PEI (Sigma-Aldrich, 25 kDa) solution was adjusted to 1 μg μL−1 with deionized water and passed through a 0.22 μm pore size filter prior to mixing with pDNA solution (140 ng μL−1). To prepare PEI/pDNA complexes, 2 μg of pDNA in solution was mixed with 10 μg of PEI in solution. The mixture was gently vortexed for 30 s and incubated for 30 min at 4 °C. The resulting PEI/pDNA (10/2) complexes were separately mixed with 75, 100, 150, 200, 250 or 300 μg of BSF in solution (5 μg μL−1) and then gently vortexed for 30 s and incubated for 30 min at 4 °C to form BSF/PEI/pDNA (75/10/2, 100/10/2, 150/10/2, 200/10/2 and 300/10/2) ternary complexes. Finally, the resulting BSF/PEI/pDNA complexes were each mixed with 20 μg of CBSF in solution (1 μg μL−1) by gently vortexing for 30 s and subsequently incubating for 30 min at 4 °C to form CBSF/BSF/PEI/pDNA (20/75/10/2, 20/100/10/2, 20/150/10/2, 20/200/10/2 and 20/300/10/2) complexes. PEI/pDNA (10/2) complexes were prepared as a control group.
2.6 Gel retardation assay
The binding of CBSF, BSF, PEI and pDNA was investigated by agarose gel retardation electrophoresis. Complex suspensions of CBSF/BSF/PEI/pDNA (weight ratios 20/75/10/2, 20/100/10/2, 20/150/10/2, 20/200/10/2 and 20/300/10/2) and PEI/pDNA (weight ratio 10/2) were prepared according to the procedure described above. These complex suspensions were loaded onto a 1.0 wt% agarose gel with ethidium bromide (0.5 μg mL−1) and run with Tris–acetate buffer at 100 V for 30 min. Finally, the results were observed by irradiation under UV light.
2.7 Characterization of CBSF/BSF/PEI/pDNA complexes
The suspensions of PEI/pDNA and CBSF/BSF/PEI/pDNA complexes were tested in triplicate for particle size and surface charge using a zeta potential analyzer at 25 °C. The morphology of the complexes was observed by scanning electron microscopy (SEM) (Hitachi S-4800; Hitachi Ltd, Tokyo, Japan).
2.8
In vitro gene transfection
EA.hy926 cells were seeded in glass-bottomed cell culture dishes (NEST, Lot no. 2013008) at a density of 1 × 105 cells per dish and allowed to reach 80% confluence. Complex suspensions of CBSF/BSF/PEI/pDNA (weight ratios 20/75/10/2, 20/100/10/2, 20/150/10/2, 20/200/10/2 and 20/300/10/2) and PEI/pDNA (weight ratio 10/2) were freshly prepared before transfection, and the medium in each well was replaced by fresh serum-free DMEM. The complexes (4.0 μg mL−1) were added to the dishes with serum-free DMEM, and the cells were incubated with the complexes at 37 °C in an atmosphere containing 5% CO2 for 4 h. The medium was then removed and replaced with a complete medium. After 24 h, the expression of GFP in the cells was observed by confocal laser scanning microscopy (FV1000; Olympus, Tokyo, Japan). The relative mean fluorescence intensity (MFI) of the green fluorescence images of EA.hy926 cells transfected with PEI/pDNA complexes at a weight ratio of 10/2 and with CBSF/BSF/PEI/pDNA complexes at weight ratios of 20/75/10/2, 20/100/10/2, 20/150/10/2, 20/200/10/2, 20/250/10/2 and 20/300/10/2 for 24 h, which indicated the expression levels of GFP in the cells, was quantitatively analysed using ImageJ software (NIH, Bethesda, MD, USA).36,37
2.9 Cytotoxicity assay
The cytotoxicity was quantitatively evaluated by a Cell Count Kit-8 assay (CCK-8, Beyotime, China). The EA.hy926 cells were seeded in 96-well plates at a density of 5 × 103 cells per well and allowed to attach overnight. Then, the cells were cocultured with PEI/pDNA (10/2) or CBSF/BSF/PEI/pDNA (20/150/10/2) complexes (4.0 μg mL−1) in serum-free Dulbecco's modified Eagle medium (DMEM, Gibco, USA). Cells cultured with no complexes were used as a control group. After 4 h, the medium was replaced with 200 μL of fresh DMEM containing 10% fetal bovine serum (FBS, Gibco, USA) for an additional 1 day or 2 days. Then, the medium was removed, 500 μL of culture medium containing 50 μL of CCK-8 solution was added to each well, and the cells were incubated at 37 °C for 4 h. The culture medium containing soluble formazan derivatives was transferred into a centrifuge tube and centrifuged at 1000 rpm for 10 min. The absorbance at 450 nm was measured using a microplate reader (Bio-Tek Synergy HT, USA). The relative cell viability (%) was calculated according to eqn (1), where OD450(sample) was obtained in the presence of carrier/pDNA complexes, and OD450(control) was obtained in the absence of carrier/pDNA complexes. |  | (1) |
2.10 Incorporation of carrier/pDNA complexes into BSF scaffolds
The three-dimensional BSF scaffolds were prepared according to the procedures described previously.38 In short, the BSF solution prepared according to Section 2.1 was diluted to 1.5 wt%, and MES, NHS and EDC were then added to the BSF solution at 20%, 10% and 20% of the BSF weight in solution, respectively. The mixed solution was stirred slowly and allowed to react in an ice bath for 1 h. Subsequently, the EDC-activated BSF solution was poured into stainless steel vessels (2 mm thickness) and frozen at −40 °C for 8 h, followed by lyophilization for 48 h to obtain porous BSF scaffolds. To introduce the carrier/pDNA complexes into the BSF scaffolds, 0.5 mL of sterilized phosphate buffer (PBS, pH 7.4) containing PEI/pDNA (10/2) or CBSF/BSF/PEI/pDNA (20/150/10/2) complexes (4.0 μg mL−1) was dropped onto the dried BSF scaffolds, which were then kept at 4 °C overnight to facilitate the complete incorporation of the complexes and subsequently lyophilized to obtain carrier/pDNA complex-loaded BSF scaffolds.
2.11 The morphologies of the cells on the BSF scaffolds
To investigate the morphologies of EA.hy926 cells on the BSF scaffolds loaded with PEI/pDNA (10/2) or CBSF/BSF/PEI/pDNA (20/150/10/2) complexes, BSF scaffolds prepared as described in Section 2.10 were seeded with EA.hy926 cells at a density of 5 × 104 cells per well and cultured at 37 °C in an atmosphere containing 5% CO2 for 48 h. BSF scaffolds without complexes were used as a control group. After 48 h, the scaffolds were washed three times with PBS and then fixed in 2.5 wt% glutaraldehyde (Sigma-Aldrich) at 37 °C for 2 h. The fixed samples were dehydrated by immersion in an alcohol/water gradient (50%, 75%, 90% and 100%) and then lyophilized. The morphologies of the cells on the scaffolds were observed by SEM after sputter-coating with gold.
2.12 CAM angiogenesis assay
Fertilized chicken eggs (Xuzhou, China) were placed in tissue culture dishes after 3 days of incubation in a 38 °C ventilated, humid egg incubator and then incubated in a sterile humid incubator at 37 °C for another 8 days. A 10 mm × 10 mm window was created along the horizontal axis of the eggs by cutting and removing the shell, and BSF scaffolds without complexes, scaffolds loaded with PEI/pDNA (10/2) complexes and scaffolds loaded with CBSF/BSF/PEI/pDNA (20/150/10/2) complexes were immediately used to cover the CAMs. The windows were sealed with Scotch Magic Tape, and the eggs were returned to the incubator. After 4 days, the tape was removed from the windows. The CAMs with BSF scaffolds were cut and fixed in 4% paraformaldehyde for 40 min before imaging with a high-definition camera (Canon PowerShot A560). CAM angiogenesis area rates were calculated using Image-Pro Plus 6.0 image analysis software. The neovascularization ratio (VA/CAM%) was defined as the ratio of the area of newly formed vessels to the total area of the CAM, where VA represents the area of newly formed vessels, and CAM represents the total area of the CAM.
2.13
In vivo implantation of BSF scaffolds
The animal experiments were conducted in accordance with the Management Ordinance for Experimental Animals of China ((2001) No. 545) and the Jiangsu Province experimental animal management rules ((2008) No. 26). Forty-five Sprague-Dawley rats (180–200 g, SPF grade, male) were divided randomly into three groups and used as graft recipients of BSF scaffolds without complexes or BSF scaffolds loaded with PEI/pDNA (10/2) complexes or CBSF/BSF/PEI/pDNA (20/150/10/2) complexes, under the approval of the Experimental Animal Center of Soochow University. The number of animals in each group was 15, and one piece of BSF scaffold was implanted into the dorsal full-thickness wound of each rat. The BSF scaffolds (approximately 1.1 mm in thickness) were cut into 20 mm × 20 mm pieces and sterilized by γ-ray irradiation. The surgical pattern and procedure of the in vivo implantation of BSF scaffolds are shown in Fig. 1. As described previously,39,40 animals were kept under general anesthesia during surgery through the intro-abdominal injection of pentobarbital sodium (30–60 mg per kilogram body weight). After shaving and disinfection, full-thickness wounds (approximately 20 mm × 20 mm) were created on the upper back of each rat. The detached full-thickness skin samples were separated using a drum dermatome (the clearance of the drum dermatome was 0.2 mm) to obtain split-thickness skin grafts. The BSF scaffolds without complexes, scaffolds loaded with PEI/pDNA (10/2) complexes and scaffolds loaded with CBSF/BSF/PEI/pDNA (20/150/10/2) complexes were implanted separately into the defect sites as dermal regeneration scaffolds, and then covered with split-thickness skin grafts. The wounds were then closed with sutures, covered with Vaseline gauze and dry gauze, and fixed with circular bandages. After surgery, each animal was caged individually with free access to water and food, and the gauze was changed every other day.
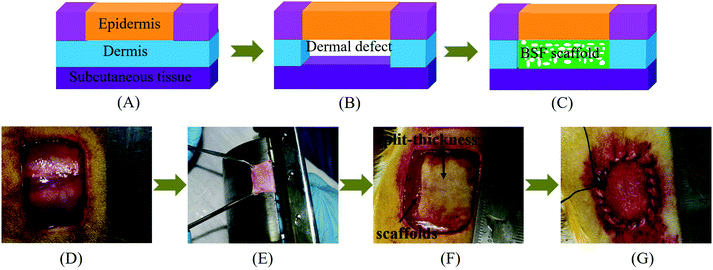 |
| Fig. 1 (A–C) Surgical pattern of the BSF scaffold implantation. (A) Normal skin, (B) skin with dermal defects, and (C) skin with implantation of BSF scaffolds. (D and E) Procedure of the in vivo implantation of BSF scaffolds. (D) Creating full thickness wounds (approximately 20 mm × 20 mm) on the upper back of a SD rat, (E) separating split-thickness skin grafts from the detached full thickness skin samples using a drum dermatome, (F) implanting BSF scaffolds into the defect sites and covering with split-thickness skin grafts, and (G) closing with sutures. | |
2.14 Histological examination
Five rats from each group were sacrificed to evaluate skin repair and histomorphology at 1, 2 and 4 weeks, respectively. After removing the Vaseline gauze and bandages, the healing state of the wound of each rat was observed and photographed, and the size of the wound was measured. The wound closure rate (%) was calculated according to eqn (2), where A0 (mm2) represents the area of original wound and At (mm2) represents the area of unhealed wound. | 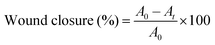 | (2) |
Specimens containing scaffolds were harvested from the wounds and immediately fixed in 4% formaldehyde in PBS at 4 °C, then subjected to multiple serial tissue sectioning and embedded in paraffin for hematoxylin–eosin (HE) and Masson staining for histological analysis. Five specimens were randomly selected from the zone of the scaffolds (not from the edge of the scaffolds) per group for measurement and analysis. The stained sections were observed using a light microscope (Olympus IX51, Japan). The histological images showed the implanted scaffold part. HE images were used to calculate the total area in the visual field S0 (mm2) and the total area of neotissue in the visual field Sn (mm2) with Image-Pro Plus 6.0 software (Media Cybernetics, Inc., Rockville, MD, USA). The tissue regeneration ratio Tr (%) was calculated using eqn (3). The density of blood vessels was calculated from HE images and normalized to the number of blood vessels per mm2. Five HE images were examined for each group at each time point.
| 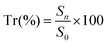 | (3) |
2.15 Statistical analysis
The results are expressed as the mean ± standard deviation. Experiments were performed at least in triplicate. The significance of differences between the mean values was calculated by ANOVA (t-test). Probability values of p < 0.05 (*) or p < 0.01 (**) were considered to be significant.
3. Results
3.1 Characterization of CBSF
The synthesis scheme of CBSF is illustrated in Fig. 2(A). The carboxyl groups of BSF were activated with EDC/NHS and then reacted with the amino groups of spermine to form amide bonds. As shown in Fig. 2(B), the spectrum of pristine BSF shows bands at 2979 and 3295 cm−1, which were assigned to the absorption of carboxyl groups.41 After the modification of BSF with spermine, the band at 2979 cm−1 disappeared, implying that the carboxyl groups were consumed in the reaction with the amino groups in spermine. The band at 3295 cm−1 shifted to 3416 cm−1, which was attributed to the new N–H or
N–H symmetrical and asymmetrical stretching vibrations, which appeared near 3351 cm−1 in the spermine spectrum.42 In contrast to the pristine BSF, the amide I absorption of CBSF at 1650 cm−1 shifted to lower wavenumbers (1635 cm−1). The absorption of amide V shifted from 692 cm−1 to 718 cm−1, suggesting that the chemical environment of the amide bonds that were formed by the reaction of the –COOH in the side chains of BSF with the –NH2 in spermine was significantly different from that of the peptide bonds in the BSF main chains.43 The significant absorption at 956 cm−1 in the spectrum of CBSF could be attributed to the O
C–N in-plane bending vibration, which was caused by the reaction between –COOH in the side chains of BSF and –NH2 in spermine.43 Thus, the FTIR spectra suggested that BSF was modified by spermine.
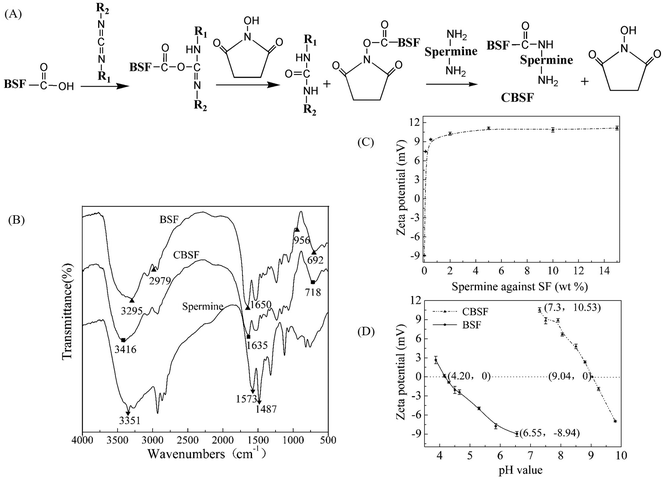 |
| Fig. 2 (A) Schematic presentation of the synthetic reaction of CBSF. (B) FTIR spectra of BSF, CBSF (modified with 5% spermine as a proportion of BSF) and spermine. (C) Zeta potential of CBSF at different weight ratios of spermine with respect to BSF. (D) Zeta potential of BSF and CBSF measured at pH values ranging from 3 to 10. | |
The zeta potential values of different ratios of spermine with respect to BSF were measured at various weight ratios ranging from 0 to 15% (Fig. 2(C)). The zeta potential of BSF before modification was approximately −9.0 mV. Using 0.1% spermine with respect to the weight of BSF changed the negative surface charge of BSF to a positive charge and converted BSF into CBSF, and the zeta potential of CBSF increased sharply to +7.5 mV. When the weight of spermine with respect to BSF was 2% or 5%, the zeta potential of CBSF increased further to +10.5 mV and +11.3 mV, respectively. As the weight of spermine with respect to BSF increased to 10% or 15%, there was no further significant increase in the zeta potential of CBSF. The zeta potentials of the pristine BSF and CBSF were measured at pH values ranging from 3 to 10 by means of the point of zero charge determined by potentiometric titration. As shown in Fig. 2(D), the isoelectric point (pI) of the pristine BSF is approximately 4.20. After modification by 5% spermine with respect to the weight of BSF, the pI of CBSF significantly increased to approximately 9.04, suggesting that the negatively charged carboxyl groups in the side chain of BSF were consumed in the amidation reaction between the amino groups in spermine and the carboxyl groups in BSF, exposing a large number of amino groups on the surface of BSF and changing the surface charge of BSF from negative to positive. These results further suggested that BSF was modified by spermine. CBSF modified with 5% spermine with respect to the weight of BSF was used in the subsequent experiments.
3.2 Characterization of CBSF/BSF/PEI/pDNA complexes
As shown in Fig. 3(A), BSF and CBSF were coated in sequence on the surface of PEI/pDNA complexes via electrostatic interactions to form CBSF/BSF/PEI/pDNA quaternary complexes. To prepare the quaternary complexes, positively charged PEI/pDNA binary complexes were first formed through electrostatic interactions between the anionic pDNA and cationic PEI molecules. The subsequent addition of negatively charged BSF made the positive charge on the surface of PEI/pDNA complexes neutralized, resulting in negatively charged BSF/PEI/pDNA ternary complexes. Finally, CBSF was added to the BSF/PEI/pDNA solution, resulting in CBSF/BSF/PEI/pDNA quaternary complexes via electrostatic interactions, hydrophobic bonds and hydrogen bonds between CBSF and the preformed ternary complexes.
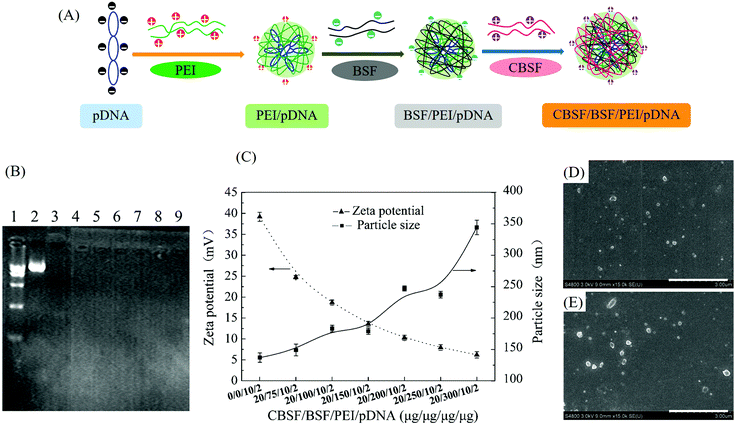 |
| Fig. 3 (A) Schematic illustration of the formation of CBSF/BSF/PEI/pDNA complexes. (B) Agarose gel electrophoresis of CBSF/BSF/PEI/pDNA complexes. Line 1: marker, lane 2: naked pDNA (control), lane 3: PEI/pDNA (10/2, control), lanes 4, 5, 6, 7, 8 and 9: CBSF/BSF/PEI/pDNA at weight ratios of 20/75/10/2, 20/100/10/2, 20/150/10/2, 20/200/10/2, 20/250/10/2 and 20/300/10/2, respectively. (C) Zeta potential and particle size of complexes at different CBSF/BSF/PEI/pDNA weight ratios. (D and E) SEM images of PEI/pDNA (10/2) and CBSF/BSF/PEI/pDNA (20/150/10/2) complexes, respectively. Scale bar: 3 μm. | |
The pDNA condensation ability of the complexes at various CBSF/BSF/PEI/pDNA weight ratios was investigated by an agarose gel retardation electrophoresis assay. As shown in Fig. 3(B), the marker and the naked pDNA displayed two distinct fluorescent bands. The mobility of the pDNA was retarded by PEI at a PEI/pDNA weight ratio of 10/2, suggesting that the pDNA could be packaged and covered completely with PEI via electrostatic interactions. After BSF and CBSF were coated in sequence on the surface of the PEI/pDNA binary complex, the quaternary complexes with different CBSF/BSF/PEI/pDNA weight ratios (20/75/10/2, 20/100/10/2, 20/150/10/2, 20/200/10/2, 20/250/10/2 and 20/300/10/2) could achieve complete retardation of pDNA, indicating that the condensation ability and stability of the PEI/pDNA complexes were not affected by coating with BSF and CBSF.
The particle sizes (hydrodynamic diameter) and zeta potentials of the complexes at various weight ratios are shown in Fig. 3(C). The PEI/pDNA complex (weight ratio 10/2) showed a high zeta potential value of approximately +40 mV and a small particle size of approximately 136 nm. Upon the addition of BSF and CBSF, the zeta potential of the quaternary complexes gradually decreased, while the particle size displayed a clear increasing trend. The zeta potential values of CBSF/BSF/PEI/pDNA complexes at weight ratios of 20/75/10/2 and 20/150/10/2 were approximately +25 mV and +14 mV, respectively, and the average particle sizes of these two complexes were approximately 150 nm and 180 nm, respectively. When the weight ratio of CBSF/BSF/PEI/pDNA exceeded 20/200/10/2, the zeta potentials of the quaternary complexes decreased to less than +10 mV, while the particle size of the complexes increased to greater than 250 nm.
The morphology of the PEI/pDNA (10/2) (Fig. 3(D)) and CBSF/BSF/PEI/pDNA (20/150/10/2) (Fig. 3(E)) complexes was observed by SEM. The micrographs showed that most complexes were uniform nanospheres approximately 100–200 nm in diameter, slightly smaller than the average sizes measured in solution.
3.3 Cytotoxicity and in vitro transfection of CBSF/BSF/PEI/pDNA complexes
The in vitro cytotoxicity of the CBSF/BSF/PEI/pDNA complexes at various weight ratios towards EA.hy926 cells was evaluated by the CCK-8 assay and compared with that of the PEI/pDNA complexes. As shown in Fig. 4(A), the PEI/pDNA complexes resulted in a cell viability of (85.1 ± 1.6)%. The CBSF/BSF/PEI/pDNA quaternary complexes resulted in a cell viability of (90.0 ± 1.0)% at a weight ratio of 20/75/10/2, which was significantly higher than that of the PEI/pDNA binary complexes (p < 0.05). When the weight ratio of CBSF/BSFPEI/pDNA was 20/100/10/2, the quaternary complexes resulted in a cell viability of (93.9 ± 1.3)%. When the weight ratio of CBSF/BSFPEI/pDNA was 20/150/10/2 or higher, the CBSF/BSFPEI/pDNA complexes exhibited lower cytotoxicity than the PEI/pDNA binary complexes, resulting in a higher cell viability of (97.1 ± 1.0)%. These results demonstrated that BSF and CBSF could significantly reduce the cytotoxicity of the PEI/pDNA complexes (p < 0.05).
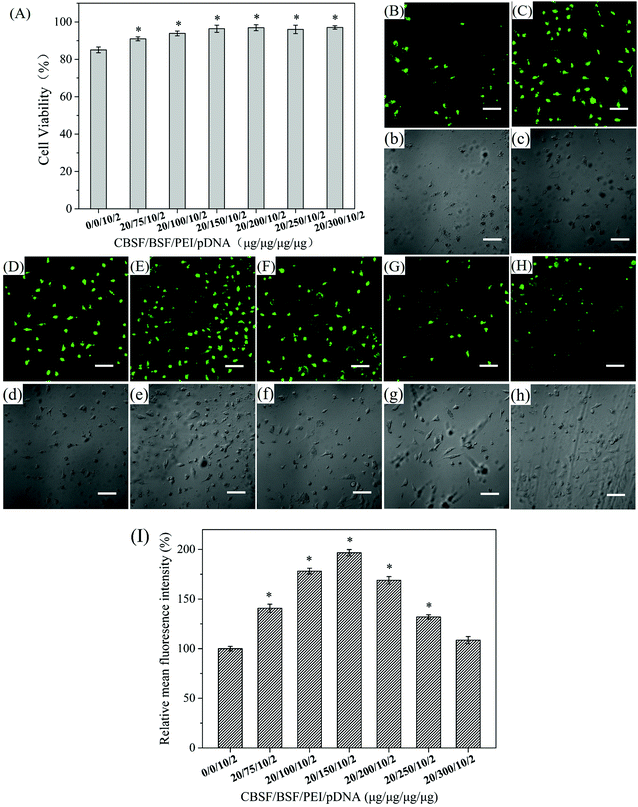 |
| Fig. 4 (A) Viability of EA.hy926 cells cocultured with CBSF/BSF/PEI/pDNA complexes at predetermined weight ratios, *p < 0.05. Confocal microscopic images of EA.hy926 cells transfected with (B and b) PEI/pDNA complexes at a weight ratio of 10/2 and with CBSF/BSF/PEI/pDNA complexes at weight ratios of (C and c) 20/75/10/2, (D and d) 20/100/10/2, (E and e) 20/150/10/2, (F, f) 20/200/10/2, (G and g) 20/250/10/2 and (H and h) 20/300/10/2 for 24 h. (B–H) Fluorescence field images and (b–h) bright field images. Scale bar: 100 μm. (I) Relative mean fluorescence intensity (MFI) of the green fluorescence images of EA.hy926 cells transfected with CBSF/BSF/PEI/pDNA complexes at predetermined weight ratios, *p < 0.05. | |
The in vitro transfection of PEI/pDNA and CBSF/BSF/PEI/pDNA complexes was assessed in EA.hy926 cells. After 24 h of transfection, green fluorescence images of the transfected EA.hy926 cells of the binary complexes and the quaternary complexes at various weight ratios were obtained by laser scanning confocal microscopy, as shown in Fig. 4(B–H and b–h). The cells transfected with the PEI/pDNA binary complexes (Fig. 4(B)) exhibited lower GFP expression levels than the cells transfected with CBSF/BSF/PEI/pDNA quaternary complexes (Fig. 4(C–F)), and the cells shrank significantly (Fig. 4(b)), suggesting that the PEI/pDNA complexes exhibited obvious cytotoxicity. The CBSF/BSF/PEI/pDNA quaternary complexes at a weight ratio of 20/75/10/2 or 20/100/10/2 (Fig. 4(C and D)) resulted in more fluorescent cells than the PEI/pDNA complexes (Fig. 4(B)), but the cells exhibited a contracted shape (Fig. 4(c and d)). The number of fluorescent cells increased with increasing CBSF/BSF/PEI/pDNA weight ratio, and the maximum level of GFP expression was observed at a weight ratio of 20/150/10/2 (Fig. 4(E)). The morphology of the transfected cells at this weight ratio changed to spreading, with polygonal characteristics (Fig. 4(e)). The GFP expression levels decreased as the weight ratios increased further (Fig. 4(F–H)) but remained higher than the GFP expression levels resulting from the PEI/pDNA binary complexes. The cells transfected with CBSF/BSF/PEI/pDNA at a weight ratio of 20/200/10/2, 20/250/10/2 or 20/300/10/2 exhibited a fully extended shape (Fig. 4(f–h)). The above results suggested that the coating of CBSF and BSF on the surface of PEI/pDNA complexes significantly reduced the cytotoxicity of the PEI/pDNA complexes and enhanced the delivery of pDNA into EA.hy926 cells, thereby increasing the expression of GFP.
To further compare the transfection efficiency, relative MFI of the green fluorescence images of EA.hy926 cells transfected with PEI/pDNA complexes and with CBSF/BSF/PEI/pDNA complexes was quantitatively calculated through the Image J software. As shown in Fig. 4(I), the CBSF/BSF/PEI/pDNA complexes resulted in a relative MFI of approximately 140% at a weight ratio of 20/75/10/2, which was significantly higher than that of the PEI/pDNA binary complexes (p < 0.05). The relative MFI increased with increasing CBSF/BSF/PEI/pDNA weight ratio, and the maximum relative MFI was approximately 196% at a weight ratio of 20/150/10/2. The relative MFI decreased as the weight ratios increased further but remained higher than that of the PEI/pDNA binary complexes.
3.4 SEM images of the cells on the BSF scaffolds
The morphologies of the cells cultured on the three groups of BSF scaffolds for 48 h are shown in Fig. 5(A1–C1 and A2–C2). The cells on the BSF scaffolds without complexes adhered tightly to the surface of the BSF scaffolds (arrows, Fig. 5(A1 andA2)). However, the transfected cells on the BSF scaffolds loaded with PEI/pDNA complexes not only aggregated significantly but also shrank markedly (arrows, Fig. 5(B1 and B2)). The transfected cells on the scaffolds loaded with CBSF/BSF/PEI/pDNA complexes exhibited full spreading on the surface of the scaffolds through protruding lamellipodia (arrows, Fig. 5(C1 and C2)). These results suggested that coating the surface of PEI/pDNA binary complexes with CBSF and BSF could effectively reduce the cytotoxicity of the PEI/pDNA complexes.
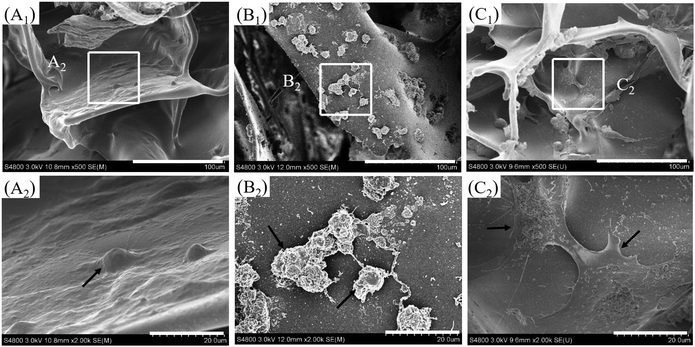 |
| Fig. 5 SEM images of EA.hy926 cells cultured for 48 h on BSF scaffolds: (A1 and A2) without complexes; (B1 and B2) loaded with PEI/pDNA (10/2); and (C1 and C2) loaded with CBSF/BSF/PEI/pDNA (20/150/10/2). Arrows indicate cells. Scale bars: (A1–C1) 100 μm, (A2–C2) 20 μm. | |
3.5 CAM assay
The CAM assay is considered an effective experimental model for the investigation of the phenomenon and mechanism of angiogenesis of vascular endothelial cells. In this study, PEI/pDNA and CBSF/BSF/PEI/pDNA complexes were incorporated into porous BSF scaffolds to investigate the influence of carrier/VEGF165–Ang-1 coexpression pDNA complexes on angiogenesis in the CAM. As shown in Fig. 6(A), the scaffolds without complexes contained a small number of tertiary blood vessels. Several tertiary and secondary blood vessels were observed on the scaffolds loaded with PEI/pDNA complexes (Fig. 6(B)). Interestingly, the blood vessels formed on the scaffolds loaded with CBSF/BSF/PEI/pDNA complexes were arranged in an organized manner with regular branching of the larger primary blood vessels into secondary blood vessels and tertiary blood vessels (Fig. 6(C)). Quantitative analysis revealed that the neovascularization ratio of the scaffolds loaded with CBSF/BSF/PEI/pDNA complexes (40.0 ± 8.5)% was twice as high as that of the scaffolds loaded with PEI/pDNA complexes (20.8 ± 2.5)% (Fig. 6(D)). The macroscopic evaluation and semiquantitative analysis of the neovascularization ratio of the CAM assay showed that the scaffolds loaded with CBSF/BSF/PEI/pDNA complexes resulted in more intense angiogenesis than the scaffolds without complexes or the scaffolds loaded with PEI/pDNA complexes, suggesting that the CBSF/BSF/PEI/pDNA quaternary complexes could transfect vascular endothelial cells on the CAM and express VEGF165 and Ang-1 to promote angiogenesis more effectively than the PEI/pDNA binary complexes (P < 0.01).
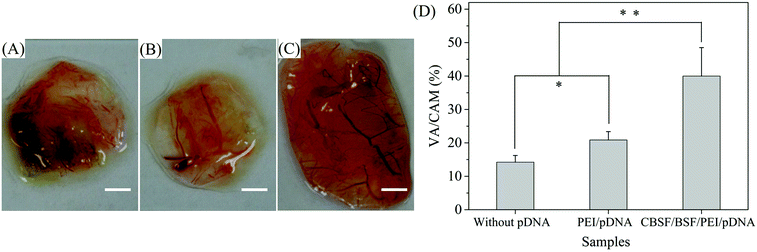 |
| Fig. 6 Digital images of the CAM after covering with BSF scaffolds for 4 days: (A) without complexes; (B) loaded with PEI/pDNA (10/2) complexes; and (C) loaded with CBSF/BSF/PEI/pDNA (20/150/10/2) complexes. Scale bar: 5 mm. (D) Quantification of the neovascularization ratio in the CAM assay. Values are represented as the mean ± SD; *P < 0.05 and **P < 0.01. | |
3.6
In vivo dermal regeneration
Vital signs and animal behavior were normal at each time point. Images from different time points after the transplantation of BSF scaffolds are shown in Fig. 7, and no empyema or infection between grafted scaffolds and the surrounding tissue was observed for any of the SD rats (Fig. 7(A)). The wound closure in all BSF scaffolds at different time points is shown in Fig. 7(B). At 1 week, the area of the closed wound of all three scaffolds exhibited no obvious difference. After two weeks of implantation, the area of the closed wound was significantly larger in the scaffolds loaded with CBSF/BSF/PEI/pDNA quaternary complexes (about 85%) than in the scaffolds without complexes (about 48%) or loaded with PEI/pDNA complexes (about 56%) (p < 0.05). At 4 weeks, unhealed wound was still observed in the scaffolds without complexes or loaded with PEI/pDNA complexes, whereas the wound had disappeared and healed completely in the scaffolds loaded with CBSF/BSF/PEI/pDNA complexes, suggesting that the scaffolds loaded with quaternary complexes could significantly accelerate wound healing.
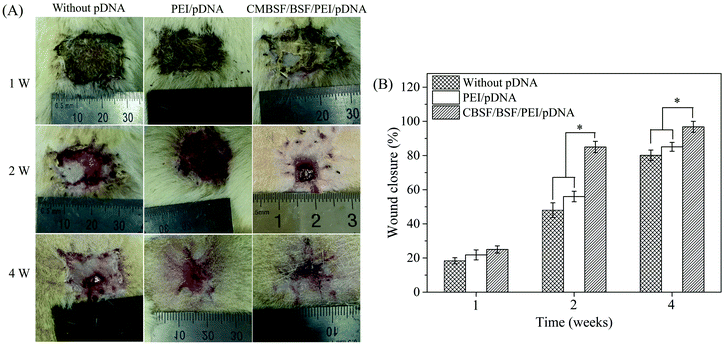 |
| Fig. 7 (A) Macroscopic observations of skin wounds after transplantation of BSF scaffolds without pDNA, loaded with PEI/pDNA (10/2) complexes and loaded with CBSF/BSF/PEI/pDNA (20/150/10/2) complexes for 1, 2 and 4 weeks. (B) Quantitative evaluation of wound closure. Values are represented as the mean ± SD; *P < 0.05. | |
Histological analyses of dermal regeneration with HE staining are shown in Fig. 8(A). Some repair cells had migrated into the scaffolds, mild inflammation reactions were observed, and newly regenerated dermal tissue appeared at the marginal region of the wounds at the first week postimplantation. A small number of vascular-like structures appeared in all groups, and the blood vessels had diameters of 10–20 μm in the first week. After two weeks, the number of repair cells and newly regenerated tissue in the scaffolds continued to increase, the neotissue and scaffolds were fully fused, and no cysts, fibrosis or inflammatory reactions were observed. The distribution of regenerated tissue was clearly more uniform in the scaffolds loaded with CBSF/BSF/PEI/pDNA quaternary complexes. Moreover, blood vessels were observed in the defect sites. At the fourth week, the residual fragments of scaffolds after degradation in vivo had been surrounded by a large amount of neotissue. The numbers of matured capillaries and the amount of newly regenerated tissue in the scaffolds loaded with CBSF/BSF/PEI/pDNA quaternary complexes were significantly higher than in the scaffolds without complexes or loaded with PEI/pDNA complexes. The numbers of newly formed blood vessels in the three groups of scaffolds are summarized quantitatively in Fig. 8(B). At 1 week, the blood vessel densities in the BSF scaffolds loaded with PEI/pDNA and the scaffolds loaded with CBSF/BSF/PEI/pDNA complexes were (41.8 ± 3.5) and (51.5 ± 4.4) per mm2, respectively, which were significantly higher than in the BSF scaffolds without complexes (p < 0.05). After 2 weeks of implantation, the vessel density in the scaffolds loaded with CBSF/BSF/PEI/pDNA complexes increased to (68.6 ± 3.8) per mm2, which was significantly higher than in the scaffolds without complexes or loaded with PEI/pDNA complexes (p < 0.05). The blood vessel densities in all groups decreased at the fourth week postimplantation. The tissue regeneration ratios in all BSF scaffolds at different time points are shown in Fig. 8(C). At 1 week, the tissue regeneration ratios of all three scaffolds exhibited no obvious difference. The tissue regeneration ratios at 2 and 4 weeks in the scaffolds loaded with CBSF/BSF/PEI/pDNA quaternary complexes were (82.6 ± 4.3)% and (92.1 ± 3.1)%, respectively, which were significantly higher than the corresponding values in the scaffolds without complexes or the scaffolds loaded with PEI/pDNA complexes (p < 0.05). These results indicated that the scaffolds loaded with CBSF/BSF/PEI/pDNA complexes had a remarkable ability to promote vascularization, neotissue formation and wound healing.
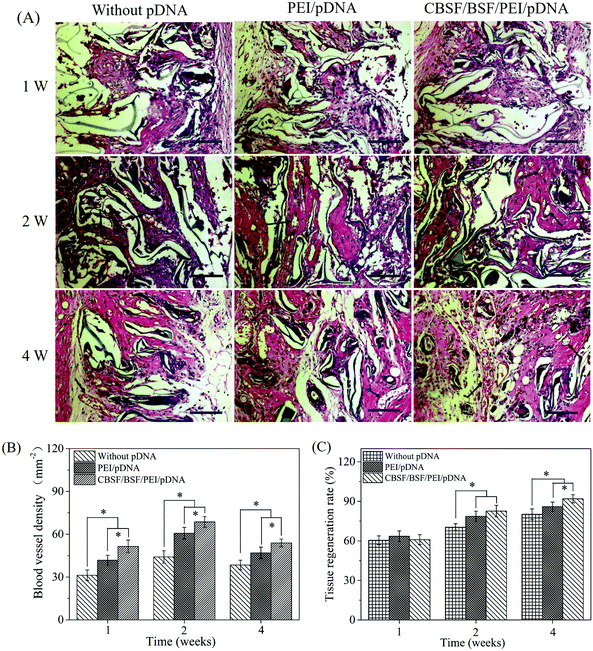 |
| Fig. 8 (A) HE images of BSF scaffolds without pDNA, loaded with PEI/pDNA (10/2) complexes and loaded with CBSF/BSF/PEI/pDNA (20/150/10/2) complexes after transplantation for 1, 2 and 4 weeks. Black arrows indicate blood vessels. Scale bar = 100 μm. (B) Blood vessel density within BSF scaffolds after implantation. (C) Tissue regeneration ratio in BSF scaffolds. *p < 0.05. | |
The deposition of collagen at different time points was revealed by Masson's staining and is shown in Fig. 9. At the first week, only a small amount of collagen deposition was detected in all groups. With the regeneration of microvascular networks and the proliferation of fibroblasts in the BSF scaffolds, more collagen was deposited in the defect sites after two weeks. The deposition of collagen in the scaffolds loaded with CBSF/BSF/PEI/pDNA quaternary complexes was higher and more regular than in the scaffolds without complexes or loaded with PEI/pDNA binary complexes. After 4 weeks, more collagen was deposited in the defect sites, and the alignment of collagen in the CBSF/BSF/PEI/pDNA group was more regular than in the other two groups, demonstrating that the scaffolds loaded with CBSF/BSF/PEI/pDNA complexes could significantly accelerate neotissue formation and collagen deposition in dermal tissue regeneration.
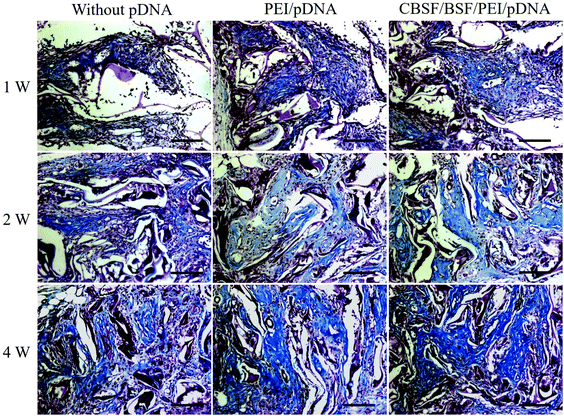 |
| Fig. 9 Masson staining images of BSF scaffolds without complexes, loaded with PEI/pDNA (10/2) complexes and loaded with CBSF/BSF/PEI/pDNA (20/150/10/2) complexes after transplantation for 1, 2 and 4 weeks. Scale = 100 μm. | |
4. Discussion
Dermal regeneration scaffolds are highly important in treating full-thickness skin defects.27,44 However, the major limitation of dermal regeneration scaffolds for the treatment of wounds is their slow vascularization,45 which may result in graft necrosis. Sufficient vascularization of the implanted dermal scaffolds plays a crucial role in determining the survival and function of the skin graft. Angiogenesis, the process of new blood vessel growth, is a basic process of vascularization and is extensively enhanced by various angiogenic growth factors such as bFGF, VEGF, Ang-1 and PDGF,46–48 among which VEGF and Ang-1 are the most important growth factors that regulate blood vessel formation, acting on specific vascular endothelial cell surface receptors (Flk-1/Flt-1 and Tie2, respectively).49 Ang-1 and VEGF play complementary roles during vascular development. VEGF acts early during vessel formation, whereas Ang-1 acts later during vessel remodeling, maturation and stabilization.50 Despite the vast interest in growth factors and their potential for wound healing, the direct application of exogenous growth factors has limited success due to their sensitivity and instability, since their active half-lives are only on the order of minutes in serum.12 By contrast, biomaterial scaffolds loaded with genes encoding angiogenic growth factors can avoid the disadvantages of exogenous growth factors with short half-lives and low stability. Thus, the combination of dermal regeneration scaffolds with the delivery of genes encoding angiogenic growth factors would be an appealing approach to provide sustained bioactive angiogenic growth factors via transfected cells to promote angiogenesis.51,52
This work is the first report of the synthesis of CBSF and the use of Bombyx mori silk fibroin as a gene delivery carrier. Gene transfection efficiency is greatly dependent on the gene delivery carrier. An ideal carrier must possess a number of key qualities including efficient transfection with minimal associated cytotoxicity and safety concerns. Among cationic gene carriers, PEI has attracted significant interest because of its excellent transfection ability both in vitro and in vivo.53,54 However, high-molecular-weight PEI is reported to be cytotoxic due to its high cationic charge density.55 To overcome the disadvantages of PEI, in this study, BSF and CBSF were coated in sequence on the surface of PEI/pDNA binary complex to shield the excess positive charge on the PEI/pDNA complex surface, resulting in CBSF/BSF/PEI/pDNA quaternary complex. To synthesize CBSF, BSF was modified with spermine with the mediation of EDC. Spermine is a polyamine that has two primary and two secondary amino groups. EDC was coupled with the carboxyl groups in the aspartic and glutamic side chains of BSF and formed unstable intermediate urea derivatives (O-acylisourea), which were converted into amine-reactive sulfo-NHS esters in the presence of NHS. Then, the O-acylisourea reacted with the primary amine groups in spermine to form stable amide bonds between BSF and spermine, resulting in CBSF (Fig. 2(A)). Both the FTIR spectra and the measurement of zeta potential indicated that spermine was covalently grafted onto BSF through the amidation reaction (Fig. 2(B and C)), changing the surface charge of BSF completely from negative to positive. As the weight ratio of spermine with respect to BSF increased from 0.1% to 2%, the zeta potential value presented a nearly linear upward tendency, probably due to the consumption of –COOH groups and the introduction of –NH2 groups into BSF. When the weight ratio of spermine was 5% or more, the zeta potential value was stable at approximately +11 mV because the carboxyl groups on the BSF were nearly consumed in the reaction with spermine, resulting in the maximal grafting of spermine. The pI of a protein is mainly determined by its number of amino groups and carboxyl groups. The zeta potentials of BSF and CBSF at pH values ranging from 3 to 10 were measured (Fig. 2(D)). The pI of BSF increased significantly from 4.2 to 9.0 after modification with spermine. These results further indicated that spermine was successfully grafted onto the side chains of BSF.
Cationic agents with high charge density have been shown to induce membrane toxicity due to their electrostatic interactions with the negatively charged glycocalyx of the cellular surface.56 Electrostatic polymer coating has recently been proposed as a promising strategy to overcome the aforementioned limitations of PEI/pDNA complexes. Various anionic polymers such as poly(acrylic acid), poly(γ-glutamic acid) and carboxylated poly(ethylene glycol) derivatives have been electrostatically deposited on cationic PEI/pDNA complexes to effectively reduce their cytotoxicity and agglutination by shielding their positive surface charges.57–59 In this study, PEI with a high positive charge was used to condense pDNA due to its “proton sponge” effect, which allowed the release of pDNA into the cytoplasm. The PEI/pDNA complex exhibited a high zeta potential of approximately +40 mV (Fig. 3(C)). It is possible to get complexes with the same surface positive charge as the CBSF/BSF/PEI/pDNA quaternary complexes if the surface of PEI/pDNA binary complexes was only coated with low concentration of BSF. However, we wanted to know whether the carrier/pDNA complexes could still transfect cells when the outermost layer of the complexes was Bombyx mori silk fibroin, which has not been reported in previous literature. Therefore, in order to weaken the high positive charge density on the PEI/pDNA complex surface and meanwhile make Bombyx mori silk fibroin exist on the outermost layer of the complexes, firstly, negatively charged BSF was coated on the surface of the preformed PEI/pDNA binary complexes via electrostatic interactions, which reversed the surface charge from positive to negative (Fig. 3(A)). CBSF was subsequently adsorbed on the BSF/PEI/pDNA complex surface to form CBSF/BSF/PEI/pDNA quaternary complexes, again reversing the surface charge. The coating of CBSF on the BSF/PEI/pDNA complex surface enhanced the electrostatic interaction between the ternary complexes and negatively charged cell membranes, thereby improving the transfection efficiency. After 24 h of transfection, the EA.hy926 cells transfected with PEI/pDNA binary complexes exhibited lower GFP expression levels than the cells transfected with CBSF/BSF/PEI/pDNA quaternary complexes (Fig. 4(B–H)), and the cells transfected with PEI/pDNA complexes were significantly shrunken (Fig. 4(b)). As the CBSF/BSF/PEI/pDNA weight ratio increased, the expression of GFP improved (Fig. 4(C–E)). The maximum expression level of GFP was observed at a weight ratio of 20/150/10/2 (Fig. 4(E)), and these transfected cells were fully extended, with polygonal characteristics (Fig. 4(e)). The SEM images of EA.hy926 cells cultured on the PEI/pDNA or CBSF/BSF/PEI/pDNA loaded scaffolds (Fig. 5(A1–C1 and A2–C2)) further showed that coating BSF on the surface of PEI/pDNA binary complexes could effectively shield the excess positive charge of PEI to reduce the cytotoxicity of the PEI/pDNA complexes (Fig. 4(A)). Meanwhile, coating CBSF on the surface of the BSF/PEI/pDNA ternary complexes endowed the surface of CBSF/BSF/PEI/pDNA complexes with an appropriate positive charge, resulting in a higher transfection efficiency. As the weight ratio of CBSF/BSF/PEI/pDNA increased, the zeta potential of the quaternary complexes showed a decreasing trend, which weakened the electrostatic interactions between the complexes and cell membranes, thereby decreasing the density of fluorescent cells (Fig. 4(F–H)). The quantitative measurements of relative MFI of the green fluorescence images further suggested that the coating of BSF and CBSF on the PEI/pDNA complex surface could effectively improve the transfection efficiency (Fig. 4(I)).
The CAM assay proved to be an efficient model to assess the efficiency of scaffolds in inducing neovascularization.60 The angiogenic activities of scaffolds loaded with carrier/pDNA complexes were investigated in the CAM assay. As illustrated in Fig. 6(A–C), a significantly higher number of highly branched neovessels were observed in the CBSF/BSF/PEI/pDNA group than in the other two groups, suggesting that the cells in the CAM were successfully transfected with CBSF/BSF/PEI/pDNA complexes, leading to higher expression levels of VEGF and Ang-1 inside the scaffolds and thereby accelerating the process of angiogenesis (Fig. 6(D)). The results showed that quaternary complex-loaded scaffolds exhibited the fastest vascularization rate when compared with the scaffolds without complexes and the binary complex-loaded scaffolds.
To investigate whether the BSF scaffolds loaded with carrier/pDNA complexes could effectively improve vascularization, thereby promoting the regeneration of dermal tissue, BSF scaffolds loaded with carrier/pDNA complexes were also implanted into defect sites in Sprague-Dawley rats as dermal regeneration scaffolds. At 2 weeks, the area of the closed wound in the scaffolds loaded with CBSF/BSF/PEI/pDNA quaternary complexes was approximately 85%, which was significantly larger than in the scaffolds without complexes or loaded with PEI/pDNA complexes (p < 0.05) (Fig. 7(A)). After implantation for four weeks, the wound had disappeared and healed completely in the scaffolds loaded with CBSF/BSF/PEI/pDNA complexes (Fig. 7(B)), suggesting the acceleration of wound healing. The neovascularization processes and neotissue formation were monitored by HE and Masson staining at different postsurgery time points. At the first week of skin repair, some amount of newly regenerated dermal tissue appeared at the margins of the wounds (Fig. 8(A)), and the scaffolds loaded with CBSF/BSF/PEI/pDNA quaternary complexes showed a blood vessel density of (51.5 ± 4.4) per mm2, which was significantly higher than that in the scaffolds without complexes and in the scaffolds loaded with PEI/pDNA binary complexes (p < 0.05) (Fig. 8(B)). After two weeks, the distribution of regenerated tissue and collagen was clearly more uniform in the scaffolds loaded with CBSF/BSF/PEI/pDNA quaternary complexes than in the scaffolds without complexes or loaded with PEI/pDNA binary complexes (Fig. 9). The vessel density in the scaffolds loaded with CBSF/BSF/PEI/pDNA complexes increased to (68.6 ± 3.8) per mm2, which was significantly higher than in the scaffolds without complexes or loaded with PEI/pDNA complexes. The blood vessel density in all three kinds of scaffolds decreased after four weeks of implantation. The tissue regeneration ratio in the scaffolds loaded with CBSF/BSF/PEI/pDNA quaternary complexes was (92.0 ± 3.1)%, which was significantly higher than that in the scaffolds without complexes and in the scaffolds loaded with PEI/pDNA complexes (Fig. 8(C)). Further deposition of collagens was observed in the defect sites. Compared to the observation in scaffolds without complexes or loaded with PEI/pDNA complexes, the alignment of collagens in the scaffolds loaded with CBSF/BSF/PEI/pDNA complexes was more regular and more similar to that in normal dermal tissue. The above results suggested that when the CBSF/BSF/PEI/pDNA complexes were incorporated into the BSF scaffolds, the carrier/VEGF165–Ang-1 coexpression pDNA complexes could transfect the host cells in situ and effectively stimulate the formation of capillary and microvascular networks in the scaffolds, supplying sufficient oxygen and nutrients for repair cells and thereby promoting the migration and proliferation of fibroblasts that infiltrated the scaffolds. Furthermore, the fibroblasts in the scaffolds can effectively secrete an extracellular matrix, resulting in the deposition of collagen in the scaffolds and thereby facilitating the regeneration of dermal tissue. Therefore, scaffolds loaded with the CBSF/BSF/PEI/pDNA quaternary complexes could effectively promote vascularization and the regeneration of dermal tissue, offering potential for dermal tissue reconstruction.
5. Conclusions
In this study, BSF was successfully modified with spermine through an amidation reaction between the amino groups in the spermine and the carboxyl groups in the side chain of BSF, using carbodiimide as an activator, and the surface charge of BSF was thereby converted from negative to positive. BSF and CBSF were successively coated on the surface of PEI/pDNA binary complexes to form CBSF/BSF/PEI/pDNA quaternary complexes. The successive coating of BSF and CBSF not only effectively shielded the excess positive charge on the PEI/pDNA complex surface but also endowed the CBSF/BSF/PEI/pDNA complexes with an appropriate positive charge. The results of coculturing EA.hy926 cells with the carrier/pDNA complexes indicated that the CBSF/BSF/PEI/pDNA quaternary complexes exhibited lower cytotoxicity and higher transfection efficiency than the PEI/pDNA complexes. The CAM assay showed that scaffolds loaded with the quaternary complexes had denser blood vessel networks than the scaffolds without complexes or the scaffolds loaded with PEI/pDNA binary complexes. The in vivo experimental results of implanting BSF scaffolds into dorsal full-thickness wounds in Sprague-Dawley rats as dermal regeneration scaffolds suggested that the process of angiogenesis was significantly accelerated by CBSF/BSF/PEI/pDNA loaded scaffolds, leading to faster collagen deposition and regeneration of the dermal tissue. These results revealed that the scaffolds loaded with CBSF/BSF/PEI/pDNA quaternary complexes provided a promising approach to promoting the reconstruction of dermal tissue.
Conflicts of interest
There are no conflicts to declare.
Acknowledgements
This work was supported by the National Key Research and Development Program of China (Project No. 2017YFC1103602), the National Natural Science Foundation of China (31370968), the Applied Basic Research Program of Nantong City (GY 12016047), and the Jiangsu Province Ordinary Universities and Colleges Graduate Scientific and Innovation Plan (KYZZ16_0087).
References
- F. S. Frueh, M. D. Menger, N. Lindenblatt, P. Giovanoli and M. W. Laschke, Crit. Rev. Biotechnol., 2017, 37, 613–625 CrossRef CAS PubMed.
- J. Xiang, J. Li, J. He, X. Tang, C. Dou, Z. Cao, B. Yu, C. Zhao, F. Kang, L. Yang, S. Dong and X. Yang, ACS Appl. Mater. Interfaces, 2016, 8, 4489–4499 CrossRef CAS PubMed.
- E. C. Novosel, C. Kleinhans and P. J. Kluger, Adv. Drug Delivery Rev., 2011, 63, 300–311 CrossRef CAS PubMed.
- L. Dew, S. MacNeil and C. K. Chong, Regener. Med., 2015, 10, 211–224 CrossRef CAS PubMed.
- Q. Wang, Y. Zhang, B. Li and L. Chen, J. Mater. Chem. B, 2017, 5, 6963–6972 RSC.
- X. Wu, M. Bruschi, T. Waag, S. Schweeberg, Y. Tian, T. Meinhardt, R. Stigler, K. Larsson, M. Funk, D. Steinmüller-Nethl, M. Rasse and A. Kruege, J. Mater. Chem. B, 2017, 5, 6629–6636 RSC.
- R. Murali, T. Ponrasu, K. Cheirmadurai and P. Thanikaivelan, J. Biomed. Mater. Res., Part A, 2016, 104, 388–396 CrossRef CAS PubMed.
- H. Ferreira, A. Martins, M. L. A. da Silva, S. Amorim, S. Faria, R. A. Pires, R. L. Reis and N. M. Neves, J. Mater. Chem. B, 2018, 6, 2104–2115 RSC.
- J. Chen, Y. Li, B. Wang, J. Yang, B. C. Heng, Z. Yang, Z. Ge and J. Lin, J. Mater. Chem. B, 2018, 6, 675–687 RSC.
- L. L. Y. Chiu and M. Radisic, Biomaterials, 2010, 31, 226–241 CrossRef CAS PubMed.
- J. Gavard, V. Patel and J. S. Gutkind, Dev. Cell, 2008, 14, 25–36 CrossRef CAS PubMed.
- H. L. Xu, W. Z. Yu, C. T. Lu, X. K. Li and Y. Z. Zhao, Biotechnol. J., 2017, 12, 1600243 CrossRef PubMed.
- R. Guo, S. Xu, L. Ma, A. Huang and C. Gao, Biomaterials, 2011, 32, 1019–1031 CrossRef CAS PubMed.
- Y. Han, R. Tao, Y. Han, T. Sun, J. Chai, G. Xu and J. Liu, Cytotherapy, 2014, 16, 160–169 CrossRef CAS.
- H. N. Yang, J. H. Choi, J. S. Park, S. Y. Jeon, K. D. Park and K. H. Park, Biomaterials, 2014, 35, 4716–4728 CrossRef CAS PubMed.
- S. R. Choudhury, E. Hudry, C. A. Maguire, M. Sena-Esteves, X. O. Breakefield and P. Grandi, Neuropharmacology, 2017, 120, 63–80 CrossRef CAS PubMed.
- M. Foldvari, D. W. Chen, N. Nafissi, D. Calderon, L. Narsineni and A. Rafiee, J. Controlled Release, 2016, 240, 165–190 CrossRef CAS.
- S. O’Rorke, M. Keeney and A. Pandit, Prog. Polym. Sci., 2010, 35, 441–458 CrossRef.
- H. Yin, R. L. Kanasty, A. A. Eltoukhy, A. J. Vegas, J. R. Dorkin and D. G. Anderson, Nat. Rev. Genet., 2014, 15, 541–555 CrossRef CAS.
- G. David, L. Clima, M. Calin, C. A. Constantinescu, M. Balan-Porcarasu, C. M. Uritu and B. C. Simionescu, Polym. Chem., 2018, 9, 1072–1081 RSC.
- A. P. Pandey and K. K. Sawant, Mater. Sci. Eng., C, 2016, 68, 904–918 CrossRef CAS.
- G. G. Lazarus and M. Singh, Colloids Surf., B, 2016, 145, 906–911 CrossRef CAS.
- Y. Hu, D. Zhou, C. Li, H. Zhou, J. Chen, Z. Zhang and T. Guo, Acta Biomater., 2013, 9, 5003–5012 CrossRef CAS.
- M. M. Xun, Y. P. Xiao, J. Zhang, Y. H. Liu, Q. Peng, Q. Guo, W. X. Wu, Y. Xu and X. Q. Yu, Polymer, 2015, 65, 45–54 CrossRef CAS.
- L. Parhamifar, A. K. Larsen, A. C. Hunter, T. L. Andresen and S. M. Moghimi, Soft Matter, 2010, 6, 4001–4009 RSC.
- S. K. Tripathi, S. Gupta, K. C. Gupta and P. Kumar, RSC Adv., 2013, 3, 15687–15697 RSC.
- X. Li, R. You, Z. Luo, G. Chen and M. Li, J. Mater. Chem. B, 2016, 4, 2903–2912 RSC.
- Q. Zhang, S. Yan, R. You, D. L. Kaplan, Y. Liu, J. Qu, X. Li, M. Li and X. Wang, J. Biomed. Mater. Res., Part A, 2016, 104, 3045–3057 CrossRef CAS.
- W. J. Kim, S. B. Kim, Y. D. Cho, W. J. Yoon, J. H. Baek, K. M. Woo and H. M. Ryoo, Biomaterials, 2017, 122, 154–162 CrossRef CAS.
- T. Yucel, M. L. Lovett and D. L. Kaplan, J. Controlled Release, 2014, 190, 381–397 CrossRef CAS.
- B. Mao, C. Liu, W. Zheng, X. Li, R. Ge, H. Shen, X. Guo, Q. Lian, X. Shen and C. Li, Biomaterials, 2018, 161, 306–320 CrossRef CAS.
- M. Wu, W. Yang, S. Chen, J. Yao, Z. Shao and X. Chen, J. Mater. Chem. B, 2018, 6, 1179–1186 RSC.
- C. Ma, L. Lv, Y. Liu, Y. Yu, R. You, J. Yang and M. Li, Biomed. Mater., 2014, 9, 035015 CrossRef.
- Y. Liu, R. You, G. Liu, X. Li, W. Sheng, J. Yang and M. Li, Int. J. Mol. Sci., 2014, 15, 7049–7063 CrossRef CAS.
- L. Li, S. Puhl, L. Meinel and O. Germershaus, Biomaterials, 2014, 35, 7929–7939 CrossRef CAS.
- F. Rancan, M. Asadian-Birjand, S. Dogan, C. Graf, L. Cuellar, S. Lommatzsch, U. Blume-Peytavi, M. Calderón and A. Vogt, J. Controlled Release, 2016, 228, 159–169 CrossRef CAS.
- X. D. Xu, Y. J. Cheng, J. Wu, H. Cheng, S. X. Cheng, R. X. Zhuo and X. Z. Zhang, Biomaterials, 2016, 76, 238–249 CrossRef CAS PubMed.
- S. Yan, Q. Zhang, J. Wang, Y. Liu, S. Lu, M. Li and D. L. Kaplan, Acta Biomater., 2013, 9, 6771–6782 CrossRef CAS.
- C. L. Dearth, T. J. Keane, C. A. Carruthers, J. E. Reing, L. Huleihel, C. A. Ranallo, E. W. Kollar and S. F. Badylak, Acta Biomater., 2016, 33, 78–87 CrossRef CAS.
- D. Li, G. Jiao, W. Zhang, X. Chen, R. Ning and C. Du, RSC Adv., 2016, 6, 19887–19896 RSC.
- J. Wang, Q. Yang, N. Cheng, X. Tao, Z. Zhang, X. Sun and Q. Zhang, Mater. Sci. Eng., C, 2016, 61, 705–711 CrossRef CAS.
- S. K. Gupta, M. Kumar, A. Guldhe, F. A. Ansari, I. Rawat, K. Kanney and F. Bux, Energy Convers. Manage., 2014, 85, 537–544 CrossRef CAS.
- Y. Yu, Y. Hu, X. Li, Y. Liu, M. Li, J. Yang and W. Sheng, Int. J. Nanomed., 2016, 11, 1013–1023 CAS.
- S. B. Mahjour, X. Fu, X. Yang, J. Fong, F. Sefat and H. Wang, Burns, 2015, 4, 1764–1774 CrossRef.
- F. Berthiaume, T. J. Maguire and M. L. Yarmush, Annu. Rev. Chem. Biomol. Eng., 2011, 2, 403–430 CrossRef.
- J. Rouwkema and A. Khademhosseini, Trends Biotechnol., 2016, 34, 733–745 CrossRef CAS.
- J. R. García, A. Y. Clark and A. J. García, J. Biomed. Mater. Res., Part A, 2016, 104, 889–900 CrossRef.
- R. Murali, T. Ponrasu, K. Cheirmadurai and P. Thanikaivelan, J. Biomed. Mater. Res., Part A, 2016, 104, 388–396 CrossRef CAS.
- L. Zhou, W. Ma, Z. Yang, F. Zhang, L. Lu, Z. Ding, B. Ding, X. Gao and C. Li, Gene Ther., 2005, 12, 196–202 CrossRef CAS.
- G. Thurston, J. S. Rudge, E. Ioffe, H. Zhou, L. Ross, S. D. Croll, N. Glazer, J. Holash, D. M. McDonald and G. D. Yancopoulos, Nat. Med., 2000, 6, 460–463 CrossRef CAS.
- R. M. Raftery, E. G. Tierney, C. M. Curtin and S. A. Cryan, F. J. O'brien, J. Controlled Release, 2015, 210, 84–94 CrossRef CAS.
- E. G. Tierney, G. P. Duffy, A. J. Hibbitts, S. A. Cryan and F. J. O’brien, J. Controlled Release, 2012, 158, 304–311 CrossRef CAS.
- M. D. Giron-Gonzalez, R. Salto-Gonzalez, F. J. Lopez-Jaramillo, A. Salinas-Castillo, A. B. Jodar-Reyes, M. Ortega-Muñoz, F. Hernandez-Mateo and F. Santoyo-Gonzalez, Bioconjugate Chem., 2016, 27, 549–561 CrossRef CAS.
- J. Lv, J. Yang, X. Hao, X. Ren, Y. Feng and W. Zhang, J. Mater. Chem. B, 2016, 4, 997–1008 RSC.
- D. Fischer, Y. Li, B. Ahlemeyer, J. Krieglstein and T. Kissel, Biomaterials, 2003, 24, 1121–1131 CrossRef CAS.
- S. Choksakulnimitr, S. Masuda, H. Tokuda, Y. Takakura and M. Hashida, J. Controlled Release, 1995, 34, 233–241 CrossRef CAS.
- S. K. Tripathi, Z. Ahmadi, K. C. Gupta and P. Kumar, Colloids Surf., B, 2016, 140, 117–120 CrossRef CAS PubMed.
- T. Kurosaki, T. Kitahara, S. Fumoto, K. Nishida, J. Nakamura, T. Niidome, Y. Kodama, H. Nakagawa, H. To and H. Sasaki, Biomaterials, 2009, 30, 2846–2853 CrossRef CAS PubMed.
- M. Sakae, T. Ito, C. Yoshihara, N. Iida-Tanaka, H. Yanagie, M. Eriguchi and Y. Koyama, Biomed. Pharmacother., 2008, 62, 448–453 CrossRef CAS.
- A. Magnaudeix, J. Usseglio, M. Lasgorceix, F. Lalloue, C. Damia, J. Brie, P. Pascaud-Mathieu and E. Champion, Acta Biomater., 2016, 38, 179–189 CrossRef CAS.
|
This journal is © The Royal Society of Chemistry 2019 |