DOI:
10.1039/C9TA06385D
(Paper)
J. Mater. Chem. A, 2019,
7, 19522-19530
Structural engineering of pyrrolo[3,4-f]benzotriazole-5,7(2H,6H)-dione-based polymers for non-fullerene organic solar cells with an efficiency over 12%†
Received
14th June 2019
, Accepted 25th July 2019
First published on 25th July 2019
Abstract
In this work, we have synthesized two wide band gap donor polymers based on benzo[1,2-b:4,5-b′]dithiophene (BDT) and pyrrolo[3,4-f]benzotriazole-5,7(2H,6H)-dione (TzBI), namely, PBDT-TzBI and PBDT-F-TzBI and studied their photovoltaic properties by blending them with ITIC as an acceptor. Polymer solar cell devices made from PBDT-TzBI:ITIC and PBDT-F-TzBI:ITIC exhibited power conversion efficiencies (PCEs) of 9.22% and 11.02% and while annealing at 160 °C, improved the device performances to 10.24% and 11.98%, respectively. Upon solvent annealing with diphenyl ether (DPE) (0.5%) and chlorobenzene (CB), the PCE of the PBDT-F-TzBI-based device increased to 12.12%. The introduction of the fluorinated benzodithiophene (BDT-F) moiety on the backbone of PBDT-F-TzBI improved the open circuit voltage, short circuit current and fill factor simultaneously. The high PCEs of the PBDT-F-TzBI:ITIC-based devices were supported by comparison and analysis of the optical and electronic properties, the charge carrier mobilities, exciton dissociation probabilities, and charge recombination behaviors of the devices.
Introduction
Organic solar cells (OSCs) attracted the attention of the scientific community due to the possibility of manufacturing light weight, flexible, and easily printed next generation solar cell devices using the roll-to-roll printing technique.1–6 Although PCBM derivatives were the acceptors of choice in the past, recent years have witnessed the rapid development of non-fullerene acceptors because of the flexibility of choosing different donor materials for tunable optical and energetic properties of the active layer of OSCs.1,7–15 In the last five years, non-fullerene OSCs showed impressively high PCEs exceeding 15% using fused-ring structured electron acceptors.11,16 These results motivate researchers to design new donor polymers with desirable properties, such as broad and intense absorption to enhance short-circuit current (Jsc) and suitable energy levels to improve open-circuit voltage (Voc). The donor–acceptor (D–A) alternating conjugated polymer synthesis is the commonly used strategy to prepare efficient donor polymers. In this regard, among the commonly investigated wide band-gap polymers are thieno[3,4-c]pyrrole-4,6-dione17–19 (TPD)-, [2,1,3]benzothiadiazole20–27 (BT)- and 2H-benzo[d][1,2,3]triazole8,28–31 (BTz)-based D–A conjugated polymers. Yin et al.32 combined TPD and BT to garner the advantages of solubility and deep HOMO energy levels simultaneously, and synthesized the [2,1,3]benzothiadiazole-5,6-dicarboxylic imide-based acceptor monomer 4,8-bis(5-bromothiophen-2-yl)-6-(2-ethylhexyl)-5H-[1,2,5]thiadiazolo[3,4-f]isoindole-5,7(6H)-dione. By copolymerizing this compound with (4,8-bis(5-(2-butyloctyl)thiophen-2-yl)benzo[1,2-b:4,5-b′]dithiophene-2,6-diyl)bis(trimethylstannane), a polymer was obtained which was blended with PC71BM to fabricate solar cells that afforded a PCE of 5.19% with Voc of 0.91 V, Jsc of 11.59 mA cm−2 and FF of 0.49. Later on McCulloch et al.33 synthesized other [2,1,3]benzothiadiazole-5,6-dicarboxylic imide-based polymers and reported a high PCE of 8.3% with a Voc of 0.80 V, Jsc of 16.45 mA cm−2 and FF of 0.63.
Cao et al.34 modified the [2,1,3]benzothiadiazole-5,6-dicarboxylic imide moiety by replacing the BT unit with BTz to prepare the acceptor monomer 4,8-bis(5-bromothiophen-2-yl)-2,6-dioctyl-[1,2,3]triazolo[4,5-f]isoindole-5,7(2H,6H)-dione (Br2-TzBI), which was envisaged to impart lower energy levels on the resulting D–A polymers with improved solubility. By copolymerizing Br2-TzBI with the weak electron donor monomer, (4,8-bis(5-(2-ethylhexyl)thiophen-2-yl)benzo[1,2-b:4,5-b′]dithiophene-2,6-diyl)bis(trimethylstannane) (Sn2-BDT), PTzBIBDT was obtained, which afforded a high PCE of 8.63% with a Voc of 0.87 V, Jsc of 13.50 mA cm−2 and FF of 0.74 in solar cell devices with PC71BM as an acceptor.34 Furthermore, by blending PTzBIBDT with ITIC, the Jsc was enhanced to 18.29 mA cm−2 and a high PCE of 10.24% with a Voc of 0.87 V and FF of 0.64 was demonstrated.35 Cao et al.36 further replaced the 5-(2-ethylhexyl)thiophene units attached to the BDT moiety of PTzBIBDT with 4-(2-ethylhexyl)phenyl units and synthesized PTzBI-Ph. The solar cells fabricated from the blend of PTzBI-Ph with ITIC gave improved Voc and FF values of 0.92 V and 0.68, respectively. However, the Jsc of the device dropped to 16.39 mA cm−2 to give a PCE of 10.19%.36 In addition, Cao et al.37 prepared different kinds of TzBI-based polymers by incorporating alkyl side groups and studied their photovoltaic performances with ITIC as an acceptor and registered a decent PCE of 7.28%. When the acceptor was changed to IT-2F, the performances of these devices were improved and a certified PCE of 12.25% with enhanced Jsc and FF could be achieved (ESI, Table S1†). These results show that enhancing Voc, Jsc and FF simultaneously is still challenging for solar cells fabricated from TzBI-based polymer donors using ITIC as an acceptor. Clearly, there is room for further improvement in the performances of solar cells made of TzBI-based polymers with some structural modifications. It is known that introduction of fluorine atoms in the polymer structure makes the HOMO and LUMO energy levels of the polymer deeper, thereby enhancing Voc.38 It has also been shown that enhanced inter/intramolecular interactions of the polymer can improve crystallinity and facilitate charge transport which results in improved Jsc and FF values.38–41 Taking these advantages into consideration, we decided to use a fluorine-containing BDT-based monomer 3 (Scheme 1) as an electron donor and polymerized it with TzBI-based monomer 1 to prepare PBDT-F-TzBI which was expected to enhance Voc, Jsc and FF simultaneously, in non-fullerene OSCs. Thus, solar cells fabricated from the PBDT-F-TzBI:ITIC blend afforded a high PCE of 12.12% with a Voc of 0.93 V, Jsc of 18.10 mA cm−2 and FF of 0.72, which is higher than those of the corresponding non-fluorinated PBDT-TzBI:ITIC-based solar cell devices. To investigate the reasons behind the variations in performances of the solar cells, we have studied the optical and electronic properties, the morphology, charge carrier mobility, exciton dissociation and charge recombination behaviors of the corresponding devices.
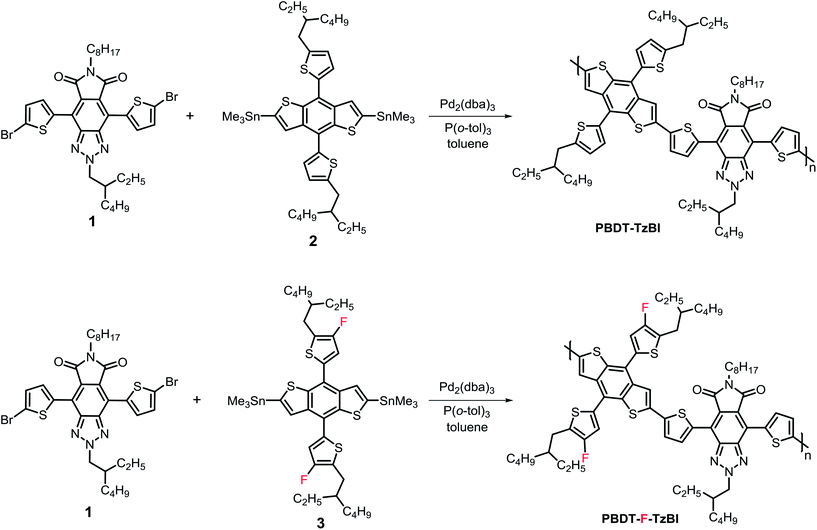 |
| Scheme 1 Synthesis of PBDT-TzBI and PBDT-F-TzBI. | |
Results and discussion
Synthesis of monomers and polymers
The chemical structures of the polymers are shown in Scheme 1. The TzBI-based acceptor monomer, 4,8-bis(5-bromothiophen-2-yl)-2-(2-ethylhexyl)-6-octyl-[1,2,3]triazolo[4,5-f]isoindole-5,7(2H,6H)-dione (1) was synthesized by modifying procedures reported in the literature (Scheme S1, ESI†).34 In this work, we have introduced a branched side chain on the benzotriazole unit of 1 to enhance the solubility of the polymer. Compound 1 was copolymerized with the BDT-based monomers (4,8-bis(5-(2-ethylhexyl)thiophen-2-yl)benzo[1,2-b:4,5-b′]dithiophene-2,6-diyl)bis(trimethylstannane) (2) and (4,8-bis(5-(2-ethylhexyl)-4-fluorothiophen-2-yl)benzo[1,2-b:4,5-b′]dithiophene-2,6-diyl)bis(trimethylstannane) (3) at 110 °C in toluene by means of the Stille coupling polymerization reaction, to yield poly-4-(5-(4,8-bis(5-(2-ethylhexyl)thiophen-2-yl)-6-methylbenzo[1,2-b:4,5-b′]dithiophen-2-yl)thiophen-2-yl)-2-(2-ethylhexyl)-8-(5-methylthiophen-2-yl)-6-octyl-[1,2,3]triazolo[4,5-f]isoindole-5,7(2H,6H)dione (PBDT-TzBI) and poly-4-(5-(4,8-bis(5-(2-ethylhexyl)-4-fluorothiophen-2-yl)-6-methylbenzo[1,2-b:4,5-b′]dithiophen-2-yl)thiophen-2-yl)-2-(2-ethylhexyl)-8-(5-methylthiophen-2-yl)-6-octyl-[1,2,3]triazolo[4,5-f]isoindole-5,7(2H,6H)-dione (PBDT-F-TzBI), respectively. The polymers were Soxhlet-extracted with methanol and diethyl ether to remove low molecular weight oligomers, and then with chloroform. The chloroform extracts were precipitated from methanol, filtered and dried to afford PBDT-TzBI and PBDT-F-TzBI. Both polymers were soluble in chloroform, chlorobenzene, and o-dichlorobenzene. The molecular weights of the polymers were determined by gel permeation chromatography (GPC) at 150 °C, using 1,2,4-trichlorobenzene as the eluent. The Mn of PBDT-TzBI and PBDT-F-TzBI were found to be 26.9 and 16.5 kDa, with polydispersity indices of 3.8 and 4.1, respectively.
Thermal properties
The thermal stabilities of the polymers were evaluated by thermogravimetric analysis (TGA), as shown in Fig. S1, ESI.† The decomposition temperature (Td) of PBDT-F-TzBI was above 430 °C, revealing good enough thermal stability for photovoltaic applications.
Optical and electrochemical properties of PBDT-TzBI and PBDT-F-TzBI
The UV-Vis absorption spectra of the polymers were recorded in CHCl3 solution and as thin films coated on glass slides, and are shown in Fig. 1. Both PBDT-TzBI and PBDT-F-TzBI showed broad absorptions in the range between 350 and 700 nm. The thin film absorption of PBDT-TzBI was red-shifted compared to that in CHCl3 solution. This revealed that the PBDT-TzBI had a strong π–π stacking property in the solid state. On the other hand, the bathochromic shift in the solid-state absorption spectrum of PBDT-F-TzBI was much less pronounced than that in solution, indicating the strong aggregation of PBDT-F-TzBI in solution, which can be caused by stronger intermolecular interactions caused by the introduction of fluorine atoms in the polymer backbone. The onsets of absorption of thin-films of PBDT-TzBI and PBDT-F-TzBI were found to be 687 and 684 nm, respectively. Accordingly, the optical band gaps were calculated from these to be 1.80 and 1.81 eV for PBDT-TzBI and PBDT-F-TzBI, respectively. This showed that the presence of fluorine had very little effect on the optical band gap of the polymer, since incorporating F atoms might have resulted in simultaneous lowering of the LUMO and HOMO energy levels of PBDT-F-TzBI.9 The thin film absorptions of PBDT-TzBI and PBDT-F-TzBI were complementary to that of ITIC in the wavelength region from 400 nm to 800 nm (Fig. 1b). Thus, high Jscs could be generated from solar cell devices fabricated from blends of the polymers with ITIC. Temperature-dependent absorbance measurements were done to study the aggregation properties of PBDT-F-TzBI and PBDT-TzBI in o-DCB solution (Fig. S2, ESI†). When the solutions were warmed up from 10 to 90 °C, the absorptions of the polymers were blue-shifted and the absorption maxima were decreased. This proved that the polymers exhibited strong interchain interactions in o-DCB at low temperature.42–44
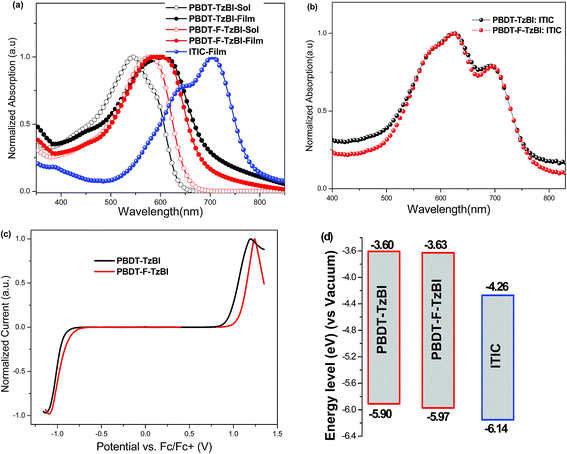 |
| Fig. 1 (a) Normalized UV-Vis absorption spectra of PBDT-TzBI, PBDT-F-TzBI, and ITIC in solutions; (b) normalized UV-Vis absorption spectra of the blends of PBDT-TzBI:ITIC and PBDT-F-TzBI:ITIC in the solid state; (c) square wave voltammograms of PBDT-TzBI and PBDT-F-TzBI; (d) energy level diagram of the polymers and ITIC based on cyclic voltammetry measurements. | |
Square wave voltammetry was used to estimate the frontier molecular orbital energy levels of the polymers. The HOMO and LUMO levels were estimated from the oxidation and reduction peak potentials of the polymers, relative to the ferrocene/ferrocenium (Fc/Fc+) redox couple, using the formula HOMO = −(Eox + 5.13) eV and LUMO = −(Ered + 5.13) eV, where Eox and Ered are the oxidation and reduction peak potentials, respectively. Thus, the HOMO energy levels were determined to be −5.90 and −5.97 eV, for PBDT-TzBI and PBDT-F-TzBI, respectively (Fig. 1, Table 1) while the LUMO levels were found to be −3.60 and −3.63 eVs for PBDT-TzBI and PBDT-F-TzBI, respectively. The HOMO energy levels determined from cyclic voltammetry (CV) measurements (Fig. S3, ESI†) showed similar trends to those obtained from SWV measurements. Under similar conditions, the HOMO and LUMO energy levels of ITIC were determined to be −6.14 and −4.26 eVs (Fig. S3, ESI†). The deeper HOMO energy level of PBDT-F-TzBI is attributed to the inductive electron withdrawing effects of the fluorine atoms from the donor moiety on the polymer backbone.
Table 1 Optical and electrochemical properties of the copolymers
Polymer |
Optical properties |
Electrochemical properties |
λ
max/nm |
λ
onset/nm |
E
optg/eV |
E
oxd./V |
HOMO/eV |
LUMO/eV |
Sol |
Film |
PBDT-TzBI
|
545 |
608 |
687 |
1.80 |
0.77 |
−5.90 |
−3.60 |
PBDT-F-TzBI
|
578 |
598 |
684 |
1.81 |
0.84 |
−5.97 |
−3.63 |
Photovoltaic properties
The photovoltaic performances of PBDT-TzBI and PBDT-F-TzBI were investigated using ITIC as an acceptor with the device geometry ITO/PEDOT:PSS/polymer:ITIC (1:1)/PDINO/Al (PDINO = perylene diimide functionalized with amino N-oxide which is used as an electron transport layer) and were characterized under AM 1.5G illumination at 100 mW cm−2 with a solar simulator. The photovoltaic parameters of the polymers are summarized in Table 2. Fig. 2 shows J–V curves and the external quantum efficiency (EQE) spectra of PBDT-TzBI:ITIC- and PBDT-F-TzBI:ITIC-based solar cell devices.
Table 2 Photovoltaic parameters of devices measured under AM 1.5G, 100 mW cm−2
Donor:acceptor |
V
oc [V] |
J
sc [mA cm−2] |
FF |
PCE [%] |
The devices were thermally annealed at 160 °C for 10 min.
0.5% DPE additive and CB vapor annealing was used.
Photocurrents obtained by integrating the EQE with the AM 1.5G spectrum are given in the parentheses.
|
PBDT-TzBI-:ITIC |
0.88 |
16.37 |
0.64 |
9.22 |
PBDT-F-TzBI:ITIC |
0.97 |
16.72 |
0.68 |
11.02 |
PBDT-TzBI-:ITICa |
0.84 |
17.78(17.16)c |
0.69 |
10.24 |
PBDT-F-TzBI:ITICa |
0.97 |
18.00 |
0.70 |
11.98 |
PBDT-F-TzBI:ITICb |
0.93 |
18.10(17.47)c |
0.72 |
12.12 |
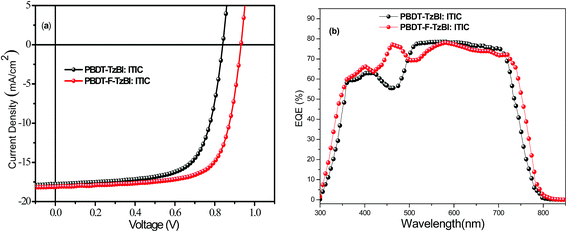 |
| Fig. 2 (a) Current density–voltage characteristics, (b) EQE curves, of PBDT-TzBI:ITIC- and PBDT-F-TzBI:ITIC-based solar cells. | |
The OSC devices made from PBDT-TzBI:ITIC exhibited a PCE of 9.22% with a Voc of 0.88 V, Jsc of 16.37 mA cm−2 and FF of 0.64, while the device based on PBDT-F-TzBI:ITIC scored a PCE of 11.02% with a Voc of 0.97 V, Jsc of 16.72 mA cm−2 and FF of 0.68. PBDT-F-TzBI-based devices exhibited higher Voc compared with PBDT-TzBI-based devices as a result of the presence of fluorine atoms that make the HOMO levels of the polymer deeper. Thermal annealing of BHJ solar cells is known to support the formation of favorable morphology by tuning the domain sizes and aggregation properties of certain donor and acceptor materials.6,45 Hence, to improve the Jsc and FF of our PSCs, the devices were thermally annealed at 160 °C for 10 min. The PCEs of the devices made from blends of PBDT-TzBI:ITIC and PBDT-F-TzBI:ITIC were both improved to 10.24% and 11.98%, respectively, due to simultaneous improvements in both Jsc and FF as shown in Table 2. It is known that efficient exciton dissociation and charge transfer requires bicontinuous interpenetrating networks between electron donors and acceptors in the BHJ active layer of the solar cell.9 Solvent additives facilitate molecular reorganization of the donor and acceptor materials and lead to the development of different crystalline structures in the active layers. This promotes the formation of the desired morphology for efficient exciton dissociation and charge transport in the BHJ.35,46 Favorable morphology is a characteristic nature of PSCs to facilitate charge transport properties that enhance the performance by improving Jsc and FF. Thus, when diphenyl ether (DPE) (0.5%) was used as an additive and CB solvent vapor annealing was conducted, the PCE of the PBDT-F-TzBI-based device increased to 12.12% with a Voc of 0.93 V, Jsc of 18.10 mA cm−2 and FF of 0.72. The slightly improved Jsc and FF of the device may be due to the favorable molecular orientation caused by the processing additive. The high PCE of the PBDT-F-TzBI:ITIC-based solar cell device makes PBDT-F-TzBI as one of the promising candidates for practical application in OSCs.
To evaluate the spectral responses of the PSCs and the accuracies of the photocurrents from the J–V measurements, external quantum efficiency (EQE) measurements were carried out (Fig. 2). Both PBDT-TzBI:ITIC- and PBDT-F-TzBI:ITIC-based solar cells showed maximum photoresponses with EQEs of more than 75% in the range between 500 and 700 nm. The photoresponses over the region between 300 and 800 nm in the EQE curves are consistent with the absorption profiles of the blend films suggesting that both the donor and acceptor materials contributed to the photocurrents. The photocurrents calculated by integrating the EQE spectra with AM 1.5G solar spectrum are consistent with the Jsc values obtained from the J–V measurements, with less than 5% difference.
Film morphology
Atomic force microscopy (AFM) and transmission electron microscopy (TEM) measurements were employed to study the surface roughness and phase separation of the blend films, and the images are shown in Fig. 3. The PBDT-F-TzBI:ITIC and PBDT-TzBI:ITIC blend films showed smooth surfaces with root mean-square (RMS) roughnesses of 0.97 and 1.07 nm, respectively. The presence of fluorine in PBDT-F-TzBI exerted limited effect on the morphology of the blend as indicated by AFM. The TEM images of the blends presented very fine features, indicating good miscibility with nanometer-scale microphase separation between the donor and the acceptor materials, which is favorable for charge separation and transport.35 The AFM and TEM images of the blends revealed that the film morphologies of both blend films were optimal but cannot tell the reason for the higher Jsc and FF of PBDT-F-TzBI:ITIC-based devices.
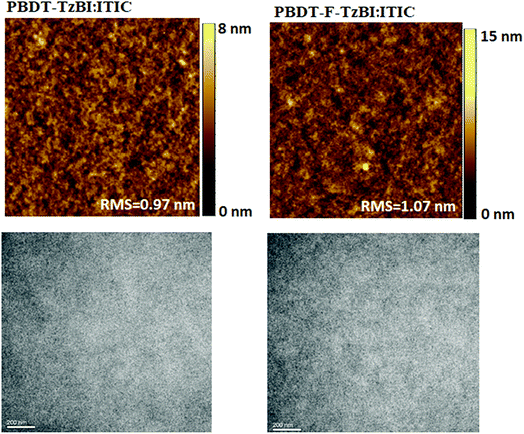 |
| Fig. 3 AFM topography (5 × 5 nm) and TEM images of the optimized PBDT-F-TzBI:ITIC and PBDT-TzBI:ITIC blend films. | |
Charge carrier mobility
To characterize the charge transport properties of the polymer:ITIC blends, the electron (μe) and hole (μh) mobilities of the PSCs were measured by the space charge limited current (SCLC) method using electron-only devices with the configuration ITO/ZnO/active layer/PIDNO/Al and hole-only devices with the configuration ITO/PEDOT:PSS/active layer/Au, respectively. The corresponding hole and electron mobilities are summarized in Table 3. The PBDT-TzBI:ITIC and PBDT-F-TzBI:ITIC blend films exhibited decent μh and μe in the order of 10−4 cm2 V−1 s−1, which were comparable with those of other TzBI-based polymers.36 Relatively higher and balanced μh and μe were found for the PBDT-F-TzBI:ITIC blend than for the PBDT-TzBI:ITIC blend. It is known that balanced hole and electron mobilities can reduce bimolecular recombination to give high photocurrent and FF, which supports the higher Jsc and FF of PBDT-F-TzBI:ITIC-based solar cells.10,44,47,48
Table 3 SCLC hole and electron mobilities of the blend films
Donor:acceptor |
SCLC μh (cm2 V−1 s−1) |
SCLC μe (cm2 V−1 s−1) |
μ
h/μe |
PBDT-TzBI:ITIC |
1.1×10−4 |
1.6×10−4 |
0.69 |
PBDT-F-TzBI:ITIC |
2.0×10−4 |
2.3×10−4 |
0.87 |
Exciton dissociation and charge recombination behavior
To study the exciton dissociation probability in PBDT-TzBI- and PBDT-F-TzBI-based devices, the photocurrent density (Jph) was evaluated as a function of the effective voltage (Veff) as shown in Fig. 4. Jph is the difference between illuminated and dark current, and Veff is the difference between Voc and Va, where Voc is the voltage at zero Jph and Va is the applied voltage. The exciton dissociation probability of a device can be calculated as Pdiss = Jph/Jsat, where Jsat stands for saturation photocurrent density.49–51 Under short circuit conditions, the Pdisss of PBDT-TzBI:ITIC- and PBDT-F-TzBI:ITIC-based devices were calculated to be 95.8% and 97.4%, respectively. The relatively higher Pdiss of the PBDT-F-TzBI-based device showed better exciton dissociation and charge collection than its PBDT-TzBI-based counterpart, which partly contributed to the higher Jsc and FF of the PBDT-F-TzBI-based devices.12,51,52
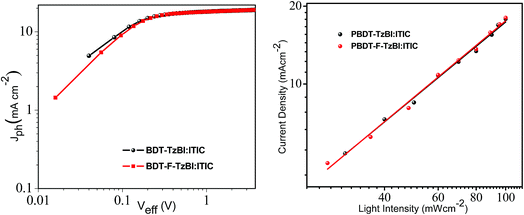 |
| Fig. 4 (a) Jph−Veff characteristics and (b) dependence of Jsc on the light intensity of PBDT-TzBI-and PBDT-F-TzBI-based devices. | |
To investigate the charge recombination behaviors of the devices, we studied the effect of light intensity (Plight) on the short-circuit current density (Jsc) of the PSCs (Fig. 4). Plight and Jsc are related to each other according to the power law as Jsc ∝ PS. The value of S should be 1 when all free carriers are swept out and collected at the electrodes prior to recombination, whereas when the value of S is less than 1, the presence of some degree of bimolecular recombination is anticipated.53–55 The S value of PBDT-F-TzBI:ITIC is 0.98, which is closer to 1, and indicates the presence of weak bimolecular recombination and efficient carrier transportation compared to PBDT-TzBI:ITIC (S = 0.97). This reduced bimolecular recombination correlates with the higher and balanced charge carrier mobility of the corresponding device that contributed partly to its higher Jsc and FF.54 We have thus demonstrated relatively higher and more balanced carrier mobility, better exciton dissociation and weaker bimolecular recombination for PBDT-F-TzBI-based devices justifying the higher Jsc and FF that resulted in a higher PCE (>12%) compared to their non-fluorinated analogs.
In conclusion, two wide band gap donor polymers, PBDT-TzBI and PBDT-F-TzBI, were successfully synthesized by polymerization of TzBI-based monomer 1 with BDT-based monomers (4,8-bis(5-(2-ethylhexyl)thiophen-2-yl)benzo[1,2-b:4,5-b′]dithiophene-2,6-diyl)bis(trimethylstannane) (2) and (4,8-bis(5-(2-ethylhexyl)-4-fluorothiophen-2-yl)benzo[1,2-b:4,5-b′]dithiophene-2,6-diyl)bis(trimethylstannane) (3), respectively. The PSCs fabricated from blends of PBDT-TzBI and PBDT-F-TzBI with ITIC annealed at 160 °C showed high PCEs of 10.24% and 11.98%, respectively. The presence of fluorine atom on the backbone of PBDT-F-TzBI lowered its HOMO energy level and improved its Voc compared to PBDT-TzBI-based devices. Moreover, due to the presence of fluorine, higher charge carrier mobility, exciton dissociation probability and weak bimolecular recombination were observed for the PBDT-F-TzBI-based device. These properties explained the higher Jsc and FF of the PBDT-F-TzBI-based device that enhanced its overall performance compared to its PBDT-TzBI-based counterpart. Using DPE (0.5%) as a processing additive and vapor phase annealing with CB, the PCE of the PBDT-F-TzBI-based device improved to 12.12% with a Voc of 0.93 V, Jsc of 18.10 mA cm−2 and FF of 0.72, which is the highest value reported so far for TzBI-based polymers using ITIC as an acceptor. The results showed that PBDT-F-TzBI is among the most promising candidates for application in OSCs.
Experimental section
Materials characterization
The intermediate compounds and monomers were characterized by 1H NMR (400 MHz) and 13C NMR (100 MHz) using a Bruker Avance 400 NMR spectrometer. The molecular weights of the polymers were determined by size exclusion chromatography (SEC) on a Waters Alliance GPCV2000 with a refractive index detector using 1,2,4-trichlorobenzene as the eluent at 150 °C with a polystyrene standard calibration. Thermogravimetric analysis (TGA) was carried out on a METTLER TOLEDO thermogravimetric analyzer TGA/DSC 3+, from 50 to 550 °C at a heating rate of 10 °C min−1 under N2 flow. A Perkin Elmer Lambda 900 UV-Vis-NIR spectrometer was used to measure the absorptions of the polymers. Square wave voltammetry (SWV) measurements were done on a CH-Instruments 650A electrochemical workstation using a three-electrode system. Platinum wires were used for both working and counter electrodes while Ag/Ag+ was used as the reference electrode calibrated with the ferrocene/ferrocenium couple (Fc/Fc+). The supporting electrolyte was tetrabutylammoniumhexafluorophosphate (Bu4NPF6) solution (0.1 M) in anhydrous acetonitrile.
PSC fabrication and characterization
Device fabrication and evaluations.
Photovoltaic devices were fabricated with a conventional device structure of ITO/PEDOT:PSS/polymer:ITIC/PDINO/Al. The patterned ITO glass (sheet resistance = 15 Ω per square) was pre-cleaned in an ultrasonic bath with ITO detergent, deionized water, acetone, and isopropyl alcohol and treated in an ultraviolet-ozone chamber (PREEN II-862) for 13 min, then exposed to oxygen plasma for 3 min, twice. A thin layer (about 30 nm) of PEDOT:PSS was then spin-coated onto the ITO glass at 4000 rpm and baked at 150 °C for 15 min. Solutions of polymer/ITIC in CB were stirred overnight and spin-coated on top of the PEDOT:PSS layer to form the active layer of about 110–150 nm. The thickness of the active layer was measured using a Veeco Dektak 150 profilometer. PIDNO solution (in CH3OH) was then spin-coated as an electron transfer layer. Finally, an Al (100 nm) metal electrode was thermally evaporated at about 4 × 10−4 Pa and the device area of 0.1 cm2 was defined by a shadow mask. The current density–voltage (J–V) characteristics were recorded with a Keithley 2400 source measurement unit under simulated 100 mW cm−2 irradiation from a Newport solar simulator. The external quantum efficiencies (EQEs) were analyzed using a certified Newport incident photon conversion efficiency (IPCE) measurement system.
Conflicts of interest
There are no conflicts to declare.
Acknowledgements
W. Mammo, B. A. Abdulahi, B. K. and Z. G. acknowledge financial support from the International Science Programme (ISP), Uppsala University, Sweden. E. Wang thanks the Swedish Research Council, the Swedish Research Council Formas and The Knut and Alice Wallenberg Foundation (2017.0186, 2016.0059) for financial support. R. Yang thanks the National Natural Science Foundation of China (51773220, 21728401) for financial support.
References
- G. Zhang, J. Zhao, P. C. Y. Chow, K. Jiang, J. Zhang, Z. Zhu, J. Zhang, F. Huang and H. Yan, Nonfullerene Acceptor Molecules for Bulk Heterojunction Organic Solar Cells, Chem. Rev., 2018, 118(7), 3447 CrossRef CAS PubMed.
- M. C. Scharber and N. S. Sariciftci, Efficiency of bulk-heterojunction organic solar cells, Prog. Polym. Sci., 2013, 38(12), 1929 CrossRef CAS PubMed.
- Z. C. Hu, L. Ying, F. Huang and Y. Cao, Towards a bright future: polymer solar cells with power conversion efficiencies over 10%, Sci. China: Chem., 2017, 60(5), 571 CrossRef CAS.
- K. A. Mazzio and C. K. Luscombe, The future of organic photovoltaics, Chem. Soc. Rev., 2015, 44(1), 78 RSC.
- W. Cao and J. Xue, Recent progress in organic photovoltaics: device architecture and optical design, Energy Environ. Sci., 2014, 7(7), 2123 RSC.
- L. Lu, T. Zheng, Q. Wu, A. M. Schneider, D. Zhao and L. Yu, Recent Advances in Bulk Heterojunction Polymer Solar Cells, Chem. Rev., 2015, 115(23), 12666 CrossRef CAS PubMed.
- C. B. Nielsen, S. Holliday, H.-Y. Chen, S. J. Cryer and I. McCulloch, Non-Fullerene Electron Acceptors for Use in Organic Solar Cells, Acc. Chem. Res., 2015, 48(11), 2803 CrossRef CAS PubMed.
- D. He, F. Zhao, J. Xin, J. J. Rech, Z. Wei, W. Ma, W. You, B. Li, L. Jiang, Y. Li and C. Wang, A Fused Ring Electron Acceptor with Decacyclic Core Enables over 13.5% Efficiency for Organic Solar Cells, Adv. Energy Mater., 2018, 8(30), 1802050 CrossRef.
- A. Tang, B. Xiao, F. Chen, J. Zhang, Z. Wei and E. Zhou, The Introduction of Fluorine and Sulfur Atoms into Benzotriazole-Based p-Type Polymers to Match with a Benzotriazole-Containing n-Type Small Molecule: “The Same-Acceptor-Strategy” to Realize High Open-Circuit Voltage, Adv. Energy Mater., 2018, 8(25), 1801582 CrossRef.
- Z. Li, X. Xu, W. Zhang, X. Meng, W. Ma, A. Yartsev, O. Inganäs, M. R. Andersson, R. A. J. Janssen and E. Wang, High Performance All-Polymer Solar Cells by Synergistic Effects of Fine-Tuned Crystallinity and Solvent Annealing, J. Am. Chem. Soc., 2016, 138(34), 10935 CrossRef CAS PubMed.
- J. Yuan, Y. Zhang, L. Zhou, G. Zhang, H.-L. Yip, T.-K. Lau, X. Lu, C. Zhu, H. Peng, P. A. Johnson, M. Leclerc, Y. Cao, J. Ulanski, Y. Li and Y. Zou, Single-Junction Organic Solar Cell with over 15% Efficiency Using Fused-Ring Acceptor with Electron-Deficient Core, Joule, 2019, 3(4), 1140 CrossRef CAS.
- Y. Wang, Y. Zhang, N. Qiu, H. Feng, H. Gao, B. Kan, Y. Ma, C. Li, X. Wan and Y. Chen, A Halogenation Strategy for over 12% Efficiency Nonfullerene Organic Solar Cells, Adv. Energy Mater., 2018, 8(15), 1702870 CrossRef.
- W. Zhao, S. Li, H. Yao, S. Zhang, Y. Zhang, B. Yang and J. Hou, Molecular Optimization Enables over 13% Efficiency in Organic Solar Cells, J. Am. Chem. Soc., 2017, 139(21), 7148 CrossRef CAS PubMed.
- H. Zhang, H. Yao, J. Hou, J. Zhu, J. Zhang, W. Li, R. Yu, B. Gao, S. Zhang and J. Hou, Over 14% Efficiency in Organic Solar Cells Enabled by Chlorinated Nonfullerene Small-Molecule Acceptors, Adv. Mater., 2018, 30(28), 1800613 CrossRef PubMed.
- Z. Luo, G. Li, W. Gao, K. Wu, Z.-G. Zhang, B. Qiu, H. Bin, L. Xue, F. Liu, Y. Li and C. Yang, A universal nonfullerene electron acceptor matching with different band-gap polymer donors for high-performance polymer solar cells, J. Mater. Chem.A, 2018, 6(16), 6874 RSC.
- B. Fan, D. Zhang, M. Li, W. Zhong, Z. Zeng, L. Ying, F. Huang and Y. Cao, Achieving over 16% efficiency for single-junction organic solar cells, Sci. China: Chem., 2019, 62(6), 746 CrossRef CAS.
- Y. Zou, A. Najari, P. Berrouard, S. Beaupré, B. Réda Aïch, Y. Tao and M. Leclerc, A Thieno[3,4-c]pyrrole-4,6-dione-Based Copolymer for Efficient Solar Cells, J. Am. Chem. Soc., 2010, 132(15), 5330 CrossRef CAS PubMed.
- C. Cabanetos, A. El Labban, J. A. Bartelt, J. D. Douglas, W. R. Mateker, J. M. J. Fréchet, M. D. McGehee and P. M. Beaujuge, Linear Side Chains in Benzo[1,2-b:4,5-b′]dithiophene–Thieno[3,4-c]pyrrole-4,6-dione Polymers Direct Self-Assembly and Solar Cell Performance, J. Am. Chem. Soc., 2013, 135(12), 4656 CrossRef CAS PubMed.
- J. Warnan, C. Cabanetos, A. E. Labban, M. R. Hansen, C. Tassone, M. F. Toney and P. M. Beaujuge, Ordering Effects in Benzo[1,2-b:4,5-b′]difuran-thieno[3,4-c]pyrrole-4,6-dione Polymers with >7% Solar Cell Efficiency, Adv. Mater., 2014, 26(25), 4357 CrossRef CAS PubMed.
- S. Chen, H. Yao, Z. Li, O. M. Awartani, Y. Liu, Z. Wang, G. Yang, J. Zhang, H. Ade and H. Yan, Surprising Effects upon Inserting Benzene Units into a Quaterthiophene-Based D–A Polymer–Improving Non-Fullerene Organic Solar Cells via Donor Polymer Design, Adv. Energy Mater., 2017, 7(12), 1602304 CrossRef.
- H. Yao, R. Yu, T. J. Shin, H. Zhang, S. Zhang, B. Jang, M. A. Uddin, H. Y. Woo and J. Hou, A Wide Bandgap Polymer with Strong π–π Interaction for Efficient Fullerene-Free Polymer Solar Cells, Adv. Energy Mater., 2016, 6(15), 1600742 CrossRef.
- C. L. Chochos, N. Leclerc, N. Gasparini, N. Zimmerman, E. Tatsi, A. Katsouras, D. Moschovas, E. Serpetzoglou, I. Konidakis, S. Fall, P. Lévêque, T. Heiser, M. Spanos, V. G. Gregoriou, E. Stratakis, T. Ameri, C. J. Brabec and A. Avgeropoulos, The role of chemical structure in indacenodithienothiophene-alt-benzothiadiazole copolymers for high performance organic solar cells with improved photo-stability through minimization of burn-in loss, J. Mater. Chem. A, 2017, 5(47), 25064 RSC.
- E. Wang, L. Hou, Z. Wang, S. Hellström, W. Mammo, F. Zhang, O. Inganäs and M. R. Andersson, Small Band Gap Polymers Synthesized via a Modified Nitration of 4,7-Dibromo-2,1,3-benzothiadiazole, Org. Lett., 2010, 12(20), 4470 CrossRef CAS PubMed.
- H. Zhou, L. Yang, A. C. Stuart, S. C. Price, S. Liu and W. You, Development of Fluorinated Benzothiadiazole as a Structural Unit for a Polymer Solar Cell of 7% Efficiency, Angew.Chem., Int. Ed., 2011, 50(13), 2995 CrossRef CAS PubMed.
- M. Helgesen, S. A. Gevorgyan, F. C. Krebs and R. A. J. Janssen, Substituted 2,1,3-Benzothiadiazole- And Thiophene-Based Polymers for Solar Cells – Introducing a New Thermocleavable Precursor, Chem. Mater., 2009, 21(19), 4669 CrossRef CAS.
- P. Murto, S. Tang, C. Larsen, X. Xu, A. Sandström, J. Pietarinen, B. Bagemihl, B. A. Abdulahi, W. Mammo, M. R. Andersson, E. Wang and L. Edman, Incorporation of Designed Donor–Acceptor–Donor Segments in a Host Polymer for Strong Near-Infrared Emission from a Large-Area Light-Emitting Electrochemical Cell, ACS Appl. Energy Mater., 2018, 1(4), 1753 CrossRef CAS.
- J. Zhao, Y. Li, G. Yang, K. Jiang, H. Lin, H. Ade, W. Ma and H. Yan, Efficient organic solar cells processed from hydrocarbon solvents, Nat. Energy, 2016, 1, 15027 CrossRef CAS.
- H. Bin, L. Gao, Z.-G. Zhang, Y. Yang, Y. Zhang, C. Zhang, S. Chen, L. Xue, C. Yang, M. Xiao and Y. Li, 11.4% Efficiency non-fullerene polymer solar cells with trialkylsilyl substituted 2D-conjugated polymer as donor, Nat. Commun., 2016, 7, 13651 CrossRef CAS PubMed.
- Z. Genene, J. Wang, X. Meng, W. Ma, X. Xu, R. Yang, W. Mammo and E. Wang, High Bandgap (1.9 eV) Polymer with Over 8% Efficiency in Bulk Heterojunction Solar Cells, Adv. Electron. Mater., 2016, 2(7), 1600084 CrossRef.
- Z. Li, X. Xu, W. Zhang, X. Meng, Z. Genene, W. Ma, W. Mammo, A. Yartsev, M. R. Andersson, R. A. J. Janssen and E. Wang, 9.0% power conversion efficiency from ternary all-polymer solar cells, Energy Environ. Sci., 2017, 10, 2212 RSC.
- X. Li, G. Huang, N. Zheng, Y. Li, X. Kang, S. Qiao, H. Jiang, W. Chen and R. Yang, High-Efficiency Polymer Solar Cells Over 13.9% With a High VOC Beyond 1.0V by Synergistic Effect of Fluorine and Sulfur, Sol. RRL, 2019, 3(4), 1900005 CrossRef.
- L. Wang, D. Cai, Q. Zheng, C. Tang, S.-C. Chen and Z. Yin, Low Band Gap Polymers Incorporating a Dicarboxylic Imide-Derived Acceptor Moiety for Efficient Polymer Solar Cells, ACS Macro Lett., 2013, 2(7), 605 CrossRef CAS.
- C. B. Nielsen, R. S. Ashraf, N. D. Treat, B. C. Schroeder, J. E. Donaghey, A. J. P. White, N. Stingelin and I. McCulloch, 2,1,3-Benzothiadiazole-5,6-Dicarboxylic Imide – A Versatile Building Block for Additive- and Annealing-Free Processing of Organic Solar Cells with Efficiencies Exceeding 8%, Adv. Mater., 2015, 27(5), 948 CrossRef CAS PubMed.
- L. Lan, Z. Chen, Q. Hu, L. Ying, R. Zhu, F. Liu, T. P. Russell, F. Huang and Y. Cao, High-Performance Polymer Solar Cells Based on a Wide-Bandgap Polymer Containing Pyrrolo[3,4-f]benzotriazole-5,7-dione with a Power Conversion Efficiency of 8.63%, Adv. Sci., 2016, 3(9), 1600032 CrossRef PubMed.
- B. Fan, K. Zhang, X.-F. Jiang, L. Ying, F. Huang and Y. Cao, High-Performance Nonfullerene Polymer Solar Cells based on Imide-Functionalized Wide-Bandgap Polymers, Adv. Mater., 2017, 29(21), 1606396 CrossRef PubMed.
- P. Zhu, B. Fan, X. Du, X. Tang, N. Li, F. Liu, L. Ying, Z. Li, W. Zhong, C. J. Brabec, F. Huang and Y. Cao, Improved Efficiency of Polymer Solar Cells by Modifying the Side Chain of Wide-Band Gap Conjugated Polymers Containing Pyrrolo[3,4-f]benzotriazole-5,7(6H)-dione Moiety, ACS Appl. Mater. Interfaces, 2018, 10(26), 22495 CrossRef CAS PubMed.
- B. Fan, X. Du, F. Liu, W. Zhong, L. Ying, R. Xie, X. Tang, K. An, J. Xin, N. Li, W. Ma, C. J. Brabec, F. Huang and Y. Cao, Fine-tuning of the chemical structure of photoactive materials for highly efficient organic photovoltaics, Nat. Energy, 2018, 3, 1051 CrossRef CAS.
- D. Dang, W. Chen, R. Yang, W. Zhu, W. Mammo and E. Wang, Fluorine substitution enhanced photovoltaic performance of a D–A1–D–A2 copolymer, Chem. Commun., 2013, 49(81), 9335 RSC.
- K. Kawashima, T. Fukuhara, Y. Suda, Y. Suzuki, T. Koganezawa, H. Yoshida, H. Ohkita, I. Osaka and K. Takimiya, Implication of Fluorine Atom on Electronic Properties, Ordering Structures, and Photovoltaic Performance in Naphthobisthiadiazole-Based Semiconducting Polymers, J. Am. Chem. Soc., 2016, 138(32), 10265 CrossRef CAS PubMed.
- M. Zhang, X. Guo, S. Zhang and J. Hou, Synergistic Effect of Fluorination on Molecular Energy Level Modulation in Highly Efficient Photovoltaic Polymers, Adv. Mater., 2014, 26(7), 1118 CrossRef CAS PubMed.
- K. Reichenbächer, H. I. Süss and J. Hulliger, Fluorine in crystal engineering—“the little atom that could”, Chem. Soc. Rev., 2005, 34(1), 22 RSC.
- W. Huang, M. Li, L. Zhang, T. Yang, Z. Zhang, H. Zeng, X. Zhang, L. Dang and Y. Liang, Molecular Engineering on Conjugated Side Chain for Polymer Solar Cells with Improved Efficiency and Accessibility, Chem. Mater., 2016, 28(16), 5887 CrossRef CAS.
- S. Zhang, B. Yang, D. Liu, H. Zhang, W. Zhao, Q. Wang, C. He and J. Hou, Correlations among Chemical Structure, Backbone Conformation, and Morphology in Two Highly Efficient Photovoltaic Polymer Materials, Macromolecules, 2016, 49(1), 120 CrossRef CAS.
- B. A. Abdulahi, X. Xu, P. Murto, O. Inganäs, W. Mammo and E. Wang, Open-Circuit Voltage Modulations on All-Polymer Solar Cells by Side Chain Engineering on 4,8-Di(thiophen-2-yl)benzo[1,2-b:4,5-b′]dithiophene-Based Donor Polymers, ACS Appl. Energy Mater., 2018, 1(6), 2918 CrossRef CAS.
- H. Benten, D. Mori, H. Ohkita and S. Ito, Recent research progress of polymer donor/polymer acceptor blend solar cells, J. Mater. Chem. A, 2016, 4(15), 5340 RSC.
- S. Kwon, H. Kang, J.-H. Lee, J. Lee, S. Hong, H. Kim and K. Lee, Effect of Processing Additives on Organic Photovoltaics: Recent Progress and Future Prospects, Adv. Energy Mater., 2017, 7(10), 1601496 CrossRef.
- C. M. Proctor, M. Kuik and T.-Q. Nguyen, Charge carrier recombination in organic solar cells, Prog. Polym. Sci., 2013, 38(12), 1941 CrossRef CAS.
- Z. Li, X. Xu, G. Zhang, T. Yu, Y. Li and Q. Peng, Highly Efficient Non-Fullerene Polymer Solar Cells Enabled by Wide Bandgap Copolymers With Conjugated Selenyl Side Chains, Sol. RRL, 2018, 2(10), 1800186 CrossRef.
- J.-L. Wu, F.-C. Chen, Y.-S. Hsiao, F.-C. Chien, P. Chen, C.-H. Kuo, M. H. Huang and C.-S. Hsu, Surface Plasmonic Effects of Metallic Nanoparticles on the Performance of Polymer Bulk Heterojunction Solar Cells, ACS Nano, 2011, 5(2), 959 CrossRef CAS PubMed.
- B. Fan, L. Ying, Z. Wang, B. He, X.-F. Jiang, F. Huang and Y. Cao, Optimisation of processing solvent and molecular weight for the production of green-solvent-processed all-polymer solar cells with a power conversion efficiency over 9%, Energy Environ. Sci., 2017, 10(5), 1243 RSC.
- S. Li, L. Ye, W. Zhao, S. Zhang, S. Mukherjee, H. Ade and J. Hou, Energy-Level Modulation of Small-Molecule Electron Acceptors to Achieve over 12% Efficiency in Polymer Solar Cells, Adv. Mater., 2016, 28(42), 9423 CrossRef CAS PubMed.
- F. Zhao, S. Dai, Y. Wu, Q. Zhang, J. Wang, L. Jiang, Q. Ling, Z. Wei, W. Ma, W. You, C. Wang and X. Zhan, Single-Junction Binary-Blend Nonfullerene Polymer Solar Cells with 12.1% Efficiency, Adv. Mater., 2017, 29(18), 1700144 CrossRef PubMed.
- A. K. K. Kyaw, D. H. Wang, D. Wynands, J. Zhang, T.-Q. Nguyen, G. C. Bazan and A. J. Heeger, Improved Light Harvesting and Improved Efficiency by Insertion of an Optical Spacer (ZnO) in Solution-Processed Small-Molecule Solar Cells, Nano Lett., 2013, 13(8), 3796 CrossRef CAS PubMed.
- Q. Fan, W. Su, X. Guo, Y. Wang, J. Chen, C. Ye, M. Zhang and Y. Li, Side-chain engineering for efficient non-fullerene polymer solar cells based on a wide-bandgap polymer donor, J. Mater. Chem. A, 2017, 5(19), 9204 RSC.
- J. K. J. vanDuren, X. Yang, J. Loos, C. W. T. Bulle-Lieuwma, A. B. Sieval, J. C. Hummelen and R. A. J. Janssen, Relating the Morphology of Poly(p-phenylene vinylene)/Methanofullerene Blends to Solar-Cell Performance, Adv. Funct. Mater., 2004, 14(5), 425 CrossRef CAS.
Footnote |
† Electronic supplementary information (ESI) available. See DOI: 10.1039/c9ta06385d |
|
This journal is © The Royal Society of Chemistry 2019 |