DOI:
10.1039/C9TA01532A
(Paper)
J. Mater. Chem. A, 2019,
7, 10971-10979
Fullerene as an efficient hybridization matrix for exploring high-performance layered-double-hydroxide-based electrodes†
Received
10th February 2019
, Accepted 2nd April 2019
First published on 2nd April 2019
Abstract
2D nanostructured fullerene (C60) nanosheets (NSs) with a strong electron-withdrawing ability are employed as a hybridization matrix for improving the electrode performance of an inorganic solid. The electrostatically-driven self-assembly of anionic C60 NSs with cationic Ni–Fe-layered double hydroxide (LDH) NSs yields a strongly-coupled nanohybrid of C60–LDH. The hybridization between fullerene and Ni–Fe-LDH NSs leads not only to the formation of a mesoporous stacking structure but also to the occurrence of strong interfacial electron transfer from Ni–Fe-LDH to C60. The obtained C60–Ni–Fe-LDH nanohybrids deliver much greater specific capacitances with better rate characteristics than the graphene–Ni–Fe-LDH nanohybrid and the pristine Ni–Fe-LDH, highlighting the superior role of fullerene as a hybridization matrix over graphene in improving the supercapacitor electrode performance of the LDH material. Such remarkable advantages of fullerene incorporated are attributable to the increase of porosity, the significant contribution of the redox process of fullerene, the improvement of charge transfer kinetics, and the enhancement of surface electrophilicity due to an electronic coupling with electron-withdrawing fullerene. The present study underscores that hybridization with fullerene provides an effective way of exploring a new family of high-performance electrochemistry-related functional nanohybrids.
Introduction
Hybridization of an inorganic solid with a low-dimensional nanostructure can provide an effective synthetic route to novel multifunctional hybrid materials with improved performances.1,2 One of the best candidates for a hybridization matrix is nanostructured carbon species like 2D graphene and 1D carbon nanotube (CNT), because the coupling with these carbonaceous compounds leads to the improvement of electrical conductivity and electrochemical activity of hybridized species.3–8 Also, hybridization with these nanocarbon species results in the expansion of the surface area via the depression of crystal agglomeration. Many functionalities of diverse inorganic solids like metal hydroxides, metal oxides, and metal chalcogenides can be remarkably enhanced by coupling with these nanocarbon species.9–11 In one instance, hybridization with 1D CNT and 2D graphene is highly effective in improving the electrode, electrocatalyst, and photocatalyst performances of layered double hydroxide (LDH).12–14 In contrast to these nanocarbons, fullerene (C60) has seldom been employed as a hybridization matrix for inorganic solids. Fullerene boasts many valuable physicochemical properties such as high electrical conductivity and high charge mobility like the other nanocarbon species.15,16 In contrast to graphene and CNT, fullerene shows high electrochemical activity for the redox process, which is beneficial for the electrode application.17 One thing of prime importance is that many unique properties of fullerene originate from its strong electron-withdrawing ability,18,19 providing a powerful method to efficiently modify the electronic structures of hybridized species. Since fullerene possesses a negative surface charge due to its electron-rich surface nature, an electrostatic interaction allows to easily couple fullerene with positively-charged exfoliated LDH nanosheets (NSs).20 The very thin thickness and wide 2D surface of the exfoliated LDH NSs enable achieving unusually strong electronic coupling with fullerene, resulting in the significant modification of the electronic structure of LDH.21 Additionally, the hybridization of LDH NSs with C60 can enhance its porosity and surface area by preventing severe stacking of LDH crystallites. Such a synergistic coupling between LDH and fullerene is supposed to be quite useful in exploring novel high-performance electrodes and electrocatalysts. Despite remarkable advantages of fullerene as the hybridization matrix, at the time of this submission, we are unaware of any other study about fullerene–LDH nanohybrids with excellent electrode functionality.
In this work, intimately-coupled fullerene–LDH nanohybrids are synthesized by the electrostatically-driven self-assembly of C60 NSs and exfoliated LDH NSs. This is the first report about the 2D–2D nanohybrid composed of fullerene NSs and exfoliated LDH NSs. Evolution of the electrode performance of LDH upon the incorporation of fullerene is investigated together with the crystal and hybrid structures of the C60–LDH nanohybrids.
Experimental
Preparation
The pristine Ni–Fe-LDH material was synthesized by co-precipitation between 6.6 mM Ni(NO3)2·6H2O and 3.3 mM Fe(NO3)3·9H2O in 1.0 M NaOH solution at the pH of 8 occurring for 6 h under N2 bubbling, as reported previously.22 The exfoliation of Ni–Fe-LDH was carried out by the dispersion of the pristine LDH material in formamide for 48 h under N2 bubbling to avoid carbonate contamination.23 The ratio of Ni–Fe-LDH to formamide was adjusted to 1 mg/1 mL, leading to the formation of the stable colloidal suspension of Ni–Fe-LDH NSs (Fig. S1†). Another precursor of C60 was also prepared in the form of a toluene-based suspension (Fig. S1†). The suspension of C60 was obtained by dispersing C60 powder (Sigma-Aldrich, 98.0% purity) in toluene at a concentration of 1 mg/1 mL. According to the zeta potential measurement, the exfoliated Ni–Fe LDH NSs showed a zeta potential of +39 mV, whereas a negative zeta potential of −88 mV occurred for C60, indicating their opposite surface charges (Fig. S2†). As illustrated in Fig. 1a, the intimately-coupled C60–Ni–Fe-LDH nanohybrids were synthesized by an electrostatically-driven self-assembly between exfoliated Ni–Fe-LDH NSs and C60. Both the precursor suspensions were with mixed each other with the addition of isopropanol.24 In the present liquid–liquid interfacial precipitation method, a good solvent for C60 (i.e. toluene) was faced with a poor solvent for C60 (i.e. isopropanol), leading to formation of the clear liquid–liquid interface. The isopropanol molecules in the poor solvent layer could be diffused into the aromatic toluene layer, resulting in the supersaturation at the interface, the formation of C60 crystal nuclei, and the following C60 crystal growth caused by the diffusion of C60 to the interfacial region. The supersaturation of C60 in the alcohol layer played a crucial role in the formation of C60 2D crystallite. Also, isopropanol could act as an activating agent between the toluene-based suspension of C60 and formamide-based suspension of Ni–Fe-LDH NSs for promoting the synthesis of self-assembled C60–Ni–Fe-LDH nanohybrids. After the vigorous shaking, the mixture was stored for 72 h in a refrigerator. After the completion of synthesis, the resulting product was thoroughly washed with ethanol and dried at 50 °C in an oven. To probe the effect of composition on the physicochemical properties and electrode performances of the C60–Ni–Fe-LDH nanohybrids, several molar ratios of C60
:
Ni–Fe-LDH = 1
:
3, 1
:
4, and 1
:
5 were employed, which correspond to the carbon contents of 73, 66, and 61 wt%, respectively (the obtained materials are denoted as CNF3, CNF4, and CNF5, respectively).
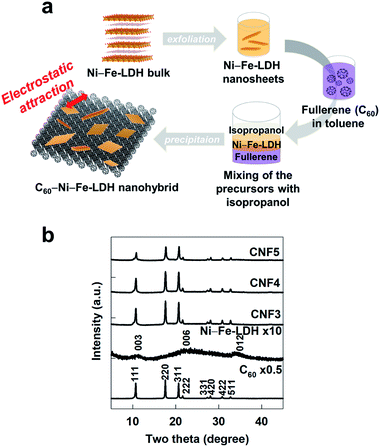 |
| Fig. 1 (a) Schematic diagram for the synthesis of the CNF nanohybrid. (b) Powder XRD patterns of CNF3, CNF4, CNF5, the pristine Ni–Fe-LDH, and C60. | |
Characterization
The zeta potentials of the colloidal suspensions of Ni–Fe-LDH and C60 were measured using a Malvern Zetasizer Nano ZS (Malvern, UK) instrument. The zeta potential was calculated from electrophoretic mobility according to the Smoluchowski approximation. The crystal structures of the CNF nanohybrids were probed with powder X-ray diffraction (XRD) analysis (Rigaku D/Max-2000/PC, Ni-filtered Cu Kα radiation, λ = 1.5418 Å, 25 °C). Micro-Raman spectra were collected with a Horiba Jobin Yvon LabRam Aramis spectrometer, in which an Ar-ion laser beam (λ = 514.5 nm) was used as an excitation source. The crystal morphologies and hybrid structures of the CNF nanohybrids were examined with high resolution-transmission electron microscopy (HR-TEM) analysis using a Jeol JEM-2100F electron microscope (200 kV) and field emission scanning electron microscopy (FE-SEM) analysis using a Jeol JSM-6700F electron microscopy. The chemical compositions and elemental distributions of the CNF nanohybrids were probed with SEM-energy dispersive X-ray spectroscopy (EDS) mapping analysis. The chemical compositions of the present materials were evaluated with an elemental analyzer (PerkinElmer 2400 Series II). The chemical bonding natures of the CNF nanohybrids were examined with Fourier transformed-infrared (FT-IR) spectroscopy. All the X-ray absorption near-edge structure (XANES) spectra at the Ni K-edge and Fe K-edge were measured at beam line 10C of the Pohang Accelerator Laboratory (PAL, Pohang, Korea). All the obtained XANES spectra were energy-calibrated by simultaneous measurement of Ni or Fe metal foil. The X-ray photoelectron spectra (XPS) were collected using a XPS machine (Thermo VG, UK, Al Kα). The internal charge transfer in the present materials was studied with photoluminescence (PL) spectroscopy using a PerkinElmer LS55 fluorescence spectrometer. The surface areas and pore structures of CNF nanohybrids were examined with N2 adsorption–desorption isotherm measurements at 77 K using a Micromeritics ASAP 2020.
Electrochemical measurement
The supercapacitor electrode functionalities of the CNF nanohybrids were studied by measuring the cyclic voltammetry (CV) and galvanostatic charge–discharge (CD) cycle data. All the electrochemical data were measured with a conventional three-electrode cell using a potentiostat/galvanostat (WonA Tech). The working electrodes were fabricated by mixing the active CNF nanohybrids, acetylene black, and polyvinylidene fluoride (PVDF) in a mass ratio of 75
:
15
:
10. The homogeneous mixing was achieved by stirring for 1 h in N-methyl-2-pyrrolidone. The obtained slurry was deposited on a Ni foam (2.5 mg cm−2) and then dried at 80 °C for 2 h. A Pt mesh was used as the counter electrode, whereas a saturated calomel electrode (SCE) was employed as the reference electrode. 1.0 M aqueous KOH solution was used as an electrolyte. The CV data were measured in the potential region of 0.0–0.55 V (V vs. SCE) at variable scan rates from 10 to 80 mV s−1. The galvanostatic CD data were collected at a constant current density of 1 A g−1. The electrochemical impedance spectroscopy (EIS) data were measured in the frequency range of 0.01–105 Hz using an IVIUM analyzer at the open circuit potential.
Results and discussion
Powder XRD, micro-Raman, elemental analysis, HR-TEM, FE-SEM, EDS-elemental mapping, and FT-IR analyses
As plotted in powder XRD patterns of Fig. 1b, the precursor C60 displays well-defined Bragg reflections of the face-centered cubic close packing lattice of fullerene, whereas the typical XRD peaks of the LDH phase are discernible for the pristine Ni–Fe-LDH.25,26 The intensities of Bragg reflections are much weaker for the pristine Ni–Fe-LDH than for the pristine C60, indicating the lower crystallinity of the LDH component than fullerene. All the present CNF nanohybrids commonly show well-defined Bragg reflections of C60 but no peaks of the pristine LDH phase. No clear observation of the LDH-related peaks for the CNF nanohybrids is attributable to the homogeneous dispersion of exfoliated LDH nanosheets without any phase separation as well as to its poor crystallinity. The least-squares fitting analysis clearly demonstrates only negligible alterations of the lattice parameters of fullerene upon the hybridization with LDH NSs (Table S1†), indicating the good maintenance of the fullerene lattice in the resulting nanohybrids. Similar to the XRD results, the CNF nanohybrids show distinct Raman spectral features of C60 but no distinct phonon lines of Ni–Fe-LDH, see Fig. S3.† This result can be ascribed to the low content and poor crystallinity of the Ni–Fe-LDH component in the present nanohybrids.
The nanoscale hybridization between fullerene NSs and LDH NSs is obviously evidenced by TEM analysis. Like the exfoliated Ni–Fe-LDH NSs, fullerene also forms highly anisotropic 2D crystallites in the mixed media of toluene and isopropanol, see Fig. S4.† Such a formation of 2D NS crystallites is attributable to the assembly of C60 molecules caused by the van-der-Waals-type intermolecular interaction in the liquid–liquid interfacial region. The present CNF4 nanohybrid displays a clear TEM image of intimately-coupled hybrid structures of C60 and Ni–Fe-LDH NSs (Fig. 2a), which is further confirmed by FE-SEM analysis with different magnifications (Fig. S5†). Similar TEM images are also observed for the other CNF3 and CNF5 nanohybrids (Fig. S6†), confirming the formation of intimate nanohybrid materials with different compositions. The formation of the nanointerface between C60 and Ni–Fe-LDH domains is obviously evidenced by the HR-TEM image showing distinct lattice fringes of both the components (Fig. 2a). The selected area electron diffraction (SAED) analysis cross-confirms the intimate hybridization of both C60 and Ni–Fe-LDH components, see Fig. 2b. The EDS-elemental mapping analysis provides strong evidence for the homogeneous hybridization of LDH NSs with C60 NSs, see Fig. 2c. The CNF4 nanohybrid shows a uniform distribution of carbon, nickel, and iron elements in the entire sections of this material, confirming the homogeneous coupling between C60 and Ni–Fe-LDH. According to the EDS analysis, all the present CNF nanohybrids commonly have a Ni/Fe ratio of ∼2/1, which is almost the same as the Ni/Fe ratios of the pristine Ni–Fe-LDH (∼2.0), indicating the negligible effect of hybridization on the chemical composition of Ni–Fe-LDH. The concentration of fullerene in the present materials is estimated with CHNS elemental analysis; the molar ratio of C60/Ni–Fe-LDH is determined to be 1/2.7 for CNF3, 1/3.4 for CNF4, and 1/4.6 for CNF5, which correspond to the carbon contents of 75, 69, and 63 wt% for CNF3, CNF4, and CNF5, respectively. This result is in good agreement with the initial ratio of reactants in the precursors. The co-existence of fullerene and LDH in the present CNF nanohybrids is further verified by FT-IR spectroscopy. As plotted in Fig. 2d, the reference C60 exhibits strong IR bands corresponding to the carbon–carbon stretching modes at ∼520, ∼570, ∼1180, and ∼1430 cm−1.27 Intense nitrate-related IR bands appear at ∼670 and ∼1380 cm−1 and broad OH−-related bands are discernible at ∼1500–1700 cm−1 for the pristine Ni–Fe-LDH. All the present CNF nanohybrids demonstrate distinct IR bands of the C60 phase and weaker bands related to nitrate and hydroxyl components of Ni–Fe-LDH, emphasizing the homogeneous hybridization between C60 NSs and Ni–Fe-LDH NSs.
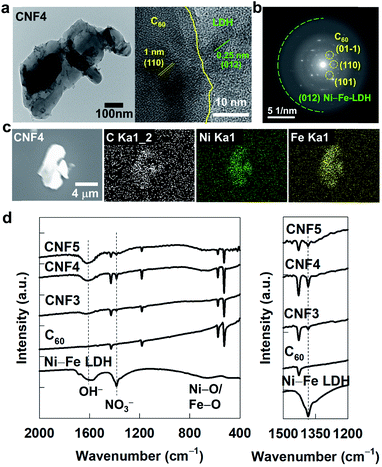 |
| Fig. 2 (a) TEM images, (b) SAED pattern and (c) EDS-elemental maps of CNF4. (d) FT-IR spectra of CNF3, CNF4, CNF5, the pristine Ni–Fe-LDH, and C60. | |
XANES, XPS, and PL analyses
The effect of fullerene hybridization on the electronic and local crystal structures of Ni–Fe-LDH is investigated with the Ni K-edge and Fe K-edge XANES spectroscopic analyses. As plotted in Fig. 3a, the Ni K-edge XANES spectral features of the present CNF nanohybrids are quite similar to that of the pristine Ni–Fe-LDH, highlighting the existence of Ni–Fe-LDH NSs in these materials. As can be seen clearly from the right panel of Fig. 3a, the CNF nanohybrids possess higher edge energies than the pristine Ni–Fe-LDH, clearly demonstrating the increase of the Ni oxidation state upon the hybridization with fullerene. All the materials under investigation exhibit a weak pre-edge peak P related to the dipole-forbidden 1s → 3d transition at ∼8336 eV, indicating the stabilization of nickel ions in the centrosymmetric octahedral sites of the LDH lattice.28 In the main-edge region, there are several peaks A and B corresponding to the dipole-allowed 1s → 4p transitions.29 The shapes of these features A and B are almost identical to the CNF nanohybrids and the pristine Ni–Fe-LDH, confirming the stabilization of Ni–Fe-LDH in the present nanohybrids.
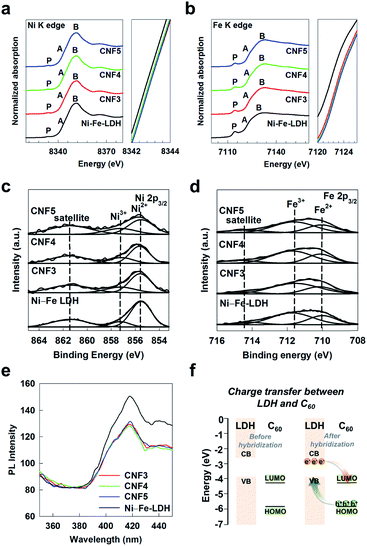 |
| Fig. 3 (a) Ni K-edge XANES spectra, (b) Fe K-edge XANES spectra, (c) Ni 2p3/2 XPS data, (d) Fe 2p3/2 XPS data, and (e) PL spectra of Ni–Fe-LDH, CNF3, CNF4, and CNF5. (f) Schematic diagram for interfacial charge transfer in the CNF nanohybrid. | |
The Fe K-edge XANES spectra of the present materials are illustrated in Fig. 3b. All the CNF nanohybrids commonly display a nearly identical spectral feature to the pristine Ni–Fe-LDH, confirming the incorporation of Ni–Fe-LDH in the present nanohybrids. Like Ni K-edge XANES data, the hybridization with fullerene displaces the Fe K-edge energy of the pristine Ni–Fe-LDH toward the higher energy side (right panel of Fig. 3b), emphasizing the electron transfer from Ni–Fe-LDH to electron-withdrawing C60. Almost the same spectral features of the pre-edge peak P and main-edge peaks A and B are discernible for the CNF nanohybrids and the pristine Ni–Fe-LDH, verifying the incorporation of Ni–Fe-LDH in the present nanohybrids.30 All the present XANES results provide strong evidence for the incorporation of Ni–Fe-LDH NSs in the present CNF nanohybrids and the occurrence of significant interfacial electron transfer with fullerene.
The evolutions of Ni and Fe oxidation states upon hybridization are quantitatively determined with peak convolution analysis for Ni 2p and Fe 2p XPS data. As plotted in Fig. 3c, the Ni 2p3/2 XPS features of the present CNF nanohybrids and the pristine Ni–Fe-LDH can be well-reproduced with Ni2+, Ni3+, and satellite peaks related to shakedown processes.31 As listed in Table S2,† the hybridization with C60 gives rise to the increase of Ni3+ content, clearly demonstrating the oxidation of Ni ions caused by interfacial charge transfer. Similarly, according to the Fe 2p XPS results in Fig. 3d and Table S2,† the relative concentration of Fe3+ species becomes greater for the CNF nanohybrids than for the pristine Ni–Fe-LDH, demonstrating the increase of the Fe oxidation state upon hybridization with C60. Also, the chemical bonding character of carbon species in the present CNF nanohybrids is examined with C 1s XPS analysis. As shown in Fig. S7,† all the materials under investigation exhibit a similar C 1s spectral feature consisting of three components at ∼284.8, ∼285.8, and ∼286.5 eV, which correspond to the the C–C bond, oxygen-related bond, and satellite peak, respectively.32 According to curve-fitting analysis, the proportion of a satellite peak related to the π–π* shakeup transition becomes larger with increasing the Ni–Fe-LDH content; 2.8, 3.6, 4.8, and 6.2% for C60, CNF3, CNF4, and CNF5, respectively. Since the spectral weight for the π–π* shakeup transition can provide a sensitive measure for the degree of the covalency of carbon species,33 the observed intensity increase of this component with increasing the LDH content strongly suggests the enhanced covalent interaction between carbon elements in the C60 component of the CNF nanohybrid. The electron injection from Ni–Fe-LDH into the C π(2p) bonding orbital of C60 leads to the increase of the bond order and the enhancement of bond covalency (Fig. 3f). All the XPS and XANES results presented here highlight the increase of average Ni and Fe oxidation states upon the hybridization with fullerene via the interfacial charge transfer. The occurrence of significant interfacial charge transfer between Ni–Fe-LDH and C60 is further evidenced by PL spectroscopy. As can be seen clearly from Fig. 3e, the PL intensities of the CNF nanohybrids are weaker than that of the pristine Ni–Fe-LDH. Such a decrease in PL intensity can be regarded as evidence for the significant electron transfer between Ni–Fe-LDH and C60, confirming the strong electronic coupling between the two components. As illustrated in Fig. 3f, the observed interfacial electron transfer between C60 and Ni–Fe-LDH can be understood in terms of relative energies of band positions. Since the valence band of Ni–Fe-LDH possesses a higher energy than the empty LUMO level of the C60 molecule, an efficient electron transfer can occur from Ni–Fe-LDH to C60.
N2 adsorption–desorption isotherm analysis
The pore structures and surface areas of the CNF nanohybrids are examined with N2 adsorption–desorption isotherm measurements. As plotted in Fig. 4a, only negligible N2 adsorptions commonly appear for the pristine Ni–Fe-LDH and C60. Conversely, all the present CNF nanohybrids display efficient N2 adsorption with similar isotherm behaviour with a weak hysteresis, which can be classified as Brunauer–Deming–Deming–Teller (BDDT)-type IV isotherms and H3-type hysteresis according to the IUPAC classification.34 This observation reflects the formation of the aggregates of plate-shaped crystallites with slit-shaped mesopores upon the hybridization, as observed for any other 2D NS-based hybrid materials.35 From the fitting analysis based on the Brunauer–Emmett–Teller (BET) equation, the surface areas of CNF3, CNF4, CNF5, Ni–Fe-LDH, and C60 are determined to be 16.5, 36.9, 44.1, 1.4, and 1.3 m2 g−1, respectively. It is worthwhile to note that, despite very low surface areas of the pristine C60 and Ni–Fe-LDH, the CNF nanohybrids have much larger surface areas, underscoring the beneficial effect of hybridization on the porosity. This result can be ascribed to the optimization of the open stacking structure by the hybridization with fullerene NSs. The pore size calculation using the Barrett–Joyner–Halenda (BJH) equation clearly demonstrates the presence of mesopores with the average size of ∼3–4 nm in the present CNF nanohybrids, see Fig. 4b. The pore volumes of the present materials are determined as 0.040, 0.073, 0.085, 0.0045, and 0.0027 cm3 g−1 for CNF3, CNF4, CNF5, Ni–Fe-LDH, and C60, respectively. This result emphasizes that the enhancement of the surface area upon the hybridization originates mainly from the increase of pore volume.
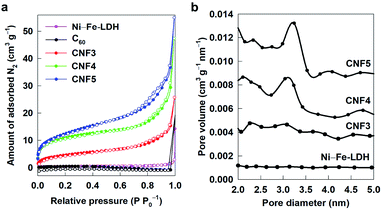 |
| Fig. 4 (a) N2 adsorption–desorption isotherms and (b) pore size distribution curves of CNF3, CNF4, CNF5, Ni–Fe-LDH, and C60. | |
Electrochemical measurements
All the present CNF nanohybrids are applied as electrode materials for the supercapacitor to study the effect of fullerene addition on the electrode functionality of Ni–Fe-LDH. Fig. 5a–c and S8† present CV curves of the CNF nanohybrids as well as the pristine Ni–Fe-LDH and C60, which are measured at a scan rate of 10 mV s−1 with a potential range of 0–0.55 V (V vs. SCE) in the 1.0 M KOH electrolyte. All the present materials show pseudocapacitance-type CV curves with several redox peaks. A set of redox peaks are discernible at ∼0.50 and ∼0.40/0.30 V for the pristine Ni–Fe-LDH, which are assigned as Ni2+/Ni3+ and Fe2+/Fe3+ couples, respectively. The pristine C60 shows distinct peaks at ∼0.48 and ∼0.38/0.30 V corresponding to the redox process of fullerene, highlighting its unique high activity for redox reactions as electrode for the supercapacitor. This is in stark contrast to the other carbon-based materials like CNT and graphene showing no redox activity.36,37 All the CNF nanohybrids commonly show several peaks corresponding to the redox processes of C60 and Ni–Fe-LDH in their CV curves, indicating the contributions of both components to the specific capacitance of the present nanohybrids. This finding strongly suggests that the faradaic process of fullerene as well as LDH makes a significant contribution to the specific capacitance of the CNF nanohybrid. One thing of prime importance is that the hybridization with fullerene significantly increases the area of the CV curve, indicating the enhancement of the specific capacitances of hybridized components due to their synergistic coupling. The extended electrochemical cycling for the CNF nanohybrids causes notable shifts of anodic and cathodic peaks to the negative and positive potential regions, respectively. This observation is attributable to the effective amplification of current upon coupling with highly-conductive fullerene.38 As plotted in the specific capacitance plots of Fig. 5d, these nanohybrids deliver much greater specific capacitances than both the precursors of C60 and Ni–Fe-LDH NSs, underscoring the beneficial effect of hybridization. A significant increase of specific capacitance proceeding the cycles is commonly observed for all the CNF nanohybrids. This observation can be ascribed to the activation process of electrode materials and the increased electroactive surface area during the cycle process.39–41 A similar gradual increase of capacitance was reported for many other LDH-based supercapacitor materials.39–41 Among the present nanohybrids, CNF4 delivers the largest specific capacitance of 1299 F g−1. Beyond the optimal C60/Ni–Fe-LDH ratio of 0.25, a further increase of the C60 content to 0.33 (CNF3) decreases the specific capacitance of the nanohybrid, which is attributable to the accompanying lowering of the surface area and the depression of the hybridization effect.42 This result confirms that the CNF4 nanohybrid possesses an optimal ratio of C60/Ni–Fe-LDH for electrode application. As plotted in Fig. 5e, the incorporation of fullerene improves the electrode performance of Ni–Fe-LDH at all the scan rates employed, highlighting the validity of the present hybridization strategy with fullerene in improving the electrode performance of the LDH material. A depressed dense stacking of LDH NSs caused by the intimate mixing with fullerene NSs enhances the accessibility of ions to adsorption sites even under high scan rate conditions. The beneficial effect of fullerene hybridization on the electrode performance of LDH is cross-confirmed by galvanostatic CD cycling measurements. As depicted in Fig. 5f, all the CNF nanohybrids demonstrate similar CD curves with some curvature, as observed for the precursor C60 and Ni–Fe-LDH. Like the CV results, amongst the CNF nanohybrids, CNF4 delivers the largest specific capacitance of 2697 F g−1 at a current density of 1 A g−1 for the 2500th cycle, see Fig. S9.† To the best of our knowledge, the observed specific capacitance of the CNF4 nanohybrid is one of the largest capacitances for Ni–Fe-LDH-based materials ever-reported (Table S3†). Additionally, to probe the influence of nanoscale hybridization on the electrode performance of the CNF nanohybrid, the physical mixture of C60 and Ni–Fe-LDH in a molar ratio of 1
:
4 is synthesized and tested as the supercapacitor electrode. As can be seen clearly from Fig. S10,† the physical mixture delivers a much smaller capacitance of 490 F g−1 than the CNF4 nanohybrid (1299 F g−1), demonstrating the crucial role of nanoscale mixing between C60 and LDH NSs. This result underscores the advantage of nanoscale hybridization with C60 in exploring efficient electrode materials.
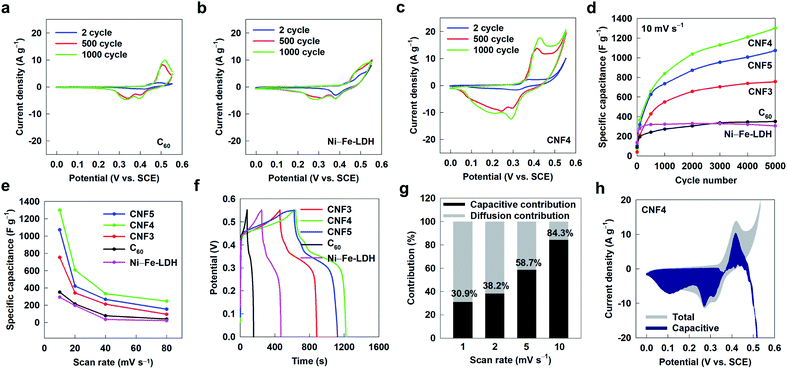 |
| Fig. 5 (a, b and c) CV curves, (d) specific capacitance plots at a scan rate of 10 mV s−1, (e) rate-dependent capacitance plots, and (f) CD curves of CNF3, CNF4, CNF5, pristine Ni–Fe-LDH, and C60. (g) The fraction of the capacitive and diffusion-controlled contributions of the CNF4 electrode at various scan rates. (h) Capacitive contribution curve of CNF4 at a scan rate of 10 mV s−1. | |
To further investigate the effect of hybridization on the electrochemical kinetics, the capacitive contributions of CNF4, C60, and Ni–Fe-LDH at different scan rates are calculated using the following equation: i(V) = k1v + k2v1/2. In this equation, the total specific capacitance contains both capacitive contribution (k1v) and diffusion contribution (k2v1/2).43 The proportion of the capacitive distribution becomes larger with increasing the scan rate, as presented in Fig. 5g, h, and S11.† At a scan rate of 10 mV s−1, the maximum percentage of the capacitive distribution is determined as 84.3%, 54.8%, and 34.2% for CNF4, C60, and Ni–Fe-LDH, respectively. Such a larger contribution of the capacitive-dominant reaction for CNF4 than for C60 and Ni–Fe-LDH is attributable to the faster charge transfer kinetics and larger surface area of the hybrid electrode. The observed remarkable capacitive contribution of CNF4 reflects its excellent rate performance for the supercapacitor, verifying the fast redox pseudocapacitive reaction in this material.
The effect of fullerene incorporation on the transport property of Ni–Fe-LDH is investigated with EIS analysis. As depicted in Fig. 6a, the present CNF nanohybrids commonly display a semicircle at the high frequency region and a straight line at the low frequency region. The hybridization with fullerene causes a notable decrease in the radius of the semicircle in the EIS spectra, indicating the improvement of charge transfer kinetics upon the incorporation of conductive C60. Among the present nanohybrids, the CNF3 nanohybrid exhibits the smallest radius of the semicircle. From the curve fitting analysis in Fig. 6a, smaller charge transfer resistances (Rct) of 53.4, 69.1, and 84.5 Ω occur for CNF3, CNF4, and CNF5, respectively, compared with that of the pristine Ni–Fe-LDH (140.2 Ω), indicating their excellent charge transfer properties. Also, all the CNF nanohybrids commonly display steeper slopes of the straight line than the pristine Ni–Fe-LDH, confirming the enhancement of electrical conductivity upon the incorporation of fullerene. The present EIS result is attributable to the optimization of the pore structure caused by the intimate hybridization between C60 and LDH NSs, leading to the facilitation of ion diffusion into the nanohybrid lattice. To obtain quantitative information about the charge transfer properties of the CNF4 nanohybrid before and after the electrochemical cycling, curve fitting analysis is carried out for the present Nyquist plots using the equivalent circuit (Fig. S12†). According to the fitting analysis, the charge transfer resistance (Rct) is determined to be 69.1 Ω for the as-prepared CNF4 nanohybrid and 45.1 Ω for its 5000-cycled derivative, clearly demonstrating the significant improvement of charge transfer kinetics upon the extended cycling. Such an improved charge transfer property makes an additional contribution to the gradual increase of specific capacitance up to the 5000th cycle (Fig. 5d). The slope of the real part of the impedance data is plotted in Fig. 6b as a function of the inverse square root of the angular frequency in the Warburg region to determine the diffusion coefficient. The Warburg coefficient is calculated from the Warburg impedance (Zw) using the following equation: Zω = (1 − j)σω−1/2, where σ is the Warburg coefficient and ω is the angular frequency. The Warburg coefficient (σ) value can be estimated from the slope of the linear plot of the real (Z′) or imaginary (Z′′) part against the angular frequency (ω−1/2). The Warburg coefficient can be evaluated by the following equation: σ = RT(nF)−2(AC*)−1(2D)−1/2, where R is the gas content, T is the absolute temperature, n is the charge-transfer number, F is the Faraday constant, A is the area of the electrode surface, C* is the electrolyte concentration, and D is the diffusion coefficient. As can be seen from this equation, the Warburg coefficient is inversely proportional to the diffusion coefficient, indicating that the smaller Warburg coefficient implies more efficient ion diffusion taking part in the redox reactions across the electrode–electrolyte interface. The Warburg coefficients of the present materials are calculated to be 72.9 Ω s−1/2 for Ni–Fe-LDH, 21.4 Ω s−1/2 for C60, 43.9 Ω s−1/2 for CNF3, 28.9 Ω s−1/2 for CNF4, and 51.5 Ω s−1/2 for CNF5, respectively. Among the present nanohybrids, the CNF4 nanohybrid possesses the smallest Warburg coefficient, indicating the excellent ion diffusion property of these materials due to the creation of the wide-open pore structure.
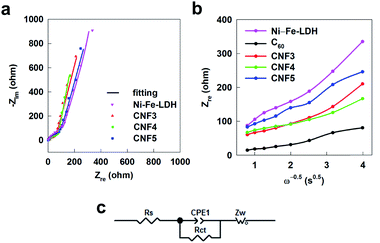 |
| Fig. 6 (a) Nyquist plots and (b) Zrevs. ω−0.5 of CNF3, CNF4, CNF5, the pristine Ni–Fe-LDH, and C60. (c) Equivalent circuit employed for the fitting analysis of EIS data. | |
Summarizing all the experimental findings presented here, the CNF4 nanohybrid shows the best supercapacitor performance among the present CNF nanohybrids. This finding can be explained in terms of the following factors: (1) the intimate hybridization between Ni–Fe-LDH and C60 is effective in enhancing the porosity of the stacking structure via the depression of the self-stacking of precursor NSs. Taking into account the higher content of C60 than Ni–Fe-LDH in the present CNF nanohybrids, an increase of the LDH content from CNF3 to CNF5 can enhance the mixing effect between LDH and C60 components via the increase of LDH/C60 proportion, leading to the enhancement of the surface area and pore diameter of the nanohybrid. (2) There occurs a significant electronic coupling between C60 and Ni–Fe-LDH, as evidenced by XANES, XPS and PL analyses. The incorporation of conductive C60 significantly lowers the charge transfer resistance (Rct) of the CNF nanohybrids compared with that of the pristine Ni–Fe-LDH, highlighting the enhancement of the charge transfer property. In fact, among the present nanohybrids, the CNF3 nanohybrid with the largest C60 content exhibits the smallest Rct value, confirming the beneficial effect of C60 incorporation on the charge transport property. Since both the factors (1) and (2) exhibit the opposite dependence on the C60/Ni–Fe-LDH ratio, the superior electrode performance of the CNF4 nanohybrid with the intermediate C60/Ni–Fe-LDH ratio over the other nanohybrids can be ascribed to a compromise of both the factors. (3) According to the Warburg coefficient calculations, the hybridization between C60 and Ni–Fe-LDH also improves the ion diffusion capability. Among the present nanohybrids, the CNF4 nanohybrid with the optimal C60/Ni–Fe-LDH ratio shows the smallest Warburg coefficient and the highest ion diffusivity. The fastest ion diffusion property of CNF4 can make an additional contribution to the best electrode performance of this material.
To compare the hybridization efficiency of fullerene with that of widely-used graphene for improving the electrode performance of metal hydroxide, the self-assembled nanohybrid of reduced graphene oxide (rGO)–Ni–Fe-LDH with the same molar concentration as CNF4 is also synthesized (this material is denoted as GNF4) and applied as the electrode for the supercapacitor. The homogeneous hybridization between rGO and Ni–Fe-LDH is confirmed by powder XRD, TEM, and EDS-mapping analyses, see Fig. 7a and S13.† As plotted in Fig. 7b, the capacitance plots measured by CV curves clearly demonstrate that the GNF4 nanohybrid shows a larger specific capacitance of 650 F g−1 than that of the pristine Ni–Fe-LDH but smaller than that of the CNF4 nanohybrid. Also, as shown in the CD data of Fig. 7c, the electrode performance of the GNF4 nanohybrid is poorer than that of CNF4, highlighting the better role of C60 than graphene in improving the electrode performance of LDH. According to the EIS analysis in Fig. 7d, the GNF4 nanohybrid exhibits a higher charge transfer resistance (Rct) of 80.3 Ω than that of CNF4 (69.1 Ω), demonstrating a higher efficiency of hybridization with C60 in improving the charge transfer kinetics of LDH compared with that with rGO. The superior efficacy of fullerene as a hybridization matrix over rGO can be ascribed to its better charge transfer ability, its significant redox activity, and its stronger interfacial electronic coupling with LDH NSs.
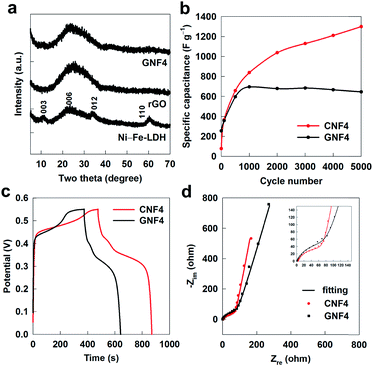 |
| Fig. 7 (a) Powder XRD patterns of the Ni–Fe-LDH, rGO and GNF4 nanohybrid. (b) Specific capacitance plots, (c) CD curves and (d) Nyquist plots of the CNF4 and GNF4 nanohybrids. | |
Conclusions
In this report, an effective way to remarkably improve the electrode performance of LDH is developed by employing fullerene as a hybridization matrix. The obtained intimately-coupled nanohybrids of C60–Ni–Fe-LDH show much greater specific capacitance with improved rate characteristics than the pristine Ni–Fe-LDH, underscoring the beneficial effect of fullerene incorporation on the supercapacitor electrode performance of LDH. Among the nanohybrids synthesized in this study, the CNF4 nanohybrid with an appropriate C60/Ni–Fe-LDH ratio of 1/4 delivers the largest capacitance of 1299 F g−1 (at a scan rate of 10 mV s−1)/2697 F g−1 (at a current density of 1 A g−1), which is 3 times larger than that of the pristine Ni–Fe-LDH. This is one of the largest specific capacitances of Ni–Fe-LDH-based electrode materials. The beneficial effect of fullerene hybridization on the electrode performance is attributable to the enhancement of the porosity of LDH, the significant contribution of the redox process of fullerene, and the enhancement of charge transport kinetics and surface electrophilicity. The present study highlights the remarkable effectiveness of C60 as a nanocarbon additive for exploring highly efficient LDH-based electrode materials. It is worthwhile to mention that the efficiency of fullerene as a hybridization matrix is much superior to that of widely-used graphene, confirming the validity of the present synthetic strategy for synthesizing efficient hybrid electrode materials. Since there are many application of the LDH material as electrodes, photocatalysts, and electrocatalysts,12–14 the hybridization with fullerene can provide an effective synthetic methodology to novel LDH-based functional materials. Judging from the 2D NS morphology and high electrical conductivity of self-assembled fullerene nanosheets, this 2D species can be universally used as an efficient conductive hybridization matrix for a variety of inorganic solids such as metal, metal oxide, metal chalcogenide, and metal phosphide, as reported for graphene NSs.44–47 Thus, the present synthetic strategy with assembled fullerene NSs can open a new chapter for the development of a novel type of nanocarbon-based functional nanohybrids. Our current project is employing the present hybridization strategy to diverse couples of functional inorganic nanostructures for exploiting novel multifunctional nanohybrids with improved performances.
Conflicts of interest
There are no conflicts to declare.
Acknowledgements
This work was supported by the National Research Foundation of Korea (NRF) grant funded by the Korea government (MSIP) (No. NRF-2017R1A2A1A17069463) and by the Korea government (MSIT) (No. NRF-2017R1A5A1015365). The experiments at PAL were supported in part by MOST and POSTECH.
References
- X. Jin, S.-J. Shin, J. Kim, N.-S. Lee, H. Kim and S.-J. Hwang, J. Mater. Chem. A, 2018, 6, 15237 RSC.
- H. Jin, C. Guo, X. Liu, J. Liu, A. Vasileff, Y. Jiao, Y. Zheng and S.-Z. Qiao, Chem. Rev., 2018, 118, 6337 CrossRef CAS PubMed.
- D. Matochova, M. Medved, A. Bakandritsos, T. Stekly, R. Zbiril and M. Otyepka, J. Phys. Chem. Lett., 2018, 9, 3580 CrossRef CAS PubMed.
- Q. Zheng, R. Xie, L. Fang, Z. Cai and Z. Ma, J. Mater. Chem. A, 2018, 6, 24407 RSC.
- Y. Fang, X. Xu, Y. Du, X. Zhu, X. Zhou and J. Bao, J. Mater. Chem. A, 2018, 6, 11244 RSC.
- M. S. Javed, N. Shaheen, S. Hussain, J. Li, S. S. A. Shah, Y. Abbas, M. A. Ahmad, R. Raza and W. Mai, J. Mater. Chem. A, 2019, 7, 946 RSC.
- X. Xu, Z. Dou, E. Gu, L. Si, X. Zhou and J. Bao, J. Mater. Chem. A, 2017, 5, 13411 RSC.
- E. Gu, S. Liu, Z. Zhang, Y. Fang, X. Zhou and J. Bao, J. Alloys Compd., 2018, 767, 131 CrossRef CAS.
- D. Zhou, Z. Cai, X. Lei, W. Tian, Y. Bi, Y. Jia, N. Han, T. Gao, Q. Zhang, Y. Kuang, J. Pan, X. Sun and X. Duan, Adv. Energy Mater., 2018, 8, 1701905 CrossRef.
- J. Zhao, J. Chen, S. Xu, M. Shao, Q. Zhang, F. Wei, J. Ma, M. Wei, D. G. Evans and X. Duan, Adv. Funct. Mater., 2014, 24, 2938 CrossRef CAS.
- P. Xiong, R. Ma, N. Sakai, L. Nurdiwijayanto and T. Sasaki, ACS Energy Lett., 2018, 3, 997 CrossRef CAS.
- J.-Y. Hwang, H. M. Kim, S. Shin and Y.-K. Sun, Adv. Funct. Mater., 2018, 28, 1704294 CrossRef.
- N. H. Kwon, M. Kim, X. Jin, J. Lim, I. Y. Kim, N.-S. Lee, H. Kim and S.-J. Hwang, NPG Asia Mater., 2018, 10, 659 CrossRef CAS.
- J. L. Gunjakar, I. Y. Kim, J. M. Lee, N.-S. Lee and S.-J. Hwang, Energy Environ. Sci., 2013, 6, 1008 RSC.
- R. N. Goyal, V. K. Gupta and N. Bachheti, Anal. Chim. Acta, 2007, 597, 82 CrossRef CAS PubMed.
- L. Chen, M. Wu, G. Shao, J. Hu, G. He, T. Bu, J.-P. Yi and J. Xia, R. Soc. Open Sci., 2018, 5, 172041 CrossRef PubMed.
- J. Fried, M. A. Lebedeva, K. Porfyrakis, U. Stimming and T. W. Chamberlain, J. Am. Chem. Soc., 2018, 140, 401 CrossRef PubMed.
- H. Imahori and Y. Sakata, Eur. J. Org. Chem., 1999, 1999, 2445 CrossRef.
- P.-M. Allemand, A. Koch, F. Wudl, Y. Rubin, F. Diederich, M. M. Alvarez, S. J. Anz and R. L. Whetten, J. Am. Chem. Soc., 1991, 113, 1050 CrossRef CAS.
- R. R. Delgado, M. A. Vidaurre, C. P. D. Pauli, M. A. Ulibarri and M. J. Avena, J. Colloid Interface Sci., 2004, 280, 431 CrossRef PubMed.
- Q. Wang and D. O'Hare, Chem. Rev., 2012, 112, 4124 CrossRef CAS PubMed.
- J. M. Lee, T. H. Gu, N. H. Kwon, S. M. Oh and S.-J. Hwang, J. Phys. Chem. C, 2016, 120, 8451 CrossRef CAS.
- J. M. Lee, J. L. Gunjakar, Y. Ham, I. Y. Kim, K. Domen and S.-J. Hwang, Chem.–Eur. J., 2014, 20, 17004 CrossRef CAS PubMed.
- M. Sathish and K. Miyazawa, J. Am. Chem. Soc., 2007, 129, 13816 CrossRef CAS PubMed.
- B. M. Ginzburg, S. Tuichiev, S. K. Tabarov, A. A. Shepelevskii and L. A. Shibaev, Tech. Phys., 2005, 50, 1458 CrossRef CAS.
- M. S. Islam, M. Kim, X. Jin, S. M. Oh, N.-S. Lee, H. Kim and S.-J. Hwang, ACS Energy Lett., 2018, 3, 952 CrossRef CAS.
- H. I. Elim, R. Anandakathir, R. Jakubiak, L. Y. Chiang, W. Ji and L.-S. Tan, J. Mater. Chem., 2007, 17, 1826 RSC.
- M.-J. Paek, T. W. Kim and S.-J. Hwang, J. Phys. Chem. Solids, 2008, 69, 1444 CrossRef CAS.
- S.-J. Hwang, C.-W. Kwon, J. Portier, G. Campet, H.-S. Park, J.-H. Choy, P. V. Huong, M. Yoshimura and M. Kakihana, J. Phys. Chem. B, 2002, 106, 4053 CrossRef CAS.
- M. A. Woo, T. W. Kim, I. Y. Kim and S.-J. Hwang, Solid State Ionics, 2011, 182, 91 CrossRef CAS.
- Y. Wang, M. Qiao, Y. Li and S. Wang, Small, 2018, 14, 1800136 CrossRef PubMed.
- D. García, L. Rodríguez-Pérez, M. A. Herranz, D. Peña, E. Guitián, S. Bailey, Q. Al-Galiby, M. Noori, C. J. Lambert, D. Pérez and N. Martín, Chem. Commun., 2016, 52, 6677 RSC.
- A. Kuzume, E. Herrero, J. M. Feliu, E. Ahlberg, R. J. Nichols and D. J. Schiffrin, Phys. Chem. Chem. Phys., 2005, 7, 1293 RSC.
-
T. Allen, Particle Size Measurements, Chapman and Hall, London, 4th edn, 1980 Search PubMed.
- T.-H. Gu, J. L. Gunjakar, I. Y. Kim, S. B. Patil, J. M. Lee, X. Jin, N.-S. Lee and S.-J. Hwang, Small, 2015, 11, 3921 CrossRef CAS PubMed.
- Y. Zhang, B. Lin, Y. Sun, X. Zhang, H. Yang and J. Wang, RSC Adv., 2015, 5, 58100 RSC.
- T. K. Shruthi, N. llayaraja, D. Jeyakumar and M. Sathish, RSC Adv., 2014, 4, 24248 RSC.
- H. Ma, J. He, D.-B. Xiong, J. Wu, Q. Li, V. Dravid and Y. Zhao, ACS Appl. Mater. Interfaces, 2016, 8, 1992 CrossRef CAS PubMed.
- D. Su, Z. Tang, J. Xie, Z. Bian, J. Zhang, D. Yang, D. Zhang, J. Wang, Y. Liu, A. Yuan and Q. Kong, Appl. Surf. Sci., 2019, 469, 487 CrossRef CAS.
- L. Liu, T. Guan, L. Fang, F. Wu, Y. Lu, H. Luo, X. Song, M. Zhou, B. Hu, D. Wei and H. Shi, J. Alloys Compd., 2018, 763, 926 CrossRef CAS.
- X. Hao, Y. Zhang, Z. Diao, H. Chen, A. Zhang and Z. Wang, RSC Adv., 2014, 4, 63901 RSC.
- X. Jin, S.-J. Shin, N. Kim, B. Kang, H. Piao, J.-H. Choy, H. Kim and S.-J. Hwang, Nano Energy, 2018, 53, 841 CrossRef CAS.
- Y. Liu, X.-Y. Yu, Y. Fang, X. Zhu, J. Bao, X. Zhou and X. W. Lou, Joule, 2018, 2, 725 CrossRef CAS.
- A. Abdelhafix, A. Vitale, P. Buntin, B. deGlee, C. Joiner, A. Robertson, E. M. Vogel, J. Warner and F. M. Alamgir, Energy Environ. Sci., 2018, 11, 1610 RSC.
- B. Park, S. M. Oh, X. Jin, K. Adpakpang, N.-S. Lee and S.-J. Hwang, Chem.–Eur. J., 2017, 23, 6544 CrossRef CAS PubMed.
- S. Lee, X. Jin, I. Y. Kim, T.-H. Gu, J.-W. Choi, S. Nahm and S.-J. Hwang, J. Phys. Chem. C, 2016, 120, 11786 CrossRef CAS.
- J. Li, M. Yan, X. Zhou, Z.-Q. Huang, Z. Xia, C.-R. Chang, Y. Ma and Y. Qu, Adv. Funct. Mater., 2016, 26, 6785 CrossRef CAS.
Footnote |
† Electronic supplementary information (ESI) available: Photoimages, zeta potentials, and TEM data of exfoliated Ni–Fe-LDH NSs and C60, lattice parameters, micro-Raman data, FE-SEM images, C 1s, Ni 2p and Fe 2p XPS, CV curves and CD data of CNF nanohybrids, table of the specific capacitances of Ni–Fe-LDH-based electrode materials, CV curves and specific capacitance plots of the physical mixture of C60 and Ni–Fe-LDH, capacitive contribution data of C60 and Ni–Fe-LDH, Nyquist plot of the cycled CNF4 electrode. See DOI: 10.1039/c9ta01532a |
|
This journal is © The Royal Society of Chemistry 2019 |