Graphene quantum dot induced tunable growth of nanostructured MnCo2O4.5 composites for high-performance supercapacitors†
Received
1st June 2019
, Accepted 19th July 2019
First published on 22nd July 2019
Abstract
Achieving an optimal surface structure and adequate carbon distribution is an important criterion for transition metal oxide/carbon composites targeted for supercapacitor applications. In this study, a one-step hydrothermal reaction is developed to incorporate graphene quantum dots (GQDs) and MnCo2O4.5 with GQDs serving as both a conductive filling material and structure inducing agent. The process is fine-tuned based on GQD quantities to generate various morphologies including nanospheres, nanoneedles and nanoarrays. And a mechanism is proposed to explain the roles of GQDs in triggering the structural transformation. Among the different structures, the nanoneedle composites (denoted as MCO-40 GQDs) exhibit an apparent porous structure and even GQD distribution, which establishes a conductive network that provides excellent charge transfer capability for optimal electrochemical performances. As a result, the MCO-40 GQD nanoneedle electrode delivers the largest capacitance of 1625 F g−1 at 1 A g−1, which is four times higher than the capacitance of a pure MnCo2O4.5 nanosphere electrode (368 F g−1 at 1 A g−1). Moreover, the asymmetric supercapacitor fabricated with MCO-40 GQDs and reduced graphene oxide exhibits long cycle stability and a high energy density of 46 Wh kg−1 at a power density of 66 W kg−1, surpassing all previously reported capacitive devices based on MnCo2O4.5. Overall, this study illustrates the exciting roles of GQDs in material synthesis as a structure inducing agent and provides for the first time a reference for constructing MnCo2O4.5 based advanced nanomaterials with various shapes for energy storage applications.
Introduction
The research and development processes of supercapacitors for energy storage applications have always been an important part of the global effort to lower fossil fuel reliance.1 In comparison to batteries, supercapacitors provide higher power density and longer cycle life as a result of their highly reversible ion adsorption mechanism that differs from the intercalation process in lithium ion batteries.1,2 They are generally categorized into either electrochemical double-layer capacitors or pseudo-capacitors (redox supercapacitors). The former operates with a mechanism of surface ion adsorption only and is reliant on high surface area carbon materials, whereas the latter utilizes both adsorption and surface faradaic redox reactions to provide additional energy storage capacity.3–6 In recent years, complex transition metal oxides (TMOs) with rich redox reactions, synergistic effects and unique morphologies have emerged as ideal candidates to achieve maximum supercapacitor electrochemical performances.7–15 Common strategies of synthesizing TMOs with multiple metal elements include co-precipitation and foreign ion doping, while generating a desirable morphology using inducing agents and compositing with carbon materials are also repeatedly investigated to alleviate issues associated with the low surface area and low conductivity of the materials.2
Among the different complex TMOs, MnCo2O4.5 is one that has yet to realize its high theoretical potential in spite of significant research efforts through the strategies mentioned above.16–21 In light of this matter, graphene quantum dots (GQDs), a recently emerged zero-dimensional graphene based material with various interesting properties, may be the key to achieving the long waited breakthrough. One particularly useful property of GQDs is the high functional group abundancy in its surface, which is important for improving solvent dispersibility to simplify various reactions and aiding molecular interaction for electrolyte penetration.22,23 In combination with their high electrical conductivity and high surface area, GQDs have been frequently established as a coating layer to improve material performance through methods such as stirring attachment,24 electrodeposition,25 and plasma-enhanced chemical vapor deposition,26 while other studies directly incorporate GQDs into their synthetic process and utilize its capability of electrostatically absorbing ions/molecules to create nucleation sites and direct the crystal growing mechanism, which results in the generation of GQD-doped materials blessed with enhanced electrical conductivity.25,27,28
Given the desirable properties of GQDs, in this study, the material is explored as a conductive structure-directing agent to fine-tune the morphology of MnCo2O4.5 and increase their limited conductivity. The effects GQDs may have in the process are systematically investigated based on the morphological, crystallographic and elemental properties of the product obtained, and an optimal GQD quantity is identified. The resulting nanoneedle structured MnCo2O4.5/GQD composite prepared with the optimized GQD content demonstrates excellent electrochemical properties, achieving a specific capacitance of 1625 F g−1 at 1 A g−1 which far exceeds that of the pure MnCo2O4.5 nanosphere. The asymmetrical supercapacitor device prepared with this composite material also displays capacitance and power capability that are top-notch for supercapacitors based on MnCo2O4.5.
Experimental section
Synthesis of GQDs
All chemicals were purchased from Sigma-Aldrich Canada and used as received without any further purification. In a typical synthesis,29 2 g of solid citric acid (99.5%) is heated in a 50 mL round bottom flask within a silicone oil bath at 200 °C for 40 min to form an orange liquid. Then 20 mL of distilled deionized (DDI) water was added and the solution was dialyzed by using a 1 kDa dialysis tube membrane for 7 days to purify the GQDs. Finally, the GQD powder was extracted from the solution by freeze drying for 4 days.
Synthesis of MnCo2O4.5/GQD composites
A piece of 1 cm × 2 cm Ni foam was first cleaned with 3 M hydrochloric acid (HCl) slowly to remove the NiO layer, which was then washed with deionized water and acetone to clean off any remaining HCl. To synthesize MnCo2O4.5, 2 mmol Mn(NO3)2·4H2O (≥97.0%), 4 mmol Co(NO3)2·6H2O (98%), 24 mmol urea (99.0–100.5%) and different amounts of GQD powder (0, 10, 20, 40, 60 and 80 mg) were dissolved in a mixture of 40 mL ethanol (99%) and 40 mL deionized water and stirred for 30 min. Next, the solution was transferred into a 100 mL stainless-steel autoclave and the Ni foam was immersed into the solution. The autoclave was then sealed and heated at 90 °C for 8 h. Upon completion of the process, the precursor-loaded Ni foam was removed from the solution and washed with DDI water, dried under vacuum at 70 °C for 12 h, and then calcined in air at 250 °C for 2 h with a ramp rate of 1 °C min−1 to obtain the final product MCO-X GQDs.
Material characterization
X-ray diffraction (XRD) patterns of the samples were obtained using a Rigaku Miniflex 600 X-ray Diffractometer that contains a graphite monochromator and a Cu Kα radiation source (λ = 1.5406 Å). The surface morphology of the materials was observed with a Zeiss ULTRA plus Field Emission Scanning Electron Microscope (FESEMs). Energy dispersive X-ray spectroscopy (EDS) spectra, transmission electron microscopy (TEM) images and high resolution transmission electron microscopy (HRTEM) images of the samples were obtained using FEI TITAN 80-300 LB Transmission Electron Microscopes at the Canadian Center for Electron Microscopy (CCEM) in McMaster University. X-ray photoelectron spectra of the MnCo2O4.5 were collected using a Thermal Scientific K-Alpha X-ray Photoelectron Spectrometer System and the XPS spectra were calibrated with the C–C 1s peak at 284.5 eV. The UV-vis absorption spectra and photoluminescence of the GQDs were tested on a monochromatic microplate spectrophotometer (Genesys 10S UV-vis) and fluorescence spectrometer (PerkinElmer LS 55). The size distribution of the as-prepared GQDs was measured using Bruker atomic force microscopy (AFM) probes.
Electrochemical measurement
Cyclic voltammetry (CV), galvanostatic charge–discharge (GCD), and electrochemical impedance spectroscopy (EIS) of the as-prepared MCO-X GQD electrodes were all carried out on a computer-controlled BioLogic VMP-300 workstation. A three-electrode configuration was adapted for the CV and GCD experiments. The MCO-X GQD working electrodes have a MnCo2O4.5 mass loading of around 1.5 mg cm−2, the electrolyte is 2 M KOH, the counter electrode is platinum foil, and a Hg/HgO electrode was used as the reference electrode. CV was performed within the potential range of 0–0.6 V, and GCD was also performed within the same voltage region. The EIS scan was collected at open circuit voltage in the frequency range of 100 kHz to 10 mHz. The electrochemical performance of the MCO-40 GQD based asymmetric supercapacitor was measured in a two-electrode system at room temperature. To prepare the counter electrode, reduced graphene oxide (80 wt%), acetylene black (10 wt%) and polyvinylidene fluoride (10 wt%) were mixed in N-methyl-2-pyrrolidinone to form a slurry, which was pressed on a piece of 1 cm × 2 cm Ni foam and then dried at 80 °C overnight in a vacuum oven. The measurement also used 2 M KOH aqueous solution as the electrolyte.
The specific capacitance C (F g−1) of the electrodes is calculated by using the following equation:30
The energy density E (Wh kg−1) and power density P (W kg−1) are calculated based on the following equations:31
In the above equations, i, Δt, V, and m represent the discharge current density (A g−1), discharge time (s), potential window (V), and mass of active materials (g), respectively.
The voltammetric charges of the active materials were measured by CV for calculation using the following equation:32
The mass ratio of the positive electrode and negative electrode in the asymmetric supercapacitor is selected using the following equation derived from charge balance:32
Base on the calculation, the mass loading of rGO and MCO-40 GQDs in the asymmetric supercapacitor was selected to be 9 mg and 1.5 mg, respectively.
Results and discussion
To begin, the GQDs were prepared with a previously reported one-step process and was purified by dialysis.29 As shown in their atomic force microscopy (AFM) analysis, the sizes of the as-prepared GQDs are fairly uniform and around 90% of their topographic heights are less than 2 nm (Fig. 1), which indicates that they consist of 1 to 3 graphene layers.33 The UV-vis spectrum of the GQDs shows a strong absorption at 248 nm and a weaker adsorption at 361 nm, which can be attributed to the π–π* transition of C
C and the n–π* transition of C
O, respectively (Fig. S1, ESI†).34–36 In the photoluminescence (PL) spectrum, the emission wavelength of these GQDs shows little variation when the excitation beam wavelength is changed from 320 to 400 nm in 20 nm steps, indicative of the uniformity of the samples. Finally, the as-prepared GQDs emit a blue light under the luminescence of a 365 nm UV beam, which matches reports in previous studies.29,37,38
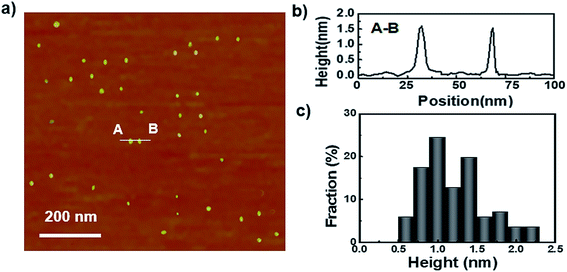 |
| Fig. 1 (a) AFM topography image of the as-prepared GQDs dropped on mica substrates and (b) height profiles of the GQD along the white line displayed in (a). (c) Height distribution of all GQDs presented in (a). | |
Upon confirming the quality of the GQDs, they were utilized in the synthesis of a bimetallic MnCo hydroxide/GQD precursor, which was further annealed to obtain the MnCo2O4.5/GQD composite (denoted as MCO-X GQDs hereafter, with X representing the amount of added GQDs). It is anticipated that the GQDs will interact with the Mn2+ and Co2+ cations and urea through surface group electrostatic attraction, hydrogen bonding and dipole–dipole interaction in the hydrothermal reaction, which alters the crystal growth mechanism and thus the morphologies of the final products.39 Schematic illustrations and scanning electron microscopy (SEM) images of MCO-X GQDs prepared with different amounts of GQDs are demonstrated in Fig. 2. As shown, when GQDs are absence, pure MCO adapts a nanosphere architecture that has diameters of around 200 nm. Upon adding 20 mg GQDs as the precursor, the morphology of the MCO/GQD composites drastically changed to interconnected nanosheets that form a porous layer on the nickel foam surface. When the amount of GQDs is raised to 40 mg, thin nanoneedles with a length of around 300 nm are found on the MCO-40 GQDs. If the amount of GQDs is further increased to 60 mg, the nanoneedles would begin to aggregate and adjust to form a nanoarray-like structure. Finally, when 80 mg of GQDs is used, the morphology of the composites returns to nanospheres but with a diameter of ∼100 nm. The thickness of the composite layer can vary from 800 nm to 1 μm as shown in the cross sectional SEM of a small section obtained from the nickel foam on which MCO-40 GQDs were grown (Fig. S2†). These results reveal the large impacts the GQD precursor has on the crystal growth process of the material. Importantly, this provides a method which can be easily manipulated to fine-tune the crystal anisotropy and material morphologies of the MCO-X GQDs for optimal electrochemical performance.
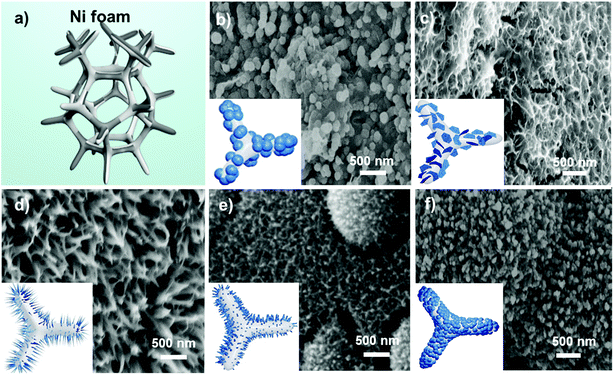 |
| Fig. 2 (a) Schematic of Ni foam and SEM images of (b) MCO, (c) MCO-20 GQDs, (d) MCO-40 GQDs, (e) MCO-60 GQDs, and (f) MCO-80 GQDs. Inset of each image shows the schematic illustration of the composite structure grown on the nickel foam. | |
The formation mechanism of the nanoparticles begins with the hydrolysis of urea to OH− and HCO3−, which react with the Mn2+ and Co3+ cations to initiate the nanoparticle nucleation following the Ostwald ripening process.40 When GQDs are absent, the growth process occurs in all directions, resulting in the formation of nanoparticles with a spherical structure. When GQDs are added, their strong electrostatic interactions with the metal ions would likely make them absorb on the surface of nuclei, and thus, change the dynamics of the process, encouraging anisotropic growth along a specific axis. For example, coordination of GQDs with the metal hydroxide can physically restrict the nanoparticle growth along the a-axis which encourages growth along b- and c-axes for the formation of nanosheets. In the same way, when more GQDs are available, the a and b axes will be covered, which promotes the growth of nanoneedles.41 However, when the GQD concentrations exceed a critical level, the excessive GQDs may simply cover the seeds completely and restrain growth in all directions. As a result, the MnCo2O4.5/GQD composites would slowly lose their anisotropy and return gradually from nanoneedles to nanoarrays and finally to their original structure of nanospheres. The lowered growth rate paired with an unchanged seed formation rate would also explain the higher abundancy and smaller sizes of MCO-80 GQDs in comparison to MCO.42
The crystal identities of the composite on different electrodes were further investigated using X-ray diffraction (XRD) spectroscopy. As shown in Fig. 3a, the addition of GQDs did not substantially impact the crystallographic properties of the end product as the XRD spectra of the MCO-X GQD composites are found to be nearly identical. All of them demonstrate peaks at 18.988°, 31.26°, 36.82°, 38.506°, 44.832°, 55.768°, 59.471° and 65.339°, which can be indexed to the (111), (220), (311), (222), (400), (422), (511) and (440) diffractions of MnCo2O4.5 (JCPDF: 32-0297). It is noted that the characteristic diffraction of graphitic carbon typically found near 25° is not detected, and this can be explained by the fact that the GQDs are very small in sizes and unlikely to form long range ordering. Of the different composites, the MCO-40 GQD composite with the nanoneedle structure is expected to provide the highest surface area desired for supercapacitor applications and is therefore selected for further examination. First, its elemental electronic configurations and composition are characterized using X-ray photoemission spectroscopy (XPS) and its survey spectrum is displayed in Fig. 3b, which confirms the presence of C, Mn, Co and O elements. In the high resolution C 1s spectrum, the absorption can be deconvoluted into four distinct peaks that represent C under different bonding conditions, which include the C
C peak at 284.8 eV, the C–OH peak at 286.4 eV, the C
O peak at 288.3 eV and the COOH peak at 289.1 eV. These functional groups available in the surfaces are what allow GQDs to influence the crystal growth mechanism and are beneficial for composite wettability (Fig. 3c).37,43 In the Mn 2p spectrum (Fig. 3d), the Mn 2p1/2 and Mn 2p3/2 peaks are found at 654.1 eV and 642.3 eV respectively; refined fitting of these two peaks shows that they are a combination of the characteristic adsorptions of Mn2+ (652.9 eV and 641.4 eV) and Mn3+ (654.1 eV and 643.3 eV).44 As for the Co 2p spectrum (Fig. 3e), two main peaks located at 795.2 eV (2p1/2) and 779.9 eV (2p3/2) are found, and each of them has a satellite peak located at a slightly higher energy level. Further deconvolution of the main peaks resulted in the intrinsic absorptions of Co with oxidation states of 2+ (797.8 eV and 782.1 eV) and 3+ (795.7 eV and 780.5 eV).44 Finally, the O 1s absorption of the composite can be divided into three major peaks, including the lattice oxygen peak (M–O–M, M represents Mn and Co) at 529.8 eV, the hydroxyl or adsorbed water oxygen peak (M–O–H) at 531.1 eV, and the peak at 530.5 eV, which confirms the formation of M–O–C bonds that interconnect GQDs and MnCo2O4.5.26 The presence of the M–O–C bonds supports the initial hypothesis regarding GQDs influencing the crystal growth mechanism and at the same time, it is beneficial to the conductivity and stability of the composite material.
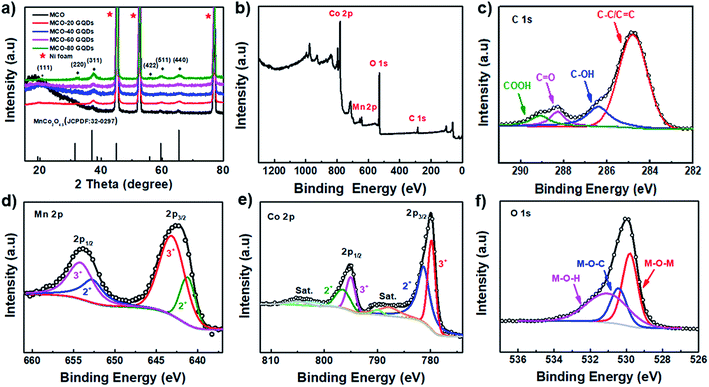 |
| Fig. 3 (a) XRD patterns of MnCo2O4.5 and MnCo2O4.5–GQD composites prepared with different GQD amounts. (b) Survey and high resolution (c) C 1s, (d) Mn 2p, (e) Co 2p and (f) O 1s XPS spectra of the porous MnCo2O4.5–40 GQDs. | |
The observation of the MCO-40 GQDs with transmission electron microscopy (TEM) largely coincides with the SEM result, showing nanoneedles with width ranging from 20 to 50 nm (Fig. 4a). The nanoneedles are also discovered to be porous and polycrystalline with varying color density across their structure. The high-resolution TEM (HRTEM) (Fig. 4b) image reveals various lattice fringes on the nanoneedles that are distinctly different. The regions surrounded by red circles demonstrate lattice patterns with a d-spacing of 0.24 nm, which corresponds to the (1120) facets of graphene quantum dots.45,46 In the region encapsulated by the black square, two sets of lattice patterns that form a 45° angle can be observed. Their lattice spacing is measured to be 0.286 nm and 0.202 nm, corresponding to the interplanar distances of (220) and (400) crystal planes that belong to MnCo2O4.5 (Fig. 4c). High-angle annular dark-field scanning transmission electron microscopy and corresponding energy-dispersive X-ray spectroscopy (EDS) mapping of MCO-40 GQDs show homogeneous distribution of Mn, Co, O and C in the material (Fig. 4d–g), which further confirms the even distribution of GQDs and MnCo2O4.5 within the MCO-40 GQD composites. Again, the presence of GQDs within the nanoneedle center is important evidence that proves their role in tuning the composite morphology. Moreover, GQDs on the composite surface and edge would ensure sufficient electrolyte penetration and mass transfer surrounding the material,47,48 while their linkage within the composite would establish a conductive network that significantly improves charge transfer.
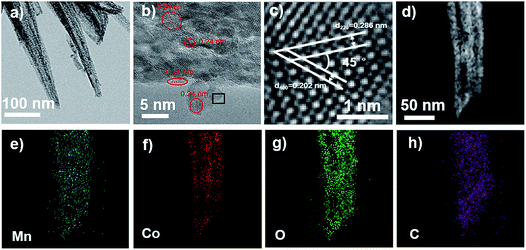 |
| Fig. 4 (a) TEM image and (b) HRTEM image of MCO-40 GQDs. (c) Inverse FFT image of a selected area found in the HRTEM image of MCO-40 GQDs. (d) High-angle annular dark-field scanning transmission electron microscopy and elemental mapping images showing the distribution of (e) Mn, (f) Co, (g) O and (h) C. | |
To examine their electrochemical performance, the composite materials were grown onto nickel foam to form free-standing electrodes for evaluation in a three electrode system with 2 M KOH electrolyte. In the cyclic voltammetry (CV) scan of the MCO electrodes, a cathodic peak and an anodic peak with clear symmetry are clearly visible, which represent the faradaic redox reaction of the Mn2+/Mn3+ and Co2+/Co3+ couples (Fig. S4a, ESI†). Their redox conversions have been previously reported and the plausible reactions MCO undergoes are described by the following equation:20
| MnCo2O4.5 + H2O + OH− MnOOH + CoOOH + e− | (1) |
| MnOOH + OH− MnO2 + H2O + e− | (2) |
CV scan comparisons between the different MCO-X GQD electrodes reveal the clear benefits of addition of the GQDs, as the integral area of the CV curves is substantially increased (Fig. S4b and 2f, ESI†). The performance improvement peaks when the GQD amount reaches 40 mg, and upon exceeding this optimal value, the specific capacitances of the resulting MCO-X GQDs decline and worsen when the GQD amount is further increased. A similar trend is also observed in the galvanostatic charge and discharge (GCD) test performed at 1 A g−1 (Fig. 5a and S5, ESI†). As previously predicted, the nanoneedle-shaped MCO-40 GQD electrode achieves the longest discharge time and the largest capacitance of 1625 F g−1 at 1 A g−1, which is around 4-fold increase in comparison to the pure MCO electrode (368 F g−1 at 1 A g−1). Fig. 5b shows the GCD curves of MCO-40 GQDs at currents ranging from 0.5 to 10 A g−1, and specific capacitances calculated from these curves indicate an 80% capacitance retention when the current density is increased from 0.5 to 10 A g−1, revealing low internal resistance and high rate capability of the material. When compared to that of MnCo2O4.5 and MnCo2O4 based materials in previous reports, the specific capacitance of the MCO-40 GQD composite is undoubtedly far superior (Table S1, ESI†).15,20,49–55
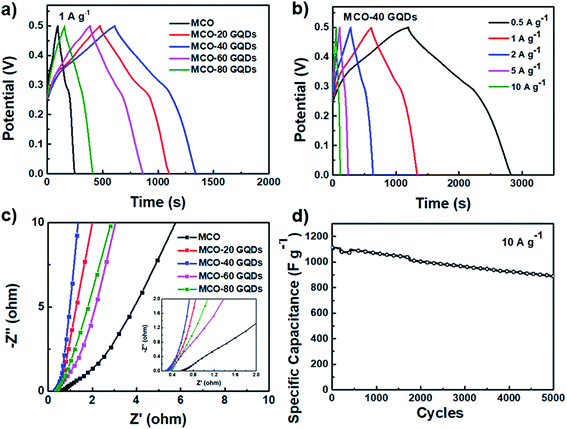 |
| Fig. 5 (a) GCD curves of the different MCO-GQD composites recorded at a current density of 1 A g−1 and (b) GCD curves of MCO-40 GQDs at different current densities. (c) Nyquist impedance plots of MCO-GQD composites in the open-circuit state. Inset shows the magnified version of the same graph. (d) Cycling stability of MCO-40 GQDs at a current density of 10 A g−1. | |
Electrode impedance spectroscopy (EIS) spectra of the various composite electrodes were also collected for comparison and the results were fitted to a Randles circuit (Fig. 5c). In the Nyquist plots, all electrodes contain a very small incomplete arc in the high frequency region which corresponds to the charge transfer process and a linear line in the mid-low frequency region which represents the capacitive and Warburg diffusion process. It can be easily identified that the series impedance of the composites is significantly reduced when GQDs are added, and this verifies the positive reinforcement GQDs have on the charge transfer of the material. Furthermore, the slope of the lines in the low frequency region rose when GQDs are present, indicating that the GQD containing composites facilitate better diffusion and exhibit stronger capacitive behaviors. The MCO-40 GQD composite also exhibits high stability as it retains 80% of its capacitance after 5000 cycles of charge and discharge at a current of 10 A g−1 (Fig. 5d). These outstanding electrochemical performances are direct results of the unique architecture induced by addition of GQDs, which provides high active site abundancy and accessibility for maximum power capability as well as an intimate contact between components for rigid structural stability and improved conductivity.
To further demonstrate the practical application of the MCO-40 GQD composite, it is assembled with a rGO electrode to construct an asymmetric MCO-40 GQDs//rGO supercapacitor. The CV curve comparison for rGO and MCO-40 GQDs is provided in Fig. S6, ESI† and the specific capacitance of the prepared rGO was measured to be 200 F g−1. Fig. 6a shows the CV curves of the asymmetric supercapacitor in a potential window of 0–1.3 V. The rectangular shape of the scans indicates good capacitive behavior of the device. The capacitance of this apparatus reaches 200 F g−1 at 0.1 A g−1 and 80 F g−1 at 5 A g−1 (Fig. 6b), corresponding to an energy density of 46 Wh kg−1 at 66 W kg−1 and 24 Wh kg−1 when the power density is drastically increased to 2800 W kg−1 (Fig. 6c). This capacitive performance is far better than that of some of the other metal oxide related devices in the literature.49,56–59 Finally, this device maintained 77% of its capacitance after 5000 cycles of operation at a current density of 1 A g−1, which is a sign of long lasting cycling stability. The robust crystal structure is further verified by post-cycling XRD analysis, which shows minimal changes in the crystal structure and morphology of MCO-40 GQDs after the cycling process (Fig. S7, ESI†).
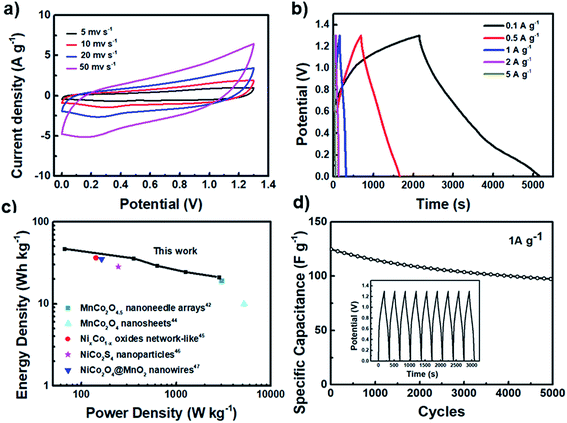 |
| Fig. 6 (a) CV graph at different scan rates, (b) GCD curves at different current densities, (c) Ragone Plot, (d) rate performances, and inside is the cycling stability test of the asymmetric MCO-40GQDs//rGO supercapacitor at 1 A g−1. | |
Conclusion
In this work, MnCo2O4.5/GQD composites with various morphologies are synthesized by a simple hydrothermal method. By tuning the GQD precursor amounts, the composites can be precisely controlled to adapt different nanostructures. Among the different products synthesized, the nanoneedle structured composite prepared using 40 mg of GQDs achieves the best electrochemical performance of 1625 F g−1 at 1 A g−1, which is about 4 times the value demonstrated by pure MnCo2O4.5 nanospheres. The GQDs are vital for achieving maximum active site abundancy and conductivity for strong energy storage capability, as well as an intimate component contact for high electrode rigidity. However, adding excessive GQDs was discovered to worsen the electrode capacitance because it begins to induce the formation of a structure that restricts ion movement. The asymmetric supercapacitor fabricated with this sample and reduced graphene oxide exhibits an energy density of 46 Wh kg−1 at a power density of 66 W kg−1, which is superior to that of other MnCo2O4.5–carbon materials in other literature. This method of utilizing GQDs for controlling the nanomaterial morphology to improve electrochemical performance can be easily adapted to other electrode materials.
Conflicts of interest
There are no conflicts to declare.
Acknowledgements
The authors acknowledge the support from the Natural Sciences and Engineering Research Council of Canada (NSERC), University of Waterloo and the Waterloo Institute of Nanotechnology.
References
- M. Salanne, B. Rotenberg, K. Naoi, K. Kaneko, P.-L. Taberna, C. P. Grey, B. Dunn and P. Simon, Efficient storage mechanisms for building better supercapacitors, Nat. Energy, 2016, 1(6), 16070 CrossRef CAS.
- W. Raza, F. Ali, N. Raza, Y. Luo, E. E. Kwon, J. Yang, S. Kumar, A. Mehmood and K.-H. Kim, Recent Advancements in Supercapacitor Technology, Nano Energy, 2018, 52, 441–473 CrossRef CAS.
- P. Simon and Y. Gogotsi, Materials for electrochemical capacitors, Nat. Mater., 2008, 7(11), 845 CrossRef CAS PubMed.
- L. Liu, Z. Niu and J. Chen, Unconventional supercapacitors from nanocarbon-based electrode materials to device configurations, Chem. Soc. Rev., 2016, 45(15), 4340–4363 RSC.
- F. Wang, X. Wu, X. Yuan, Z. Liu, Y. Zhang, L. Fu, Y. Zhu, Q. Zhou, Y. Wu and W. Huang, Latest advances in supercapacitors: from new electrode materials to novel device designs, Chem. Soc. Rev., 2017, 46(22), 6816–6854 RSC.
- Z. Yu, L. Tetard, L. Zhai and J. Thomas, Supercapacitor electrode materials: nanostructures from 0 to 3 dimensions, Energy Environ. Sci., 2015, 8(3), 702–730 RSC.
- F. Liao, X. Han, Y. Zhang, C. Xu and H. Chen, Solvothermal synthesis of porous MnCo2O4.5 spindle-like microstructures as high-performance electrode materials for supercapacitors, Ceram. Int., 2018, 44(18), 22622–22631 CrossRef CAS.
- S. G. Krishnan, M. Reddy, M. Harilal, B. Vidyadharan, I. I. Misnon, M. H. Ab Rahim, J. Ismail and R. Jose, Characterization of MgCo2O4 as an electrode for high performance supercapacitors, Electrochim. Acta, 2015, 161, 312–321 CrossRef CAS.
- K. Naoi and P. Simon, New materials and new configurations for advanced electrochemical capacitors, J. Electrochem. Soc., 2008, 17(1), 34–37 CAS.
- Y. Dong, Y. Wang, Y. Xu, C. Chen, Y. Wang, L. Jiao and H. Yuan, Facile synthesis of hierarchical nanocage MnCo2O4 for high performance supercapacitor, Electrochim. Acta, 2017, 225, 39–46 CrossRef CAS.
- L. Wu, J. Lang, P. Zhang, X. Zhang, R. Guo and X. Yan, Mesoporous Ni-doped MnCo2O4 hollow nanotubes as an anode material for sodium ion batteries with ultralong life and pseudocapacitive mechanism, J. Mater. Chem. A, 2016, 4(47), 18392–18400 RSC.
- J. Bhagwan, V. Sivasankaran, K. Yadav and Y. Sharma, Porous, one-dimensional and high aspect ratio nanofibric network of cobalt manganese oxide as a high performance material for aqueous and solid-state supercapacitor (2 V), J. Power Sources, 2016, 327, 29–37 CrossRef CAS.
- T. Pettong, P. Iamprasertkun, A. Krittayavathananon, P. Sukha, P. Sirisinudomkit, A. Seubsai, M. Chareonpanich, P. Kongkachuichay, J. Limtrakul and M. Sawangphruk, High-performance asymmetric supercapacitors of MnCo2O4 nanofibers and N-doped reduced graphene oxide aerogel, ACS Appl. Mater. Interfaces, 2016, 8(49), 34045–34053 CrossRef CAS PubMed.
- H. Che, A. Liu, J. Mu, C. Wu and X. Zhang, Template-free synthesis of novel flower-like MnCo2O4 hollow microspheres for application in supercapacitors, Ceram. Int., 2016, 42(2), 2416–2424 CrossRef CAS.
- K. N. Hui, K. San Hui, Z. Tang, V. Jadhav and Q. X. Xia, Hierarchical chestnut-like MnCo2O4 nanoneedles grown on nickel foam as binder-free electrode for high energy density asymmetric supercapacitors, J. Power Sources, 2016, 330, 195–203 CrossRef CAS.
- W. Li, K. Xu, G. Song, X. Zhou, R. Zou, J. Yang, Z. Chen and J. Hu, Facile synthesis of porous MnCo2O4.5 hierarchical architectures for high-rate supercapacitors, CrystEngComm, 2014, 16(12), 2335–2339 RSC.
- Y. Fu, H. He, X. Li, L. Wu, R. Yan, J. Zhang, X. Xu and F. Wang, Enhance supercapacitive performance of Ni foam electrode and MnCo2O4.5/Ni foam electrode, J. Mater. Sci.: Mater. Electron., 2017, 28(2), 1562–1571 CrossRef CAS.
- A. Borenstein, O. Hanna, R. Attias, S. Luski, T. Brousse and D. Aurbach, Carbon-based composite materials for supercapacitor electrodes: a review, J. Mater. Chem. A, 2017, 5(25), 12653–12672 RSC.
- J. Tian, Q. Liu, A. M. Asiri and X. Sun, Self-supported nanoporous cobalt phosphide nanowire arrays: an efficient 3D hydrogen-evolving cathode over the wide range of pH 0–14, J. Am. Chem. Soc., 2014, 136(21), 7587–7590 CrossRef CAS PubMed.
- P. Hao, Z. Zhao, L. Li, C.-C. Tuan, H. Li, Y. Sang, H. Jiang, C. Wong and H. Liu, The hybrid nanostructure of MnCo2O4.5 nanoneedle/carbon aerogel for symmetric supercapacitors with high energy density, Nanoscale, 2015, 7(34), 14401–14412 RSC.
- Y. Li, X. Peng, J. Xiang and J. Yang, Synthesis of MnCo2O4.5/Graphene Composite as Electrode Material for Supercapacitors, Int. J. Electrochem. Sci., 2017, 12(11), 10763–10772 CrossRef CAS.
- L. Ponomarenko, F. Schedin, M. Katsnelson, R. Yang, E. Hill, K. Novoselov and A. Geim, Chaotic dirac billiard in graphene quantum dots, Science, 2008, 320(5874), 356–358 CrossRef CAS PubMed.
- Z. Zhang, J. Zhang, N. Chen and L. Qu, Graphene quantum dots: an emerging material for energy-related applications and beyond, Energy Environ. Sci., 2012, 5(10), 8869–8890 RSC.
- A. B. Ganganboina, A. Dutta Chowdhury and R.-a. Doong, New avenue for appendage of graphene quantum dots on halloysite nanotubes as anode materials for high performance supercapacitors, ACS Sustainable Chem. Eng., 2017, 5(6), 4930–4940 CrossRef CAS.
- L. Chen, C. X. Guo, Q. Zhang, Y. Lei, J. Xie, S. Ee, G. Guai, Q. Song and C. M. Li, Graphene quantum-dot-doped polypyrrole counter electrode for high-performance dye-sensitized solar cells, ACS Appl. Mater. Interfaces, 2013, 5(6), 2047–2052 CrossRef CAS PubMed.
- H. Jia, Y. Cai, J. Lin, H. Liang, J. Qi, J. Cao, J. Feng and W. Fei, Heterostructural Graphene Quantum Dot/MnO2 Nanosheets toward High-Potential Window Electrodes for High-Performance Supercapacitors, Adv. Sci., 2018, 5(5), 1700887 CrossRef PubMed.
- S. Mondal, U. Rana and S. Malik, Graphene quantum dot-doped polyaniline nanofiber as high performance supercapacitor electrode materials, Chem. Commun., 2015, 51(62), 12365–12368 RSC.
- Y. Qing, Y. Jiang, H. Lin, L. Wang, A. Liu, Y. Cao, R. Sheng, Y. Guo, C. Fan and S. Zhang, Boosting the Supercapacitor Performance of Activated Carbon by Constructing Overall Conductive Networks Using Graphene Quantum Dots, J. Mater. Chem. A, 2019, 7(11), 6021–6027 RSC.
- Y. Dong, J. Shao, C. Chen, H. Li, R. Wang, Y. Chi, X. Lin and G. Chen, Blue luminescent graphene quantum dots and graphene oxide prepared by tuning the
carbonization degree of citric acid, Carbon, 2012, 50(12), 4738–4743 CrossRef CAS.
- S. K. Meher and G. R. Rao, Ultralayered Co3O4 for high-performance supercapacitor applications, J. Phys. Chem. C, 2011, 115(31), 15646–15654 CrossRef CAS.
- H.-M. Lee, V. M. G. Chandu, P. J. S. Rana, R. Vinodh, S. Kim, P. Rapur and H.-J. Kim, Hierarchical nanostructured MnCo2O4–NiCo2O4 composite as an innovative electrode for supercapacitor applications, New J. Chem., 2018, 42(21), 17190–17194 RSC.
- S. Liu, K. San Hui, K. N. Hui, J. M. Yun and K. H. Kim, Vertically stacked bilayer CuCo2O4/MnCo2O4 heterostructures on functionalized graphite paper for high-performance electrochemical capacitors, J. Mater. Chem. A, 2016, 4(21), 8061–8071 RSC.
- D. Pan, J. Zhang, Z. Li and M. Wu, Hydrothermal route for cutting graphene sheets into blue-luminescent graphene quantum dots, Adv. Mater., 2010, 22(6), 734–738 CrossRef CAS PubMed.
- L. Li, G. Wu, G. Yang, J. Peng, J. Zhao and J.-J. Zhu, Focusing on luminescent graphene quantum dots: current status and future perspectives, Nanoscale, 2013, 5(10), 4015–4039 RSC.
- J. Gu, X. Zhang, A. Pang and J. Yang, Facile synthesis and photoluminescence characteristics of blue-emitting nitrogen-doped graphene quantum dots, Nanotechnology, 2016, 27(16), 165704 CrossRef PubMed.
- L. Lin, M. Rong, F. Luo, D. Chen, Y. Wang and X. Chen, Luminescent graphene quantum dots as new fluorescent materials for environmental and biological applications, TrAC, Trends Anal. Chem., 2014, 54, 83–102 CrossRef CAS.
- S. Wang, Z.-G. Chen, I. Cole and Q. Li, Structural evolution of graphene quantum dots during thermal decomposition of citric acid and the corresponding photoluminescence, Carbon, 2015, 82, 304–313 CrossRef CAS.
- R. Ye, C. Xiang, J. Lin, Z. Peng, K. Huang, Z. Yan, N. P. Cook, E. L. Samuel, C.-C. Hwang and G. Ruan, Coal as an abundant source of graphene quantum dots, Nat. Commun., 2013, 4, 2943 CrossRef PubMed.
- P. Zheng and N. Wu, Fluorescence and sensing applications of graphene oxide and graphene quantum dots: a review, Chem.–Asian J., 2017, 12(18), 2343–2353 CrossRef CAS PubMed.
- G. M. Thorat, H. S. Jadhav and J. G. Seo, Bi-functionality of mesostructured MnCo2O4 microspheres for supercapacitor and methanol electro-oxidation, Ceram. Int., 2017, 43(2), 2670–2679 CrossRef CAS.
- G. Wei, X. Zhao, K. Du, Y. Huang, C. An, S. Qiu, M. Liu, S. Yao and Y. Wu, Flexible asymmetric supercapacitors made of 3D porous hierarchical CuCo2O4@CQDs and Fe2O3@CQDs with enhanced performance, Electrochim. Acta, 2018, 283, 248–259 CrossRef CAS.
- J. S. Wei, H. Ding, P. Zhang, Y. F. Song, J. Chen, Y. G. Wang and H. M. Xiong, Carbon dots/NiCo2O4 nanocomposites with various morphologies for high performance supercapacitors, Small, 2016, 12(43), 5927–5934 CrossRef CAS PubMed.
- Y. Li, Y. Hu, Y. Zhao, G. Shi, L. Deng, Y. Hou and L. Qu, An electrochemical avenue to green-luminescent graphene quantum dots as potential electron-acceptors for photovoltaics, Adv. Mater., 2011, 23(6), 776–780 CrossRef CAS PubMed.
- L. Ni, W. Tang, X. Liu, N. Zhang, J. Wang, S. Liang, R. Ma and G. Qiu, Hierarchical CoO/MnCo2O4.5 nanorod arrays on flexible carbon cloth as high-performance anode materials for lithium-ion batteries, Dalton
Trans., 2018, 47(11), 3775–3784 RSC.
- J. Zhu, S. Wang, J. Wang, D. Zhang and H. Li, Highly active and durable Bi2O3/TiO2 visible photocatalyst in flower-like spheres with surface-enriched Bi2O3 quantum dots, Appl. Catal., B, 2011, 102(1–2), 120–125 CrossRef CAS.
- T. Dey, S. Mukherjee, A. Ghorai, S. Das and S. K. Ray, Surface state selective tunable emission of graphene quantum dots exhibiting novel thermal quenching characteristics, Carbon, 2018, 140, 394–403 CrossRef CAS.
- Y. Zhu, X. Ji, C. Pan, Q. Sun, W. Song, L. Fang, Q. Chen and C. E. Banks, A carbon quantum dot decorated RuO2 network: outstanding supercapacitances under ultrafast charge and discharge, Energy Environ. Sci., 2013, 6(12), 3665–3675 RSC.
- Y. Zhu, Z. Wu, M. Jing, H. Hou, Y. Yang, Y. Zhang, X. Yang, W. Song, X. Jia and X. Ji, Porous NiCo2O4 spheres tuned through carbon quantum dots utilised as advanced materials for an asymmetric supercapacitor, J. Mater. Chem. A, 2015, 3(2), 866–877 RSC.
- L. Kuang, F. Ji, X. Pan, D. Wang, X. Chen, D. Jiang, Y. Zhang and B. Ding, Mesoporous MnCo2O4.5 nanoneedle arrays electrode for high-performance asymmetric supercapacitor application, Chem. Eng. J., 2017, 315, 491–499 CrossRef CAS.
- F. Li, G. Li, H. Chen, J. Q. Jia, F. Dong, Y. B. Hu, Z. G. Shang and Y. X. Zhang, Morphology and crystallinity-controlled synthesis of manganese cobalt oxide/manganese dioxides hierarchical nanostructures for high-performance supercapacitors, J. Power Sources, 2015, 296, 86–91 CrossRef CAS.
- Y. Xu, X. Wang, C. An, Y. Wang, L. Jiao and H. Yuan, Facile synthesis route of porous MnCo2O4 and CoMn2O4 nanowires and their excellent electrochemical properties in supercapacitors, J. Mater. Chem. A, 2014, 2(39), 16480–16488 RSC.
- A. K. Mondal, D. Su, S. Chen, A. Ung, H. S. Kim and G. Wang, Mesoporous MnCo2O4 with a Flake-Like Structure as Advanced Electrode Materials for Lithium-Ion Batteries and Supercapacitors, Chem.–Eur. J., 2015, 21(4), 1526–1532 CrossRef CAS PubMed.
- T. Nguyen, M. Boudard, L. Rapenne, O. Chaix-Pluchery, M. J. Carmezim and M. F. Montemor, Structural evolution, magnetic properties and electrochemical response of MnCo2O4 nanosheet films, RSC Adv., 2015, 5(35), 27844–27852 RSC.
- L.-B. Kong, C. Lu, M.-C. Liu, Y.-C. Luo, L. Kang, X. Li and F. C. Walsh, The specific capacitance of sol–gel synthesised spinel MnCo2O4 in an alkaline electrolyte, Electrochim. Acta, 2014, 115, 22–27 CrossRef CAS.
- L. Li, Y. Zhang, X. Liu, S. Shi, X. Zhao, H. Zhang, X. Ge, G. Cai, C. Gu and X. Wang, One-dimension MnCo2O4 nanowire arrays for electrochemical energy storage, Electrochim. Acta, 2014, 116, 467–474 CrossRef CAS.
- S. Sahoo, K. K. Naik and C. S. Rout, Electrodeposition of spinel MnCo2O4 nanosheets for supercapacitor applications, Nanotechnology, 2015, 26(45), 455401 CrossRef PubMed.
- Y.-M. Wang, X. Zhang, C.-Y. Guo, Y.-Q. Zhao, C.-L. Xu and H.-L. Li, Controllable synthesis of 3D NixCo1−x oxides with different morphologies for high-capacity supercapacitors, J. Mater. Chem. A, 2013, 1(42), 13290–13300 RSC.
- Y. Zhu, Z. Wu, M. Jing, X. Yang, W. Song and X. Ji, Mesoporous NiCo2S4 nanoparticles as high-performance electrode materials for supercapacitors, J. Power Sources, 2015, 273, 584–590 CrossRef CAS.
- K. Xu, W. Li, Q. Liu, B. Li, X. Liu, L. An, Z. Chen, R. Zou and J. Hu, Hierarchical mesoporous NiCo2O4@MnO2 core–shell nanowire arrays on nickel foam for aqueous asymmetric supercapacitors, J. Mater. Chem. A, 2014, 2(13), 4795–4802 RSC.
Footnote |
† Electronic supplementary information (ESI) available. See DOI: 10.1039/c9se00341j |
|
This journal is © The Royal Society of Chemistry 2019 |