DOI:
10.1039/C9SC02457C
(Edge Article)
Chem. Sci., 2019,
10, 7269-7273
Active template rotaxane synthesis through the Ni-catalyzed cross-coupling of alkylzinc reagents with redox-active esters†
Received
18th May 2019
, Accepted 15th June 2019
First published on 19th June 2019
Abstract
The synthesis of unsymmetrical axle [2]rotaxanes through a recently developed Ni-catalyzed C(sp3)–C(sp3) cross-coupling of redox-active esters (formed directly from carboxylic acids) and organozinc reagents (derived from alkyl bromides) is reported. The method also furnishes, as a minor product, the symmetrical axle [2]rotaxanes resulting from the homo-coupling of the organozinc half-thread. The rotaxanes are formed in up to 56% yield with the ratio of unsymmetrical rotaxane increasing with the cavity size of the macrocycle. In the absence of the redox-active ester neither rotaxane is formed, even though the homo-coupling rotaxane product does not incorporate the redox-active ester building block. A Ni(III) intermediate is consistent with these observations, providing support for the previously postulated mechanism of the Ni-catalyzed cross-coupling reaction.
Introduction
Active template synthesis1 exploits coupling reactions (typically metal-promoted but recently expanded to include metal-free systems2) that are accelerated through a macrocycle or loop to afford a mechanically interlocked product (rotaxane,3–5 catenane6 or knot7). The strategy has several key differences to traditional ‘passive template’ interlocked molecule synthesis,8 including avoiding the need for intercomponent recognition motifs that persist in the threaded product.9 Most active template reactions still result in new functional groups in the rotaxane axle (e.g. the CuAAC cycloaddition, the most widely employed active template reaction,3 which produces a triazole ring), but the Ni-catalyzed C(sp3)–C(sp3) homo-coupling of alkyl bromides,5 where the connection between the thread building blocks results in a C–C bond within an alkyl chain,4 does not. This type of rotaxane is sometimes referred to as an ‘impossible’10 (or ‘improbable’11) rotaxane, as the axle in the final product does not contain an obvious template nor a retrosynthetic disconnection that seemed feasible prior to the development of traceless rotaxane synthetic strategies.5,10
Aside from the conceptual interest in constructing structural- and functional-group-minimalist interlocked systems, rotaxane assembly through simple C–C bond-forming reactions allows for molecular designs free of groups that are superfluous to the function of the final structure.5,10 However, the homo-coupling of the original active template Ni-catalyzed reaction restricts its use to the synthesis of rotaxanes with symmetric axles.5 In recent years homogeneous nickel catalysis has experienced considerable progress,12 particularly with respect to alkyl–alkyl cross-coupling methods.13 In 2016, Baran and co-workers reported a Ni-catalyzed C(sp2)–C(sp3) decarboxylative cross-coupling between activated carboxylic acids, in the form of redox-active esters, and arylzinc reagents derived from aryl bromides.14a The versatility of this strategy rapidly led to the development of a range of different coupling transformations,14 including a C(sp3)–C(sp3) version using redox-active esters and alkylzinc species.14b Given its general applicability and usefulness in molecular construction, we explored the reaction's utility for active template rotaxane synthesis.
Results and discussion
Developing active template synthesis from a transition metal catalyzed reaction is far from straightforward:1 first, the metal has to stay coordinated to the macrocycle at—and between—crucial stages of the mechanism (during which the oxidation state of the ion may change several times) in order for axle formation to be directed through the cavity. Second, the macrocycle design must ensure that the metal coordinates endo-, not exo-, to the cavity and that the axle building blocks bind through opposite faces of the ring in order that their coupling leads to rotaxane formation. Finally, the conformation of the macrocycle needs to be such that the coordinated coupling reaction is favoured through the cavity rather than the generally less sterically demanding route to the side.
The Ni-catalyzed C(sp3)–C(sp3) decarboxylative cross-coupling is reported to work well with either 2,2′-bipyiridine or 4,4′-di-tert-butyl-2,2′-bipyridine ligands,14b but it was unclear whether a 6,6′-substitution pattern, which could direct a coordinated Ni-center towards the cavity of macrocycle 1a, would be tolerated by the reaction. We were pleased to find that cross-coupling of redox-active ester 2 and organozinc compound 3 (obtained from the corresponding alkyl bromide via the Grignard reagent, see ESI†) using macrocycle 1a as a ligand afforded unsymmetrical axle rotaxane 4a in an initial 16% yield (Scheme 1 and Table 1, entry 1). In addition to 4a, the symmetric [2]rotaxane, 5a, which must arise through homo-coupling of the alkylzinc reagent 3, was formed in 9% yield alongside non-interlocked threads 6 and 7. When the reaction was carried out using a macrocycle with a slightly larger cavity, 1b, the same level of conversion to rotaxane species was obtained but with an increase in the amount of cross-coupling rotaxane 4b (20%) relative to the homo-coupling product 5b (5%, Table 1, entry 2). Upon increasing the ratio of the axle building blocks to macrocycle, the amount of interlocked products increased (39%, Table 1, entry 3). While the small cavity macrocycle 1a gave the rotaxanes in a close-to-1
:
1 ratio (4a 19% and 5a 20%, Table 1, entry 3), the use of the larger macrocycle (1b) resulted in a 56% conversion with roughly 2
:
1 ratio in favour of the unsymmetrical rotaxane (4b 38% and 5b 18%, Table 1, entry 4). The reaction could be scaled up five-fold without affecting the level of conversion (0.25 mmol scale, 4b 33% and 5b 13%, Table 1, entry 5). Changing the amount of catalyst correlated linearly with the formation of the free axle, 6. However there was no significant impact on rotaxane formation; doubling the loading of NiCl2·glyme to 100 mol% resulted in 46% conversion (4b 35% and 5b 11%, Table 1, entry 6), while reducing it to 25 mol% led to 39% conversion (4b 21% and 5b 18%, Table 1, entry 7).
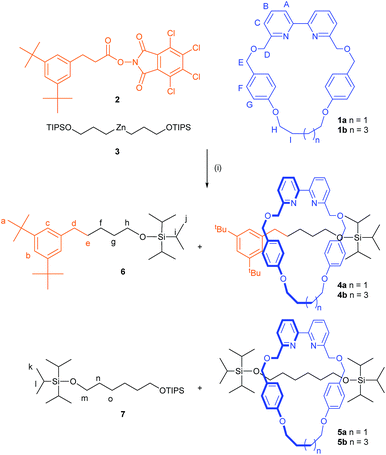 |
| Scheme 1 Synthesis of [2]rotaxanes by active template Ni-promoted coupling of a redox-active ester (2) and organozinc reagent (3). Reagents and conditions: (i) 2 (1.0 or 5.0 equiv.), 3 (2.0 or 10.0 equiv.; obtained from the corresponding alkyl bromide), 1a or 1b (1.0 equiv.), NiCl2·glyme (50 mol%), THF/DMF, r.t., 18 h. TIPS = triisopropylsilyl. | |
Table 1 Active template rotaxane formation under different reaction conditionsa
Entry |
Macrocycle |
Equiv. 2 |
Equiv. 3 |
Rotaxane yield [%] (4a : 5a)b |
Rotaxane yield [%] (4b : 5b)b |
Unless otherwise stated, 0.05 mmol (1.0 equiv.) of 1a or 1b and 0.025 mmol NiCl2·glyme (50 mol%) were used.
Ratios based on the integration of rotaxane and macrocycle 1H NMR signals.
Reaction performed at a 0.25 mmol scale with respect to 1b (1.0 equiv.).
Isolated yield and ratios.
100 mol% of NiCl2·glyme.
25 mol% of NiCl2·glyme.
|
1 |
1a
|
1.0 |
2.0 |
25 (16 : 9) |
— |
2 |
1b
|
1.0 |
2.0 |
— |
25 (4 : 1) |
3 |
1a
|
5.0 |
10.0 |
39 (19 : 20) |
— |
4 |
1b
|
5.0 |
10.0 |
— |
56 (19 : 9) |
5c |
1b
|
5.0 |
10.0 |
— |
46 (33 : 13)d |
6e |
1b
|
5.0 |
10.0 |
— |
46 (35 : 11) |
7f |
1b
|
5.0 |
10.0 |
— |
39 (7 : 6) |
8 |
1a
|
0.0 |
10.0 |
0 |
— |
9 |
1b
|
0.0 |
10.0 |
— |
0 |
We repeated similar reactions in the absence of redox-active ester 2 with the intention of obtaining exclusively the symmetric rotaxanes 5a/b, however, only the homo-coupling free thread 7 was formed under such conditions, with no interlocked species observed (Table 1, entries 8 and 9). To our initial surprise, the presence of the redox-active ester 2 appears to be essential not only for the formation of the cross-coupling rotaxanes 4a/b, but also for the homo-coupling of half-thread 3 to take place through the cavity of the macrocycle.
The interlocked structure of rotaxanes 4a and 5a was confirmed by mass spectrometry and comparison of the 1H NMR spectra with those of the non-interlocked macrocycle 1a and threads 6 and 7 (Fig. 1). In the rotaxanes the signals from the aliphatic chain in the axle are shifted upfield due to the shielding effect of the aromatic rings of the macrocycle (Fig. 1b and d). The effect is less pronounced for resonances closer to the bulky stopper groups as the macrocycle's access to this region is sterically restricted. The two faces of the macrocycle experience different chemical environments in rotaxane 4a because of the unsymmetrical axle, resulting in HE, HH and HI appearing as diastereotopic signals (Fig. 1b). In contrast, the faces of the macrocycle in symmetrical rotaxane 5a are equivalent (Fig. 1d).
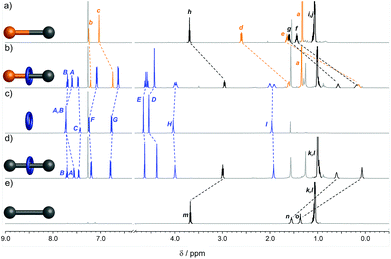 |
| Fig. 1
1H NMR spectra (600 MHz, CDCl3, 298 K) of (a) unsymmetrical thread 6, (b) rotaxane 4a, (c) macrocycle 1a, (d) rotaxane 5a, and (e) symmetrical thread 7. Assignments correspond to the labelling shown in Scheme 1. | |
The catalytic cycle likely commences14b by transmetallation of the alkylzinc unit 3 with a slowly formed Ni(I) complex A to form intermediate B (Scheme 2). Single electron transfer (SET) from the Ni centre to the phthalimide group of the redox-active ester followed by decarboxylation forms a primary alkyl radical and cationic intermediate C that after radical recombination generates Ni(III) intermediate D. This species can undergo reductive elimination15 to afford the non-interlocked thread 6, in turn regenerating the Ni(I) species A. If the reductive elimination happens through the cavity of the macrocycle, the intermediate D instead leads to the formation of the cross-coupling rotaxane 4a/b (path a).
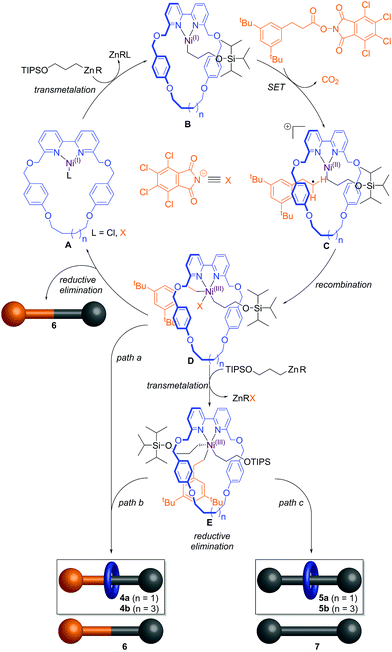 |
| Scheme 2 Proposed mechanistic cycle for the active template synthesis of rotaxanes through the Ni-promoted coupling of redox-active ester (2) and organozinc reagent (3). The key intermediate Ni(III) D leads to non-interlocked thread 6 if the reductive elimination occurs outside the macrocycle cavity or to rotaxane 4a/b if it takes place through the cavity. Alternatively, intermediate D could undergo another transmetalation event to form complex E from which either rotaxane 4a/b or 5a/b and non-interlocked thread 6 or 7 is obtained. | |
From entries 8 and 9 in Table 1, the redox-active ester is required for the homo-coupling event to take place through the cavity of the macrocycle. This can be rationalized by a second transmetalation event of Ni(III) intermediate D with organozinc unit 3,16 which would lead to intermediate E. The arrangement of the ligands around the Ni centre in E will determine the outcome of the product after the reductive elimination, forming either the cross-coupling rotaxane 4a/b and free thread 6 (path b) or the homo-coupling rotaxane 5a/b and the corresponding non-interlocked thread 7 (path c). Thus the experimental observations are consistent with, and supportive of, the original Ni(III)-intermediate mechanism postulated14b by Baran and co-workers.
Conclusions
6,6′-Substituted bipyridine macrocycles enable the active template synthesis of unsymmetrical alkyl chain axle rotaxanes through the Ni-catalyzed decarboxylative C(sp3)–C(sp3) cross-coupling of redox-active ester and organozinc building blocks derived from carboxylic acids and alkyl bromides. Rotaxane formation, including that of a minor homo-coupling product, is consistent with—and supportive of—the Ni(III) intermediate mechanism originally suggested for the coupling reaction. The combination of coordination assembly and catalysis inherent to active metal template synthesis is not only a useful tool for making functional-group-minimalist molecular structures, but can also provide experimental evidence3b,5 with regards to reaction mechanism.
Conflicts of interest
There are no conflicts to declare.
Acknowledgements
We thank the Engineering and Physical Sciences Research Council (EPSRC; EP/P027067/1) and the EU (European Research Council (ERC) Advanced Grant no. 786630; H2020 project Euro-Sequences, H2020-MSCA-ITN-2014, Grant no. 642083) for funding, the University of Manchester Mass Spectrometry Service Centre for high-resolution mass spectrometry, and Dr Brady Worrell for useful discussions. D. A. L. is a Royal Society Research Professor.
Notes and references
-
(a) J. D. Crowley, S. M. Goldup, A.-L. Lee, D. A. Leigh and R. T. McBurney, Chem. Soc. Rev., 2009, 38, 1530–1541 RSC;
(b) M. Denis and S. M. Goldup, Nat. Rev. Chem., 2017, 1, 1–17 CrossRef.
-
(a) G. De Bo, G. Dolphijn, C. T. McTernan and D. A. Leigh, J. Am. Chem. Soc., 2017, 139, 8455–8457 CrossRef CAS PubMed;
(b) S. D. P. Fielden, D. A. Leigh, C. T. McTernan, B. Pérez-Saavedra and I. J. Vitorica-Yrezabal, J. Am. Chem. Soc., 2018, 140, 6049–6052 CrossRef CAS PubMed.
- For examples of CuAAC active metal template synthesis, see:
(a) V. Aucagne, K. D. Hänni, D. A. Leigh, P. J. Lusby and D. B. Walker, J. Am. Chem. Soc., 2006, 128, 2186–2187 CrossRef CAS PubMed;
(b) V. Aucagne, J. Berna, J. D. Crowley, S. M. Goldup, K. D. Hänni, D. A. Leigh, P. J. Lusby, V. E. Ronaldson, A. M. Z. Slawin, A. Viterisi and D. B. Walker, J. Am. Chem. Soc., 2007, 129, 11950–11963 CrossRef CAS PubMed;
(c) H. Lahlali, K. Jobe, M. Watkinson and S. M. Goldup, Angew. Chem., Int. Ed., 2011, 50, 4151–4155 CrossRef CAS PubMed;
(d) B. Lewandowski, G. De Bo, J. W. Ward, M. Papmeyer, S. Kuschel, M. J. Aldegunde, P. M. E. Gramlich, D. Heckmann, S. M. Goldup, D. M. D'Souza, A. E. Fernandes and D. A. Leigh, Science, 2013, 339, 189–193 CrossRef CAS PubMed;
(e) M. J. Langton, Y. Xiong and P. D. Beer, Chem.–Eur. J., 2015, 21, 18910–18914 CrossRef CAS PubMed;
(f) A. Noor, W. K. C. Lo, S. C. Moratti and J. D. Crowley, Chem. Commun., 2014, 50, 7044–7047 RSC;
(g) J. E. M. Lewis, J. Winn, L. Cera and S. M. Goldup, J. Am. Chem. Soc., 2016, 138, 16329–16336 CrossRef CAS PubMed;
(h) A. Brown, T. Lang, K. M. Mullen and P. D. Beer, Org. Biomol. Chem., 2017, 15, 4587–4594 RSC;
(i) G. De Bo, M. A. Y. Gall, M. O. Kitching, S. Kuschel, D. A. Leigh, D. J. Tetlow and J. W. Ward, J. Am. Chem. Soc., 2017, 139, 10875–10879 CrossRef CAS PubMed;
(j) G. De Bo, M. A. Y. Gall, S. Kuschel, J. De Winter, P. Gerbaux and D. A. Leigh, Nat. Nanotechnol., 2018, 13, 381–385 CrossRef CAS PubMed;
(k) M. A. Jinks, A. de Juan, M. Denis, C. J. Fletcher, M. Galli, E. M. G. Jamieson, F. Modicom, Z. Zhang and S. M. Goldup, Angew. Chem., Int. Ed., 2018, 57, 14806–14810 CrossRef CAS PubMed;
(l) F. Modicom, E. M. G. Jamieson, E. Rochette and S. M. Goldup, Angew. Chem., Int. Ed., 2019, 58, 3875–3879 CrossRef CAS PubMed.
- For examples of active template synthesis involving C–C bond formation, see:
(a) J. Berna, J. D. Crowley, S. M. Goldup, K. D. Hänni, A.-L. Lee and D. A. Leigh, Angew. Chem., Int. Ed., 2007, 46, 5709–5713 CrossRef CAS PubMed;
(b) J. D. Crowley, K. D. Hänni, A.-L. Lee and D. A. Leigh, J. Am. Chem. Soc., 2007, 129, 12092–12093 CrossRef CAS PubMed;
(c) J. Berna, S. M. Goldup, A.-L. Lee, D. A. Leigh, M. D. Symes, G. Teobaldi and F. Zerbetto, Angew. Chem., Int. Ed., 2008, 47, 4392–4396 CrossRef CAS PubMed;
(d) S. M. Goldup, D. A. Leigh, P. J. Lusby, R. T. McBurney and A. M. Z. Slawin, Angew. Chem., Int. Ed., 2008, 47, 3381–3384 CrossRef CAS PubMed;
(e) J. D. Crowley, S. M. Goldup, N. D. Gowans, D. A. Leigh, V. E. Ronaldson and A. M. Z. Slawin, J. Am. Chem. Soc., 2010, 132, 6243–6248 CrossRef CAS PubMed;
(f) S. Hoekman, M. O. Kitching, D. A. Leigh, M. Papmeyer and D. Roke, J. Am. Chem. Soc., 2015, 137, 7656–7659 CrossRef CAS PubMed;
(g) L. D. Movsisyan, M. Franz, F. Hampel, A. L. Thompson, R. R. Tykwinski and H. L. Anderson, J. Am. Chem. Soc., 2016, 138, 1366–1376 CrossRef CAS PubMed;
(h) C. M. Storey, M. R. Gyton, R. E. Andrew and A. B. Chaplin, Angew. Chem., Int. Ed., 2018, 57, 12003–12006 CrossRef CAS PubMed;
(i) M. Franz, J. A. Januszewski, F. Hampel and R. R. Tykwinski, Eur. J. Org. Chem., 2019, 3503–3512 CrossRef CAS.
-
(a) S. M. Goldup, D. A. Leigh, R. T. McBurney, P. R. McGonigal and A. Plant, Chem. Sci., 2010, 1, 383–386 RSC;
(b) H. M. Cheng, D. A. Leigh, F. Maffei, P. R. McGonigal, A. M. Z. Slawin and J. Wu, J. Am. Chem. Soc., 2011, 133, 12298–12303 CrossRef CAS PubMed;
(c) J. J. Danon, D. A. Leigh, P. R. McGonigal, J. W. Ward and J. Wu, J. Am. Chem. Soc., 2016, 138, 12643–12674 CrossRef CAS PubMed.
-
(a) S. M. Goldup, D. A. Leigh, T. Long, P. R. McGonigal, M. D. Symes and J. Wu, J. Am. Chem. Soc., 2009, 131, 15924–15929 CrossRef CAS PubMed;
(b) J. E. M. Lewis, F. Modicom and S. M. Goldup, J. Am. Chem. Soc., 2018, 140, 4787–4791 CrossRef CAS PubMed;
(c) M. Denis, J. E. M. Lewis, F. Modicom and S. M. Goldup, Chem, 2019, 5, 1512–1520 CrossRef CAS.
-
(a) P. E. Barran, H. L. Cole, S. M. Goldup, D. A. Leigh, P. R. McGonigal, M. D. Symes, J. Wu and M. Zengerle, Angew. Chem., Int. Ed., 2011, 50, 12280–12284 CrossRef CAS PubMed;
(b) C. Romuald and F. Coutrot, Angew. Chem., Int. Ed., 2012, 51, 2544–2545 CrossRef CAS PubMed.
-
(a)
Molecular Catenanes, Rotaxanes and Knots, ed. J.-P. Sauvage and C. O. Dietrich-Buchecker, Wiley-VCH, Weinheim, 1999 Search PubMed;
(b) J. E. Beves, B. A. Blight, C. J. Campbell, D. A. Leigh and R. T. McBurney, Angew. Chem., Int. Ed., 2011, 50, 9260–9327 CrossRef CAS PubMed;
(c) J. E. M. Lewis, P. D. Beer, S. J. Loeb and S. M. Goldup, Chem. Soc. Rev., 2017, 46, 2577–2591 RSC.
-
C. J. Bruns and J. F. Stoddart, The Nature of the Mechanical Bond, John Wiley & Sons, Inc., Hoboken, NJ, USA, 2016 Search PubMed.
-
(a) J. S. Hannam, S. M. Lacy, D. A. Leigh, C. G. Saiz, A. M. Z. Slawin and S. G. Stitchell, Angew. Chem., Int. Ed., 2004, 43, 3260–3264 CrossRef CAS PubMed;
(b) S. Chao, C. Romuald, K. Fournel-Marotte, C. Clavel and F. Coutrot, Angew. Chem., Int. Ed., 2014, 53, 6914–6919 CrossRef CAS PubMed;
(c) E. A. Neal and S. M. Goldup, Chem. Commun., 2014, 50, 5128–5142 RSC;
(d) P. Waels, C. Clavel, K. Fournel-Marotte and F. Coutrot, Chem. Sci., 2015, 6, 4828–4836 RSC.
- B. Riss-Yaw, C. Clavel, P. Laurent, P. Waels and F. Coutrot, Chem.–Eur. J., 2018, 24, 13659–13666 CrossRef CAS PubMed.
- S. Z. Tasker, E. A. Standley and T. F. Jamison, Nature, 2014, 509, 299–309 CrossRef CAS PubMed.
- J. Choi and G. C. Fu, Science, 2017, 356, eaaf7230 CrossRef PubMed.
-
(a) J. Cornella, J. T. Edwards, T. Qin, S. Kawamura, J. Wang, C.-M. J. Pan, R. Gianatassio, M. Schmidt, M. D. Eastgate and P. S. Baran, J. Am. Chem. Soc., 2016, 138, 2174–2177 CrossRef CAS PubMed;
(b) T. Qin, J. Cornella, C. Li, L. R. Malins, J. T. Edwards, S. Kawamura, B. D. Maxwell, M. D. Eastgate and P. S. Baran, Science, 2016, 352, 801–805 CrossRef CAS PubMed;
(c) J. Wang, T. Qin, T.-G. Chen, L. Wimmer, J. T. Edwards, J. Cornella, B. Vokits, S. A. Shaw and P. S. Baran, Angew. Chem., Int. Ed., 2016, 55, 9676–9679 CrossRef CAS PubMed;
(d) F. Toriyama, J. Cornella, L. Wimmer, T.-G. Chen, D. D. Dixon, G. Creech and P. S. Baran, J. Am. Chem. Soc., 2016, 138, 11132–11135 CrossRef CAS PubMed;
(e) T. Qin, L. R. Malins, J. T. Edwards, R. R. Merchant, A. J. E. Movak, J. Z. Zhong, R. B. Mills, M. Yan, C. Yuan, M. D. Eastgate and P. S. Baran, Angew. Chem., Int. Ed., 2017, 129, 260–265 CrossRef PubMed;
(f) C. Li, J. Wang, L. M. Barton, S. Yu, M. Tian, D. S. Peters, M. Kumar, A. W. Yu, K. A. Johnson, A. K. Chatterjee, M. Yan and P. S. Baran, Science, 2017, 356, eaam7355 CrossRef PubMed;
(g) J. T. Edwards, R. R. Merchant, K. S. McClymont, K. W. Knouse, T. Qin, L. R. Malins, B. Vokits, S. A. Shaw, D.-H. Bao, F.-L. We, T. Zhou, M. D. Eastgate and P. S. Baran, Nature, 2017, 545, 213–218 CrossRef CAS PubMed;
(h) T. Qin, L. R. Malins, J. T. Edwards, R. R. Merchant, A. J. E. Novak, J. Z. Zhong, R. B. Mills, M. Yan, C. Yuan, M. D. Eastgate and P. S. Baran, Angew. Chem., Int. Ed., 2017, 56, 260–265 CrossRef CAS PubMed;
(i) J. M. Smith, T. Qin, R. R. Merchant, J. T. Edwards, L. R. Malins, Z. Liu, G. Che, Z. Shen, S. A. Shaw, M. D. Eastgate and P. S. Baran, Angew. Chem., Int. Ed., 2017, 56, 11906–11910 CrossRef CAS PubMed;
(j) A. Fawcett, J. Pradeilles, Y. Wang, T. Mutsuga, E. L. Myers and V. K. Aggarwal, Science, 2017, 357, 283–286 CrossRef CAS PubMed;
(k) L. Candish, M. Teders and F. Glorius, J. Am. Chem. Soc., 2017, 139, 7440–7443 CrossRef CAS PubMed;
(l) R. R. Merchant, K. M. Oberg, Y. Lin, A. J. E. Novak, J. Felding and P. S. Baran, J. Am. Chem. Soc., 2018, 140, 7462–7465 CrossRef CAS PubMed;
(m) T.-G. Chen, L. M. Barton, Y. Lin, J. Tsien, D. Kossler, I. Bastida, S. Asai, C. Bi, J. S. Chen, M. Shan, H. Fang, F. G. Fang, H. Choi, L. Hawkins, T. Qin and P. S. Baran, Nature, 2018, 560, 350–354 CrossRef CAS PubMed;
(n) J. Wang, M. Shang, H. Lundberg, K. S. Feu, S. J. Hecker, T. Qin, D. G. Blackmond and P. S. Baran, ACS Catal., 2018, 8, 9537–9542 CrossRef CAS PubMed;
(o) S. Ni, A. F. Garrido-Castro, R. R. Merchant, J. N. deGruyter, D. C. Schmitt, J. J. Mousseau, G. M. Gallego, S. Yang, M. R. Collins, J. X. Qiao, K.-S. Yeung, D. R. Langley, M. A. Poss, P. M. Scola, T. Qin and P. S. Baran, Angew. Chem., Int. Ed., 2018, 57, 14560–14565 CrossRef CAS PubMed;
(p) T.-G. Chen, H. Zhang, P. K. Mykhailiuk, R. R. Merchant, C. A. Smith, T. Qin and P. S. Baran, Angew. Chem., Int. Ed., 2019, 58, 1–6 CrossRef; For examples of C(sp3)–C(sp3) coupling where the carboxylic acid is not pre-activated, see:
(q) C. P. Johnston, R. T. Smith, S. Allmendinger and D. W. C. MacMillan, Nature, 2016, 536, 322–325 CrossRef CAS PubMed;
(r) X. Lu, B. Xiao, L. Liu and Y. Fu, Chem.–Eur. J., 2016, 22, 11161–11164 CrossRef CAS PubMed.
- J. R. Bour, N. M. Camasso, E. A. Meucci, J. W. Kampf, A. J. Canty and M. S. Sandford, J. Am. Chem. Soc., 2016, 138, 16105–16111 CrossRef CAS PubMed.
- B. Zheng, F. Tang, J. Luo, J. W. Schultz, N. P. Rath and L. M. Mirica, J. Am. Chem. Soc., 2014, 136, 6499–6504 CrossRef CAS PubMed.
Footnote |
† Electronic supplementary information (ESI) available. See DOI: 10.1039/c9sc02457c |
|
This journal is © The Royal Society of Chemistry 2019 |
Click here to see how this site uses Cookies. View our privacy policy here.