DOI:
10.1039/C9RA09031B
(Paper)
RSC Adv., 2019,
9, 41868-41876
Immobilized TiO2 nanoparticles on carbon nanotubes: an efficient heterogeneous catalyst for the synthesis of chromeno[b]pyridine derivatives under ultrasonic irradiation†
Received
1st November 2019
, Accepted 11th December 2019
First published on 17th December 2019
Abstract
A new protocol for the synthesis of chromeno[b]pyridine derivatives is described via a three-component reaction of 4-aminocoumarin, aromatic aldehydes and malononitrile catalyzed by TiO2 nanoparticles immobilized on carbon nanotubes (TiO2-CNTs) as an efficient heterogeneous catalyst under ultrasonic irradiation in water. The sustainable and economic benefits of the protocol are the high yields of products, short reaction time, simple work-up procedure, and use of a non-toxic and reusable catalyst.
1. Introduction
The chromene nucleus is a well-known favored structure motif, common and significant feature of a variety of natural products and medicinal agnates.1 Compounds containing the chromene motif have been successfully used as cosmetics, pigments2 and potential biodegradable agrochemicals.3 These compounds exhibit a wide range of interesting biological activities such as antimicrobial,4 antiviral,5,6 antitumoral,7 mutagenicitical,8 antiproliferative,9 sex pheromonal,10 antioxidant,11 enzyme inhibitoring,12 and central nervous system active drugs.13 Moreover, compounds incorporating chromene core structures are most prevalent among natural bio-active compounds. For example uvafzlelin, as a naturally occurring 4H-chromene isolated from the stems of Uvaria ufielii, can be regarded as antimicrobial against Gram-positive and acid-fast bacteria,14 conrauinone A extracted from the bark of the tree Millettia conraui is useful in the treatment of intestinal parasites,15 and erysenegalensein C has been isolated from the bark of Erythrina senegalensis and is recognized as a drug for the treatment of stomach pain, female infertility and gonorrhoea (Fig. 1).16
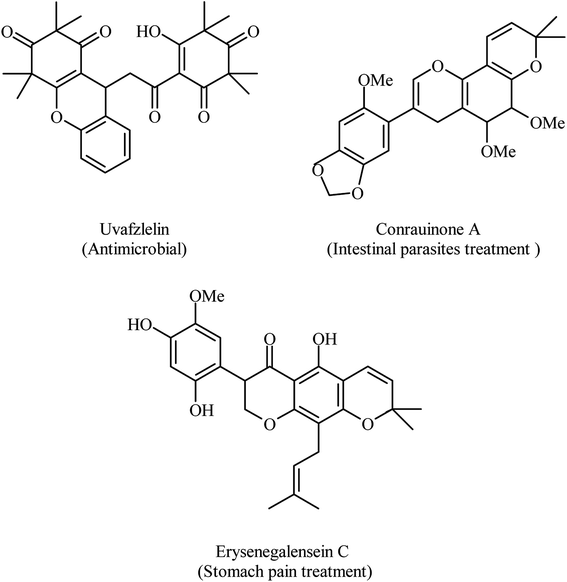 |
| Fig. 1 Natural biologically active compounds containing fused chromenes. | |
During the past decade, the utility of metal oxide nanoparticles (NPs) as efficient heterogeneous catalysts has become increasingly important aim of the organic researches in the synthesis of organic compounds. The remarkable features of these catalysts are extremely dealing with the size and shape dependent unusual physical and chemical properties.17–20 Amongst many metal oxide nanoparticles, recently titanium dioxide nanoparticles (TiO2 NPs) have been successfully employed in numerous catalytic organic and inorganic transformations due to the salient features such as high catalytic activity, non-toxicity, easy availability, moisture stability and reusability.21–28 Furthermore, carbon nanotubes (CNTs) have been emerged as promising support materials for a variety of heterogeneous nanoparticle catalysts because of their unique properties such as high quality active sites, excellent electron conductivity, chemical inertness, and retardation of electron–hole recombination.29,30 Based on, the pioneer studies of supported metal nanoparticles, the catalytic potential of TiO2 nanoparticles immobilized on carbon nanotubes (TiO2-CNTs) can be significantly improved due to their modified structural, chemical, electrical, and optical properties.31,32
Current interests in the sustainable chemistry,33 include elaborate design and development of sequences in the synthesis of alternative molecular scaffolds while combining structural diversity with eco-congeniality, are remarkable challenges for organic chemists.34 In this broad field of research, the multi-component reactions (MCRs),35 due to their ability to combine three or more reactant molecules in a single operation to construct one product containing substantial elements of all the reactants36,37 are well-established tools to achieve this near ideal goal.38–42
The use of organic solvents which is often a constitutive part of chemical or another industrial manufacturing process is mainly causing environmental pollution. According to this, attempts have been made to develop the cleaner processes using alternate energy sources and green reaction media in organic synthesis and industry. Replacing water as an ideal green medium with other popular reaction solvents, has gained increasing interest for proper practice of green chemistry because it is safe, non-toxic, unquestionably cheap and readily available.43–47 Furthermore, ultrasound can be also selected as an alternate greener approach due to its pronounced features such as improvement of reaction rates, formation of pure products in high yields and easier operation.48–50 The efficiency of sonochemistry as an innovative and powerful technique in accelerating organic transformations has been preferably expanded in several different fields of organic chemistry.51–54
We have recently developed some TiO2-CNTs catalyzed sustainable synthetic procedures leading to heterocyclic compounds.55–57 In the present work, we set out to test the efficiency of TiO2-CNTs as a reusable and neutral heterogeneous catalyst in a novel and practical approach for the preparation of 2-amino-5-oxo-4-aryl-5H-chromeno[4,3-b]pyridin-3-yl cyanides 4(a–l) via a three-component reaction of 4-aminocoumarin (1), aromatic aldehydes (2), and malononitrile (3) in aqueous media under ultrasonic irradiation (Scheme 1).
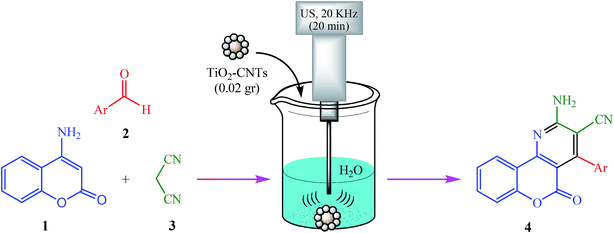 |
| Scheme 1 Ultrasound-assisted, TiO2-CNTs catalyzed preparation of 2-amino-5-oxo-4-aryl-5H-chromeno[4,3-b]pyridin-3-yl cyanides in water. | |
2. Experimental
2.1. Materials and methods
All of the chemical materials used in this work were purchased from Merck and Fluka and used without further purification. Melting points were determined on an Electrothermal 9100 apparatus. IR spectra were obtained on an ABB FT-IR (FTLA 2000) spectrometer. 1H NMR and 13C NMR spectra were recorded on a Bruker DRX-500 AVANCE at 500 and 125 MHz respectively, using TMS as internal standard and DMSO (D6) as solvent. Elemental analyses were carried out on Foss-Heraeus CHN–O-rapid analyzer instruments. The microscopic morphology of the catalyst was revealed using scanning electron microscope (SEM, Philips, XL-30) equipped with an energy dispersive X-ray detector (EDX). The transmission electron microscopy (TEM) image of the catalyst was obtained on a Philips EM208 transmission electron microscope under acceleration. Powder X-ray diffraction data were determined on a Rigaku D-max C III, X-ray Diffractometer using Cu Kα radiation (λ = 1.54 Å). Ultrasonication was performed using on a multiwave ultrasonic generator (Sonicator 3200; Bandelin, MS 73), equipped with a converter/transducer and titanium oscillator (horn), 12.5 mm in diameter, with an operation frequency of 20 KHz with a maximum power output of 200 W.
2.2. General procedure for the synthesis of TiO2-CNTs nanocomposite
The nanocomposite was synthesized by a simple sonochemical method.58 Multi walled carbon nanotubes (MWCNTs) (0.1 g) were dispersed in the mixture of solution of tetraethylorthotitanat (0.3 g) in 200 mL acetone with the aid of ultrasonication for 2 h. Further, sodium dodecyl sulfate (SDS) (0.04 g) was added as a shape controller to the above mixture. Hydrolysis was occurred rapidly when obtained mixture was poured into deionized water (200 mL). Subsequently, the produced precipitate was vacuum-filtered, washed with ethanol and dried in a vacuum oven at 77 °C for about 20 h. Finally, calcination of the resulting solid at 400 °C (heating rate: 1 °C min−1) for 30 minutes in an oven, gave the TiO2-CNTs nanocomposite powder.
2.3. General procedure for the preparation of compounds 4a–l
A mixture of 4-aminocoumarin (1, 1 mmol), aromatic aldehyde 2 (1 mmol), malononitrile (3, 1.2 mmol) and TiO2-CNTs nanocomposite (0.02 g) in H2O (3 mL) was sonicated at room temperature for 20 min in a beaker equipped with ultrasonic probe under the power of 60 W. The reaction progress was monitored by TLC using 3
:
1 ethyl acetate/n-hexane as an eluent. After completion of the reaction, the reaction was allowed to stand for 10 min at room temperature, and then the solid obtained was filtered. The residue was then dissolved in hot ethanol (3 mL) and the catalyst was removed for reusing by centrifuging, washing with ethanol, and drying in an oven. The pure product was obtained by cooling the ethanol solution to room temperature, diluted with 1 mL H2O and allowed to crystallize.
2.4. Selected spectroscopic data
2-Amino-5-oxo-4-phenyl-5H-chromeno[4,3-b]pyridin-3-yl cyanide (4a). White solid, yield: 0.295 g (94%), mp = 302–304 °C. IR (KBr): νmax 3380, 3267, 2189, 1717, 1676, 1606 cm−1. 1H NMR (500 MHz, DMSO-d6): 7.31 (2H, br d, J = 8.4 Hz, HAr), 7.36 (2H, br s, HAr), 7.38 (2H, br s, NH2), 7.45 (1H, d, J = 7.4 Hz, HAr), 7.50 (1H, t, J = 7.6 Hz, HAr), 7.71 (2H, t, J = 8.3 Hz, HAr), 7.93 (1H, d, J = 7.7 Hz, HAr) ppm. 13C NMR (125 MHz, DMSO-d6): δ 78.6, 103.4, 113.8, 117.4, 119.9, 123.4, 125.4, 129.3, 130.5, 132.0, 133.7, 141.1, 143.1, 150.2, 154.4, 162.0, 167.3 ppm. Anal. calcd for C19H11N3O2 (313.31): C, 72.84; H, 3.54; N, 13.41. Found: C, 72.94; H, 3.41; N, 13.33.
2-Amino-4-(3-chlorophenyl)-5-oxo-5H-chromeno[4,3-b]pyridin-3-yl cyanide (4b). White solid, yield: 0.323 g (93%), mp = 287–289 °C. IR (KBr): νmax 3382, 3319, 2192, 1719, 1674, 1606 cm−1. 1H NMR (500 MHz, DMSO-d6): δ 7.25 (3H, m, HAr), 7.31 (1H, d, J = 7.8 Hz, HAr), 7.34 (1H, br s, HAr), 7.43 (2H, br s, NH2), 7.45 (1H, d, J = 8.3 Hz, HAr), 7.71 (1H, t, J = 7.9 Hz, HAr), 7.91 (1H, d, J = 8.0 Hz, HAr) ppm. 13C NMR (125 MHz, DMSO-d6): δ 78.9, 104.8, 114.1, 118.4, 120.1, 123.4, 125.5, 128.5, 132.7, 133.4, 133.8, 138.2, 140.6, 141.7, 144.2, 153.0, 154.3, 163.4, 169.0 ppm. Anal. calcd for C19H10ClN3O2 (347.76): C, 65.62; H, 2.90; N, 12.08. Found: C, 65.53; H, 2.78; N, 11.91.
2-Amino-4-(2,4-dichlorophenyl)-5-oxo-5H-chromeno[4,3-b]pyridin-3-yl cyanide (4c). White solid, yield: 0.355 g (93%), mp = 296–298 °C. IR (KBr): νmax 3419, 3280, 2197, 1715, 1674, 1590 cm−1. 1H NMR (500 MHz, DMSO-d6): δ 7.37 (2H, m, HAr), 7.43 (2H, br s, NH2), 7.49 (3H, m, HAr), 7.73 (1H, t, J = 7.1 Hz, HAr), 7.95 (1H, d, J = 7.1 Hz, HAr) ppm. 13C NMR (125 MHz, DMSO-d6): δ 79.7, 102.2, 113.4, 117.5, 120.1, 123.3, 125.6, 130.0, 132.2, 134.0, 135.2, 138.0, 139.1, 140.6, 142.7, 153.4, 155.5, 162.3, 170.0 ppm. Anal. calcd for C19H9Cl2N3O2 (382.20): C, 59.71; H, 2.37; N, 11.00. Found: C, 59.87; H, 2.24; N, 11.11.
2-Amino-4-(4-cyanophenyl)-5-oxo-5H-chromeno[4,3-b]pyridin-3-yl cyanide (4d). White solid, yield: 0.321 g (95%), mp = 300–302 °C. IR (KBr): νmax 3483, 3428, 2234, 2197, 1718, 1676, 1601 cm−1. 1H NMR (500 MHz, DMSO-d6): δ 7.49 (1H, d, J = 8.2 Hz, HAr), 7.52 (1H, t, J = 7.8 Hz, HAr), 7.57 (2H, br s, NH2), 7.60 (2H, d, J = 8.2 Hz, HAr), 7.74 (1H, t, J = 7.7 Hz, HAr), 7.91 (1H, d, J = 7.8 Hz, HAr), 8.18 (2H, d, J = 8.2 Hz, HAr) ppm. 13C NMR (125 MHz, DMSO-d6): δ 78.8, 103.6, 113.8, 115.6, 117.5, 119.7, 120.5, 123.4, 125.6, 130.0, 133.4, 141.1, 143.1, 144.4, 153.1, 154.8, 162.4, 169.6 ppm. Anal. calcd for C20H10N4O2 (338.32): C, 71.00; H, 2.98; N, 16.56. Found: C, 71.14; H, 3.08; N, 16.71.
2-Amino-4-(4-fluorophenyl)-5-oxo-5H-chromeno[4,3-b]pyridin-3-yl cyanide (4e). White solid, yield: 0.318 g (96%), mp = 256–258 °C. IR (KBr): νmax 3391, 3311, 2199, 1712, 1676, 1606 cm−1. 1H NMR (500 MHz, DMSO-d6): δ 7.29 (2H, d, J = 8.0 Hz, HAr), 7.30 (1H, d, J = 7.8 Hz, HAr), 7.43 (2H, br s, NH2), 7.49 (2H, d, J = 8.0 Hz, HAr), 7.52 (1H, d, J = 7.9 Hz, HAr), 7.73 (1H, t, J = 7.8 Hz, HAr), 7.94 (1H, d, J = 7.7 Hz, HAr) ppm. 13C NMR (125 MHz, DMSO-d6): δ 78.6, 104.2, 113.8, 117.4, 119.8, 121.1, 123.4, 130.8, 132.2, 133.8, 139.0, 143.6, 150.1, 158.9, 161.8, 169.9 ppm. Anal. calcd for C19H10FN3O2 (331.30): C, 68.88; H, 3.04; N, 12.68. Found: C, 68.71; H, 2.92; N, 12.82.
2-Amino-4-(3-hydroxyphenyl)-5-oxo-5H-chromeno[4,3-b]pyridin-3-yl cyanide (4f). White solid, yield: 0.310 g (94%), mp = 292–294 °C. IR (KBr): νmax 3432, 3325, 3229, 2202, 1703, 1672, 1531, 1349 cm−1. 1H NMR (500 MHz, DMSO-d6): δ 7.47 (1H, d, J = 8.3 Hz, HAr), 7.51 (1H, t, J = 7.6 Hz, HAr), 7.56 (2H, br s, NH2), 7.64 (1H, t, J = 7.9 Hz, HAr), 7.73 (1H, t, J = 8.5 Hz, HAr), 7.82 (1H, d, J = 7.7 Hz, HAr), 7.92 (1H, d, J = 8.1 Hz, HAr), 8.13 (1H, br s, HAr), 8.15 (1H, m, HAr), 9.24 (1H, s, OH) ppm. 13C NMR (125 MHz, DMSO-d6): δ 77.8, 103.7, 113.8, 116.1, 117.4, 118.0, 119.8, 120.1, 123.3, 125.4, 134.0, 135.6, 140.6, 144.0, 149.9, 154.8, 159.0, 161.2, 170.0 ppm. Anal. calcd for C19H11N3O3 (329.31): C, 69.30; H, 3.37; N, 12.76. Found: C, 69.39; H, 3.23; N, 12.88.
2-Amino-4-(4-hydroxyphenyl)-5-oxo-5H-chromeno[4,3-b]pyridin-3-yl cyanide (4g). White solid, yield: 0.313 g (95%), mp = 285–287 °C. IR (KBr): νmax 3508, 3404, 3286, 2196, 1707, 1670, 1606 cm−1. 1H NMR (500 MHz, DMSO-d6): δ 6.71 (2H, d, J = 8.1 Hz, HAr), 7.07 (2H, d, J = 8.2 Hz, HAr), 7.37 (2H, br s, NH2), 7.46 (1H, d, J = 7.9 Hz, HAr), 7.50 (1H, t, J = 7.8 Hz, HAr), 7.71 (1H, t, J = 7.7 Hz, HAr), 7.90 (1H, d, J = 7.6 Hz, HAr), 9.27 (1H, s, OH) ppm. 13C NMR (125 MHz, DMSO-d6): δ 79.3, 105.3, 113.9, 116.1, 117.3, 120.3, 123.2, 125.4, 133.6, 134.6, 140.9, 143.8, 150.5, 155.0, 158.4, 160.4, 171.1 ppm. Anal. calcd for C19H11N3O3 (329.31): C, 69.30; H, 3.37; N, 12.76. Found: C, 69.17; H, 3.48; N, 12.84.
2-Amino-4-(4-methoxyphenyl)-5-oxo-5H-chromeno[4,3-b]pyridin-3-yl cyanide (4h). Brick-red solid, yield: 0.330 g (96%), mp = 278–280 °C. IR (KBr): νmax 3374, 3313, 2992, 2199, 1699, 1670, 1608 cm−1.1H NMR (500 MHz, DMSO-d6): δ 3.76 (3H, s, OCH3), 7.20 (2H, d, J = 8.1 Hz, HAr), 7.40 (2H, br s, NH2), 7.50 (2H, d, J = 8.1 Hz, HAr), 7.76 (1H, d, J = 7.8 Hz, HAr), 7.80 (1H, t, J = 7.8 Hz, HAr), 8.03 (1H, t, J = 7.7 Hz, HAr), 8.22 (1H, d, J = 7.7 Hz, HAr) ppm. 13C NMR (125 MHz, DMSO-d6): δ 55.7, 79.1, 103.1, 113.6, 116.7, 117.4, 120.2, 123.3, 133.7, 136.3, 138.7, 140.9, 143.9, 150.5, 153.9, 159.2, 161.9, 168.4 ppm. Anal. calcd for C20H13N3O3 (343.34): C, 70.00; H, 3.82; N, 12.24. Found: C, 70.13; H, 3.92; N, 12.13.
2-Amino-4-(4-methylphenyl)-5-oxo-5H-chromeno[4,3-b]pyridin-3-yl cyanide (4i). White solid, yield: 0.311 g (95%), mp = 291–293 °C. IR (KBr): νmax 3465, 3305, 2879, 2199, 1707, 1674, 1600 cm−1.1H NMR (500 MHz, DMSO-d6): δ 2.27 (3H, s, CH3), 7.37 (2H, m, HAr), 7.41 (2H, br s, NH2), 7.46 (1H, d, J = 8.3 Hz, HAr), 7.51 (1H, t, J = 7.6 Hz, HAr), 7.56 (2H, br s, HAr),7.73 (1H, t, J = 7.6 Hz, HAr), 7.92 (1H, d, J = 8.3 Hz, HAr) ppm. 13C NMR (125 MHz, DMSO-d6): δ 20.9, 77.1, 103.4, 113.7, 117.5, 118.4, 120.6, 123.4, 133.9, 134.2, 138.7, 140.3, 143.1, 150.7, 155.1, 159.1, 160.5, 169.8 ppm. Anal. calcd for C20H13N3O2 (327.34): C, 73.39; H, 4.00; N, 12.84. Found: C, 73.54; H, 4.20; N, 12.67.
2-Amino-4-(3-nitrophenyl)-5-oxo-5H-chromeno[4,3-b]pyridin-3-yl cyanide (4j). Yellow solid, yield: 0.344 g (96%), mp = 284–286 °C. IR (KBr): νmax 3462, 3324, 2209, 1713, 1672, 1606, 1489, 1384 cm−1. 1H NMR (500 MHz, DMSO-d6): δ 6.68 (2H, d, J = 7.7 Hz, HAr), 6.71 (1H, d, J = 7.6 Hz, HAr), 7.11 (1H, t, J = 7.7 Hz, HAr), 7.41 (2H, br s, NH2), 7.46 (1H, d, J = 8.4 Hz, HAr), 7.49 (1H, t, J = 7.6 Hz, HAr), 7.70 (1H, t, J = 8.2 Hz, HAr), 7.91 (1H, d, J = 8.2 Hz, HAr) ppm. 13C NMR (125 MHz, DMSO-d6): δ 78.9, 105.0, 113.8, 118.1, 120.1, 121.2, 123.3, 125.6, 130.4, 133.0, 133.8, 140.6, 141.3, 145.6, 147.4, 153.0, 154.1, 162.9, 170.3 ppm. Anal. calcd for C19H10N4O4 (358.31): C, 63.69; H, 2.81; N, 15.64. Found: C, 63.51; H, 2.93; N, 15.54.
2-Amino-4-(4-pyridyl)-5-oxo-5H-chromeno[4,3-b]pyridin-3-yl cyanide (4k). Yellow solid, yield: 0.302 g (96%), mp = 289–291 °C. IR (KBr): νmax 3432, 3326, 2193, 1717, 1672, 1613, 1607, 1601 cm−1. 1H NMR (500 MHz, DMSO-d6): δ 7.47 (2H, d, J = 8.1 Hz, HAr), 7.51 (2H, br s, HAr), 7.53 (2H, br s, NH2), 7.74 (1H, t, J = 7.8 Hz, HAr), 7.79 (2H, d, J = 8.1 Hz, HAr), 8.00 (1H, d, J = 7.8 Hz, HAr) ppm. 13C NMR (125 MHz, DMSO-d6): δ 77.8, 103.7, 113.8, 117.5, 119.8, 123.4, 129.8, 133.4, 140.8, 143.9, 146.2, 148.1, 149.6, 153.1, 162.4, 169.8 ppm. Anal. calcd for C18H10N4O2 (314.30): C, 68.79; H, 3.21; N, 17.83. Found: C, 68.90; H, 3.33; N, 17.93.
2-Amino-4-(2-thiophenyl)-5-oxo-5H-chromeno[4,3-b]pyridin-3-yl cyanide (4l). White solid, yield: 0.303 g (95%), mp = 280–282 °C. IR (KBr): νmax 3404, 3299, 2192, 1711, 1676, 1608, 1588, 1525 cm−1. 1H NMR (500 MHz, DMSO-d6): δ 7.23 (2H, m, HAr), 7.28 (2H, br s, HAr), 7.33 (1H, t, J = 8.3 Hz, HAr), 7.43 (2H, br s, NH2), 7.72 (1H, t, J = 8.1 Hz, HAr), 7.92 (1H, d, J = 8.0 Hz, HAr) ppm. 13C NMR (125 MHz, DMSO-d6): δ 77.2, 103.6, 113.7, 115.1, 117.4, 119.4, 123.5, 127.6, 132.8, 134.0, 140.6, 141.4, 143.9, 150.1, 153.1, 161.1, 169.3 ppm. Anal. calcd for C17H9N3O2S (319.34): C, 63.94; H, 2.84; N, 13.16. Found: C, 64.10; H, 2.92; N, 13.04.
3. Results and discussions
Firstly, TiO2-CNTs nanocomposite was prepared via a sonochemical approach based on the work of Salavati-Niasari et al.58 The XRD pattern of CNTs, TiO2 NPs, and TiO2-CNTs nanocomposite is shown in Fig. 2. All reflection peaks in Fig. 2 can be readily indexed to the anatase phase of TiO2 in the nanocomposite. Accordingly, the position of the main peaks of CNTs such as the strong diffraction peaks at 2θ = 26.0° and 43.4° are so close to those of TiO2, which could cause to overlap the CNTs peaks by TiO2, it should be noted that these peaks are not observed in the XRD patterns.59
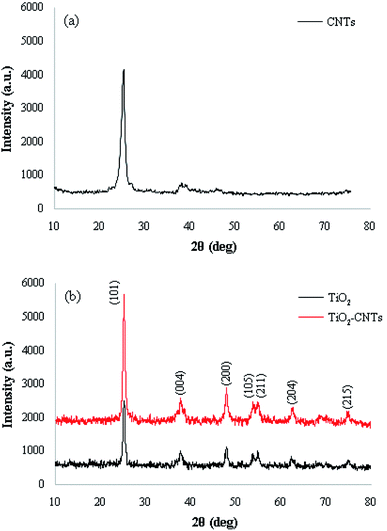 |
| Fig. 2 XRD patterns of (a) CNTs, (b) TiO2 NPs and TiO2-CNTs nanocomposite. | |
Size and morphology of the as-prepared TiO2-CNTs nanocomposite were characterized by the SEM and TEM images as depicted in Fig. 3 and 4 respectively. The SEM study reveals a continuous and mesoporous distribution of TiO2 in the form of anatase bonding to the outer surface of CNTs (Fig. 3).
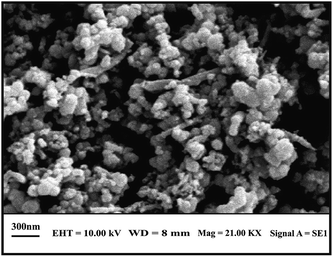 |
| Fig. 3 SEM image of TiO2-CNTs nanocomposite. | |
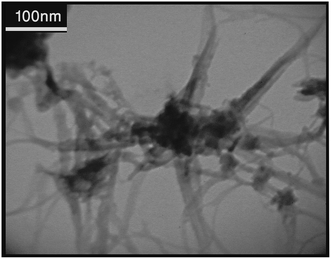 |
| Fig. 4 TEM image of TiO2-CNTs nanocomposite. | |
The average particle size of TiO2 NPs was measured by the TEM analysis to have about 16–18 nm dimensions (Fig. 4). The TEM image of CNTs also shows that CNTs are multi-walled nanotubes with the inner diameter about 12–15 nm (Fig. 5). The prepared nanocomposite was also elemental analyzed by EDX (Fig. 6a). The results show that the weight ratio is almost 50
:
50.
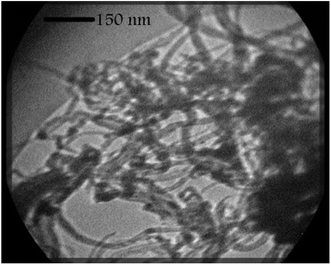 |
| Fig. 5 TEM image of CNTs nanocomposite. | |
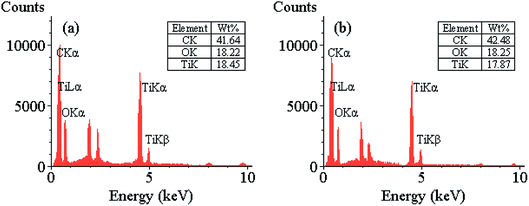 |
| Fig. 6 The results of EDX analyses of TiO2-CNTs nanocomposite (a) fresh catalyst, (b) 3rd recycle catalyst used. | |
The specific surface area and pore size distribution of CNTs and TiO2-CNTs nanocomposites were determined from the nitrogen adsorption/desorption isotherm curves (Fig. 7). The BET analyses for CNTs and TiO2-CNTs nanocomposite are also summarized in Table 1. The results indicate that the average pore diameter is reduced with the growth of CNTs over TiO2 NPs.
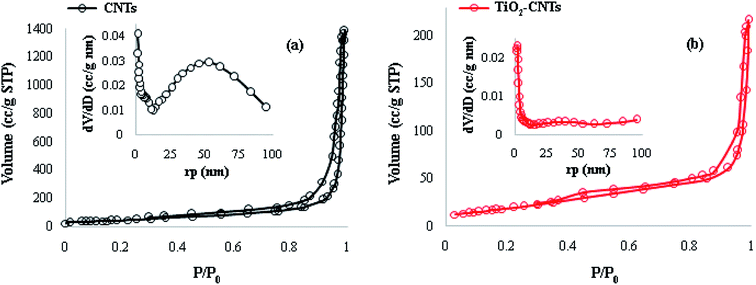 |
| Fig. 7 N2 adsorption–desorption isotherms of the (a) CNTs, (b) TiO2-CNTs. | |
Table 1 Results of BET analyses for CNTs and TiO2-CNTs
Sample |
BET surface area (m2 g−1) |
Total pore volume (cm3 g−1) |
Mean pore diameter (nm) |
CNTs |
156.32 |
2.31 |
58.43 |
TiO2-CNTs |
65.97 |
0.38 |
21.67 |
In this study, we sought to develop a simple and efficient route which allows reuse of catalyst for several times. We tried to focus on a model reaction between 4-aminocoumarin (1), benzaldehyde (2a), and malononitrile (3) for the synthesis of specific product 1a under various reaction conditions (Scheme 2 and Table 2). The preliminary experiments demonstrated that the conversion of the reactants into the desired product (4a) is mainly affected by using of TiO2-CNTs nanocomposite as catalyst. Further research on the optimal quantity of the catalyst showed that 0.03 g of catalyst, does not increase the yield of reaction (Table 2, entry 4), and this is probably because of a further amount of catalyst, can decompose the product to initial molecules. As a result, only 0.02 g of the nanocomposite was efficient to push the reaction forward in water and under ultrasonic irradiation (Table 2, entries 1–4). Whereas replacing the TiO2-CNTs nanocomposite with the bare TiO2 NPs (0.02 g), TiO2 bulk (0.02 g), and CNTs (0.02 g) under the same conditions, resulted in lower yields (Table 2, entries 3 and 5–7). In the next run of experiments, we used 0.02 g of the nanocomposite for the model reaction in water and at two different conditions involving room temperature and under reflux conditions. Interestingly, the ultrasonic irradiation allowed the catalyst to exhibit higher reactivity (Table 2, entries 3 and 8–9). In evaluating the effect of the reaction media, several solvents including, EtOH, CH2CL2, CH3CN, and DMF were tested. Results indicated that the performing reaction in water was afforded the best yield of product (94%) within 20 min. The power of ultrasonic irradiation was the last component which was explored. However, the promising results were obtained in power of 60 W (Table 2, entries 3, 14 and 15).
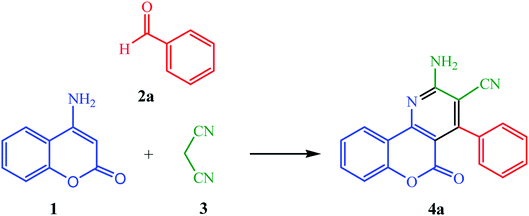 |
| Scheme 2 Synthesis of 2-amino-5-oxo-4-phenyl-5H-chromeno[4,3-b]pyridin-3-yl cyanide (4a) as model reaction for the screening of the optimized reaction conditions. | |
Table 2 Optimization of reaction conditions for the synthesis of 2-amino-5-oxo-4-phenyl-5H-chromeno[4,3-b]pyridin-3-yl cyanide (4a)
Entry |
Catalyst (g) |
Solvent (conditions) |
US powera (W) |
Time (min) |
Yieldb,c (%) |
US: ultrasonic irradiation. Isolated yield. Reaction conditions: a mixture of 4-aminocoumarin (1, 1 mmol), benzaldehyde (2a, 1 mmol), and malononitrile (3, 1.2 mmol) were kept at various reaction conditions. |
1 |
— |
H2O (US) |
60 |
40 |
56 |
2 |
TiO2-CNTs (0.01) |
H2O (US) |
60 |
30 |
82 |
3 |
TiO2-CNTs (0.02) |
H2O (US) |
60 |
20 |
94 |
4 |
TiO2-CNTs (0.03) |
H2O (US) |
60 |
20 |
90 |
5 |
TiO2 NPs (0.02) |
H2O (US) |
60 |
20 |
79 |
6 |
TiO2 bulk (0.02) |
H2O (US) |
60 |
20 |
67 |
7 |
MWCNTs (0.02) |
H2O (US) |
60 |
20 |
33 |
8 |
TiO2-CNTs (0.02) |
H2O (RT) |
— |
300 |
74 |
9 |
TiO2-CNTs (0.02) |
H2O (reflux) |
— |
120 |
89 |
10 |
TiO2-CNTs (0.02) |
EtOH (US) |
60 |
20 |
86 |
11 |
TiO2-CNTs (0.02) |
CH2CL2 (US) |
60 |
20 |
75 |
12 |
TiO2-CNTs (0.02) |
CH3CN (US) |
60 |
20 |
83 |
13 |
TiO2-CNTs (0.02) |
DMF (US) |
60 |
20 |
68 |
14 |
TiO2-CNTs (0.02) |
H2O (US) |
50 |
20 |
90 |
15 |
TiO2-CNTs (0.02) |
H2O (US) |
70 |
20 |
94 |
After completion of the model reaction, the catalyst was recovered successfully by a simple manner as described in the general procedure and subjected subsequently to at least four consecutive experiments without significant loss of activity (Scheme 3 and Table 3). From the EDX images of the fresh and third recycled catalyst reuse, the elemental structure and percentage of TiO2-CNTs nanocomposite as expected haven't changed and could be compared after and before catalysis (Fig. 6).
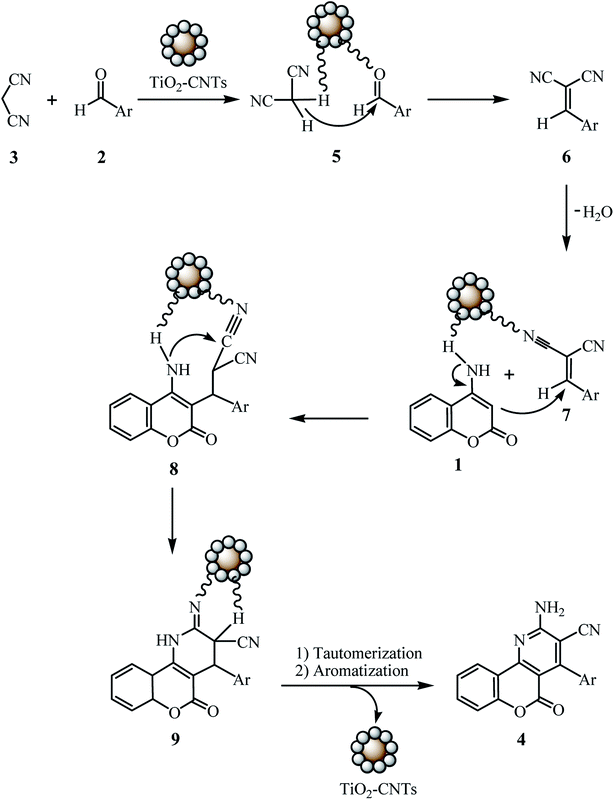 |
| Scheme 3 The proposed mechanism for the formation of 4. | |
Table 3 Recyclability of TiO2-CNTs for the synthesis of 2-amino-5-oxo-4-phenyl-5H-chromeno[4,3-b]pyridin-3-yl cyanide (4a) under optimized reaction conditions
Run |
Fresh |
1 |
2 |
3 |
4 |
Isolated yield. Reaction conditions: a mixture of 4-aminocoumarin (1, 1 mmol), benzaldehyde (2a, 1 mmol), malononitrile (3, 1.2 mmol), and in H2O (3 mL) TiO2-CNTs nanocomposite (0.02 g) in H2O (3 mL) was sonicated at room temperature for 20 min at power of 60 W. |
Isolated yield (%)a,b |
94 |
94 |
93 |
93 |
92 |
The scope and limitation of this protocol was explored by treating a range of various substituted benzaldehydes and heteroaromatic aldehydes with 4-aminocoumarin and malononitrile to form the corresponding products in high yields which were conducted under optimized reaction conditions (Table 4).
Table 4 TiO2-CNTs nanocomposite catalyzed synthesis of 2-amino-5-oxo-4-aryl-5H-chromeno[4,3-b]pyridin-3-yl cyanides 4a–l under ultrasonic irradiation in water
Products |
Ar |
Yielda,b (%) |
Mp (°C) |
Yields refer to those of pure isolated products characterized by IR, 1H NMR and 13C NMR spectral data and by elemental analyses. Reaction conditions: a mixture of 4-aminocoumarin (1, 1 mmol), aromatic aldehyde 2 (1 mmol), malononitrile (3, 1.2 mmol), and in H2O (3 mL) TiO2-CNTs nanocomposite (0.02 g) in H2O (3 mL) was sonicated at room temperature for 20 min at power of 60. |
4a |
C6H5 |
94 |
302–304 |
4b |
3-Cl–C6H4 |
93 |
287–289 |
4c |
2,4-Cl2–C6H3 |
93 |
296–298 |
4d |
4-NC–C6H4 |
95 |
300–302 |
4e |
4-F–C6H4 |
96 |
256–258 |
4f |
3-HO–C6H4 |
94 |
292–294 |
4g |
4-HO–C6H4 |
95 |
285–287 |
4h |
4-CH3O–C6H4 |
96 |
278–280 |
4i |
4-CH3–C6H4 |
95 |
291–2193 |
4j |
3-O2N–C6H4 |
96 |
284–286 |
4k |
Pyridin-4-yl |
96 |
289–291 |
4l |
Thiophen-2-yl |
95 |
280–282 |
The structures of compounds 4(a–l) were confirmed by IR, 1H NMR and 13C NMR spectroscopic data and also by elemental analyses. Spectroscopic data have been given in the Experimental Section. The synthesized catalyst was fully characterized by XRD, SEM, TEM, and EDX techniques.
Although the catalytic role of CNTs have not been proved in organic reactions, however, it is worth noting that the electron-accepting capability and conductivity of CNTs make them appropriate support to improve the conductive and dielectric properties of TiO2 NPs. In this manner, and according to the comparison as noted in entries 3, 5, and 7 in Table 2, which shows that the main catalytic role has been played by TiO2 in nanocomposite, a possible mechanism for the formation of 4 is illustrated in Scheme 2. First, TiO2 nanoparticles can act as Lewis acid–base catalyst to generate alkene 7 through the Knoevenagel condensation between aromatic aldehyde 3 and malononitrile 2. The subsequent Michael addition of 4-aminocoumarin 1 to alkene 7 is also catalyzed by TiO2 nanoparticles to form Micheal adduct 8. Further cycloaddition of amino group to the cyano moiety in intermediate 9 provides the desired product 4 after tautomerization and aromatization. In order to support experimentally the proposed mechanism, we also examined the same reaction for the synthesis 1a, in two separate steps. At first benzaldehyde (2a), and malononitrile (3) were reacted together using TiO2-CNTs (0.02 g) in the absence of 4-aminocoumarin (1). The obtained alkene 7a as solid white with mp = 87 °C (lit: 85 °C),60 was the same intermediate which was indicated on TLC in tree–component reaction. When the resulted alkene 7a reacts with 4-aminocoumarin (1) in the second step, the resulted product was exactly the same as the one obtained in one-step route.
4. Conclusion
In summary, we have reported an experimentally simple and expeditious approach to synthesize a series of chromeno[b]pyridine derivatives using 4-aminocoumarin, aromatic aldehydes, and malononitrile as substrates and TiO2-CNTs as a catalyst. This protocol offers several advantages including high yields, short reaction time, an easy work-up procedure, and catalyst reusability for several runs. It also has the ability to tolerate the use of heteroaromatic aldehydes as well as various substituted benzaldehydes.
Conflicts of interest
There are no conflicts to declare.
Acknowledgements
Shahrzad Abdolmohammadi is grateful to the Research Council of East Tehran Branch, Islamic Azad University, for financial support.
References
- R. Pratap and V. J. Ram, Chem. Rev., 2014, 114, 10476–10526 CrossRef CAS PubMed.
- G. P. Ellis, The Chemistry of Heterocyclic Compounds Chromenes, Chromanes and Chromones, ed. A.Weissberger and E. C.Taylor, John Wiley, New York, 1977, ch. 2, pp. 11–139 Search PubMed.
- E. A. A. Hafez, M. H. Elnagdi, A. G. A. Elagamey and F. M. A. A. El-Taweel, Heterocycles, 1987, 26, 903–907 CrossRef CAS.
- M. M. Khafagy, A. H. Abd el-Wahab, F. A. Eid and A. M. el-Agrody, Farmaco, 2002, 57, 715–722 CrossRef CAS PubMed.
- W. P. Smith, L. S. Sollis, D. P. Howes, C. P. Cherry, D. I. Starkey and N. K. Cobley, J. Med. Chem., 1998, 41, 787–797 CrossRef.
- A. Martinez-Grau and J. L. Marco, Bioorg. Med. Chem. Lett., 1997, 7, 3165–3170 CrossRef CAS.
- S. J. Mohr, M. A. Chirigos, F. S. Fuhrman and J. W. Pryor, Cancer Res., 1975, 35, 3750–3754 CAS.
- K. Hiramoto, A. Nasuhara, K. Michiloshi, T. Kato and K. Kikugawa, Mutat. Res., 1997, 395, 47–56 CAS.
- C. P. Dell and C. W. Smith, European patent applications EP 537949, 21 Apr 1993Chem. Abstr., 119, 1993, 139102d.
- G. Bianchi and A. Tava, Agric. Biol. Chem., 1987, 51, 2001–2002 CAS.
- K. Mukai, K. Okabe and H. Hosose, J. Org. Chem., 1989, 54, 557–560 CrossRef CAS.
- T. Ishikawa, Heterocycles, 2000, 53, 453–474 CrossRef CAS.
- F. Eiden and F. Denk, Arch. Pharm., 1991, 324, 353–354 CrossRef CAS PubMed.
- C. D. Hufford, B. O. Oguntimein, D. Van Engen, D. Muthard and J. Clardy, J. Am. Chem. Soc., 1980, 102, 7365–7367 CrossRef CAS.
- V. Fuendjiep, A. E. Nkengfack, Z. T. Fomum, B. L. Sondengam and B. Bodo, J. Nat. Prod., 1998, 61, 380–383 CrossRef CAS PubMed.
- J. Jean Wandji, Z. T. Fomum, F. Tillequin, F. Libot and M. Koch, J. Nat. Prod., 1995, 58, 105–108 CrossRef.
- C. Burda, X. B. Chen, R. Narayanan and M. A. El-Sayed, Chem. Rev., 2005, 105, 1025–1102 CrossRef CAS PubMed.
- A. Y. Kim, H. J. Lee, J. C. Park, H. Kang, H. Yang, H. Song and K. H. Park, Molecules, 2009, 14, 5169–5178 CrossRef CAS PubMed.
- K. S. Lin, C. Y. Pan, S. Chowdhury, M. T. Tu, W. T. Hong and C. T. Yeh, Molecules, 2011, 16, 348–366 CrossRef CAS PubMed.
- A. Monopoli, A. Nacci, V. Caló, F. Ciminale, P. Cotugno, A. Mangone, L. C. Giannossa, P. Azzone and N. Cioffi, Molecules, 2010, 15, 4511–4525 CrossRef CAS PubMed.
- M. L. Kantam, S. Laha, J. Yadav and B. Sreedhar, Tetrahedron Lett., 2006, 47, 6213–6216 CrossRef CAS.
- M. Hosseini-Sarvari, Acta Chim. Slov., 2007, 54, 354–359 CAS.
- J. L. Ropero-Vega, A. Aldana-Péreza, R. Gómez and M. E. Niño-Gómez, Appl. Catal., A, 2010, 379, 24–29 CrossRef CAS.
- M. Z. Kassaee, R. Mohammadi, H. Masrouri and F. Movahedi, Chin. Chem. Lett., 2011, 22, 1203–1206 CAS.
- F. Shirini, M. Alipour Khoshdel, M. Abedini and S. V. Atghia, Chin. Chem. Lett., 2011, 22, 1211–1214 CAS.
- F. Shirini, S. V. Atghia and M. Alipour Khoshdel, Iran. J. Catal., 2011, 1, 93–97 CAS.
- S. M. Sajadi, M. Naderi and S. Babadoust, J. Nat. Sci. Res., 2011, 1, 10–17 Search PubMed.
- S. Abdolmohammadi, Chin. Chem. Lett., 2012, 23, 1003–1006 CrossRef CAS.
- S. Iijima, Nature, 1991, 354, 56–58 CrossRef CAS.
- E. Auer, A. Freund, J. Pietsch and T. Tacke, Appl. Catal., A, 1998, 173, 259–271 CrossRef CAS.
- K. Woan, G. Pyrgiotakis and W. Sigmund, Adv. Mater., 2009, 21, 2233–2239 CrossRef CAS.
- J. Safari and S. Gandomi-Ravandi, J. Mol. Struct., 2014, 1065, 241–247 CrossRef.
- I. Horvath and P. Anastas, Chem. Rev., 2007, 107, 2167–2168 CrossRef CAS PubMed.
- S. L. Schreiber, Nature, 2009, 457, 153–154 CrossRef CAS PubMed.
- J. Zhu and H. Bienaymé, Multicomponent Reactions, Wiley-VCH, Weinheim, 2005 Search PubMed.
- B. M. Trost, Acc. Chem. Res., 2002, 35, 695–705 CrossRef CAS PubMed.
- P. A. Wender, V. A. Verma, T. J. Paxton and T. H. Pillow, Acc. Chem. Res., 2008, 41, 40–49 CrossRef CAS PubMed.
- S. Farshbaf, L. Sreerama, T. Khodayari and E. Vessally, Chem. Rev. Lett., 2018, 1, 56–67 Search PubMed.
- F. Behmagham, Z. Asadi and Y. J. Sadeghi, Chem. Rev. Lett., 2018, 1, 68–76 Search PubMed.
- M. Nikpassand and L. ZareFekri, Chem. Rev. Lett., 2019, 2, 7–12 Search PubMed.
- F. Valinia, N. Shojaei and P. Ojaghloo, Chem. Rev. Lett., 2019, 2, 90–97 Search PubMed.
- E. Jafari, P. Farajzadeh, N. Akbari and A. Karbakhshzadeh, Chem. Rev. Lett., 2019, 2, 123–129 Search PubMed.
- N. Parikh, D. Kumar, S. R. Roy and A. K. Chakraborti, Chem. Commun., 2011, 47, 1797–1799 RSC.
- G. Sharma, R. Kumar and A. K. Chakraborti, Tetrahedron Lett., 2008, 49, 4269–4271 CrossRef CAS.
- A. K. Chakraborti, S. Rudrawar, K. B. Jadhav, G. Kaur and S. V. Chankeshwara, Green Chem., 2007, 9, 1335–1340 RSC.
- S. V. Chankeshwara and A. K. Chakraborti, Org. Lett., 2006, 8, 3259–3262 CrossRef CAS PubMed.
- G. L. Khatik, R. Kumar and A. K. Chakraborti, Org. Lett., 2006, 8, 2433–2436 CrossRef CAS PubMed.
- B. A. Song, G. P. Zhang, S. Yang, D. Y. Hu and L. H. Jin, Ultrason. Sonochem., 2006, 13, 139–142 CrossRef CAS PubMed.
- T. J. Mason, Chem. Soc. Rev., 1997, 26, 443–451 RSC.
- R. Mawson, M. Gamage, N. S. Terefe and K. Knoerzer, Ultrasound Technol. Food Bioprocess., 2011, pp. 369–404 Search PubMed.
- Y. Q. Liu, L. H. Li, L. Yang and H. Y. Li, Chem. Pap., 2010, 64, 533–536 CAS.
- M. Meciarova, V. Polackova and S. Toma, Chem. Pap., 2002, 56, 208–213 CAS.
- M. Meciarova, S. Toma and P. Babiak, Chem. Pap., 2004, 58, 104–108 CAS.
- K. Tabatabaeian, M. Mamaghani, N. O. Mahmoodi and A. Khorshidi, Catal. Commun., 2008, 9, 416–420 CrossRef CAS.
- S. Khalilian, S. Abdolmohammadi and F. Nematolahi, Lett. Org. Chem., 2017, 14, 361–367 CrossRef CAS.
- A. Samani, S. Abdolmohammadi and A. Otaredi-Kashani, High-Throughput Screening, 2018, 21, 111–116 CrossRef CAS PubMed.
- S. Abdolmohammadi, Comb. Chem. High Throughput Screening, 2018, 21, 594–601 CrossRef CAS PubMed.
- E. Khosravifard, M. Salavati-Niasari, M. Dadkhah and Gh. Sodeifian, J. Nanostruct., 2012, 2, 191–197 Search PubMed.
- B. Gao, G. Z. Chen and G. L. Puma, Appl. Catal., B, 2009, 89, 503–509 CrossRef CAS.
- S. Balalaie and N. Nemati, Synth. Commun., 2000, 30, 869–875 CrossRef CAS.
Footnote |
† Electronic supplementary information (ESI) available. See DOI: 10.1039/c9ra09031b |
|
This journal is © The Royal Society of Chemistry 2019 |
Click here to see how this site uses Cookies. View our privacy policy here.