DOI:
10.1039/C9RA08289A
(Paper)
RSC Adv., 2019,
9, 39315-39322
Solvothermal synthesis of Mn-doped CsPbCl3 perovskite nanocrystals with tunable morphology and their size-dependent optical properties†
Received
11th October 2019
, Accepted 15th November 2019
First published on 29th November 2019
Abstract
Doping metal ions in inorganic halide perovskite (CsPbX3, X = Cl, Br, I) nanocrystals (NCs) endows the NCs with unique optical characteristics, and has thus attracted immense attention. However, controllable synthesis of high-quality doped perovskite NCs with tunable morphology still remains challenging. Here, we report a facile, effective and unified strategy for the controllable synthesis of Mn-doped CsPbCl3 quantum dots (QDs) and nanoplatelets (NPLs) via a single-step solvothermal method. The incorporation of Mn2+ into CsPbCl3 NCs introduces new broad photoluminescence (PL) emission from Mn2+ while maintaining the structure of host CsPbCl3 NCs nearly intact. The PL intensity, emission peak position and size of the NCs can be accurately adjusted by altering the experimental parameters such as Mn-to-Pb feed ratio and reaction time. Especially, by changing the amount of ligands, Mn-doped CsPbCl3 QDs, NPLs or their mixtures can be obtained. Both of the Mn-doped QDs and NPLs exhibit a size-dependent quantum confinement effect, which is confirmed by the relationship between the size of NCs and the exciton emission peaks. The solvothermal reaction condition plays an important role for the precise control of the structure, morphology and PL properties of the Mn-doped NCs. The as-prepared Mn-doped CsPbCl3 NPLs with thickness down to ∼2 nm exhibit a PL quantum yield (PLQY) of more than 22%. This work introduces a new strategy for the controllable synthesis of Mn-doped perovskite NCs, which provides ideas for the in-depth study of the dope-and-grow process and can be extended to approaches of doping other metal ions.
Introduction
Inorganic lead halide perovskite (CsPbX3, X = Cl, Br, I) nanocrystals (NCs) are novel semiconductor materials with prominent optical and electronic properties, relatively low cost and convenient liquid-phase synthesis process,1–9 thus showing promising application prospects in the fields of photodetectors,10–12 lasers,13,14 perovskite light emitting diodes (LEDs),15–20 X-ray detectors,21 bio-imaging devices,22 solar cells,9,23–25 etc. Doping provides a universal and effective way to offer the semiconductor materials with new optical, electronic and magnetic properties.26–31 For example, metal doping of II–VI semiconductor NCs (such as CdSe, ZnS) can introduce new exciton transition pathways, impart magnetism or change emission properties of the NCs.30–33 So far, the metal doping of perovskite NCs has attracted tremendous attention and in-depth research. Various kinds of metal ions, such as Bi3+, Cu2+, Ni2+, Mn2+, Cd2+, Sn2+, Rb+ and rare earth metal ions (e.g., Ce3+, Eu3+, Tb3+) have been successfully doped into perovskite NCs.34–53 These studies have proved that doping may endow perovskite NCs with enhanced photoluminescence (PL) quantum yields (QYs), new emission characteristics or improved stability, as compared to their undoped counterparts.
Among those selectable metal ions, the Mn2+ doping of CsPbX3 NCs has received great interests. Theoretically, doping of Mn2+ into CsPbX3 NCs may introduce new long-lifetime sensitized emission, where the energy transfer from the charge carriers of CsPbX3 host to the d electron of Mn2+ resulting in the d–d transition and generation of Mn2+ luminescence.42 Recently studies have shown that Mn-doped CsPbX3 (X = Cl, Br, Cl/Br) quantum dots (QDs) can be successfully synthesized via hot injection,39,40,47 anion exchange46 or anti-solvent method.44 A high Mn substitution ratio of ∼46% can be obtained for Mn doped CsPbCl3 QDs,39 while the PLQYs can be greatly enhanced due to the effective energy transfer from the CsPCl3 host to dopant Mn2+ ions.44 However, up to now the controllable synthesis of Mn-doped perovskite NCs with precisely tuned morphology and structures still remains challenging. Compared with the work of obtaining QDs, there are less studies on the preparation of Mn-doped perovskite NCs with other morphology, for example, nanoplatelets (NPLs). Controllable synthesis of Mn-doped perovskite NCs with tunable morphology is of great significant for the deeply understanding of the relationship between the microstructure and PL characteristics (i.e. emission, excitation, PLQY, lifetime, etc.) of the doped perovskite NCs. Therefore, it is required to develop a new strategy for the obtaining of Mn-doped perovskite NCs with tailored morphology and opening the way for the metal-doped perovskite NCs.
Solvothermal reaction has been demonstrated to be an effective method for the controllable synthesis of CsPbX3 NCs due to its mild crystal growth environment.54 It is believed that the solvothermal method is very suitable for the preparation of Mn-doped CsPbX3 NCs, since the process of NC nuclei growth and the Mn2+ doping would be easily controlled via the adjusting the solvothermal reaction times and/or temperatures. In this work, we developed a unified solvothermal method to prepare Mn-doped CsPbCl3 QDs and NPLs. By reacting of the Cs-oleate and Pb/MnCl2 precursors in a Teflon-lined autoclave, Mn2+ ions could be successfully doped into CsPbCl3 NCs and new Mn2+ PL emission could be introduced. Because of the sealed reaction condition, there was no need to carry out a protective gas atmosphere during experiment. Interestingly, the Mn-doped CsPbCl3 NCs show tunable morphology: Mn-doped QDs and NPLs can be well obtained via gradually changing the amount of the ligands. The relatively low solvothermal reaction temperature slows down the doping process and enables the precisely control on the emission properties, crystal structures and sizes of the Mn-doped CsPbCl3 QDs and NPLs. It is noteworthy that the as-prepared ultrathin Mn-doped CsPbCl3 NPLs have a thickness of down to ∼2 nm, which, as far as we know, has not been achieved in other works.55,56 The strategy provides a platform for further study on the mixing process of metal doping and crystal growth, which can be used as a reference for the property regulation of metal-doped perovskite NCs.
Experimental
Materials
Cesium carbonate (Cs2CO3, 99.9% metal basis) and 1-octadecene (ODE, >90%) were purchased from Macklin. Magnesium(II) chloride (MnCl2, >99% trace metal basis), lead(II) chloride (PbCl2, 99.99% metal basis), oleylamine (OLA, 70%) and oleic acid (OA, 90%) were obtained from Aladdin. Ethyl acetate (AR) and n-hexane (AR) were purchased from Beijing Chemical Reagent Ltd., China. All chemicals were employed as received with no purifications or otherwise processes.
Preparation of Cs-oleate precursor
1.995 mmol of Cs2CO3, 18 mL of ODE and 2.5 mL of OA were loaded into a 50 mL three-necked round-bottomed flask. The reaction mixture was then heated in an oil bath at 120 °C for 1 h, followed by raising the temperature to 150 °C and holding for 25 min until all Cs2CO3 reacted with OA. The Cs-oleate precursor was stored at room temperature, and a preheating process over 120 °C was necessary before use.
Preparation of Pb/MnCl2 precursor
In a typical process for Mn-doped CsPbCl3 QDs, 0.374 mmol of PbCl2, 1.87 mmol of MnCl2 and 10 mL of ODE were loaded into a 50 mL three-necked, round-bottomed flask and heated in an oil bath. When the temperature of the mixture reached 120 °C, 4 mL of OA and 4 mL of OLA were added and the temperature of the mixture was raised and kept at 130 °C until PbCl2 and MnCl2 were completely dissolved. Then the solution was naturally cooled down to the room temperature. For obtaining Mn-doped CsPbCl3 NPLs, the reaction conditions were kept as same except the amount of OA and OLA was decreased to 2–3 mL. Generally, the preheating process was not necessary for Pb/MnCl2 precursor unless precipitation occurred due to low room temperature.
Synthesis of Mn-doped CsPbCl3 NCs
17 mL of Pb/MnCl2 precursor and 0.8 mL of Cs-oleate precursor were loaded into a Teflon-lined autoclave and then maintained at 120 °C for different solvothermal reaction times. After that, the autoclave was naturally cooled down to the room temperature. All the experimental operations were operated in an open environment without protective gas atmosphere.
Isolation and purification
The Mn-doped CsPbCl3 NCs were extracted and separated from the crude solution by centrifugation at 8500 rpm for 5 min. The supernatant was then discarded and meanwhile 5 mL of n-hexene was used to redispersed the precipitates. Thereafter, 5 mL of ethyl acetate was added to induce aggregation and remove excess ligands, followed by another centrifugation at 8500 rpm for 5 min. After that, the precipitates were dispersed in 10 mL of n-hexane to form a long-term stable colloidal solution. For obtaining more uniform colloidal solution, centrifugation at 3500 rpm for 5 min is necessary to remove relatively large nanoparticles.
Characterization
The UV-visible spectrophotometry (UV-vis) measurements were collected on a Japan HITACHI U-3900H spectrometer. Transmission electron microscopy (TEM) and Energy-Dispersive X-ray (EDX) spectroscopy measurements were collected on a Philips Tecnai F20 microscope at an accelerate voltage of 200 kV. Powder X-ray diffraction patterns (XRD) were measured on a Bruker D8 Advance diffractometer using Cu Kα radiation (λ = 1.5406 Å). For the preparation of XRD samples, 1–2 mL of as-prepared colloidal solution are dripped onto a zero-diffraction silicon wafer. The PL spectra and PLQY measurements were acquired using a Fluorolog-3 Horiba Jobin-Yvon spectrofluorimeter (excited at 365 nm) equipped with an integrating sphere. PL lifetimes were measured using a time-corrected single-photon-counting (TCSPC) system. The wavelengths of the excitation sources for exciton emission peak and Mn2+ emission peak are 371 nm (by a pulsed diode light source) and 365 nm (by a Xenon lamp), respectively.
Results and discussion
In this work, the Mn-doped CsPbCl3 NCs were prepared via a facile solvothermal method in which Mn was introduced into Pb precursors by simply adding of additional MnCl2. Both of QDs and NPLs can be well prepared via changing the amount of OA and OLA in the precursor.
Synthesis and properties of Mn-doped CsPbCl3 QDs
Firstly, Mn-doped CsPbCl3 QDs with low doping concentration were prepared with a Mn-to-Pb molar feed ratio of 5
:
1 and a low solvothermal treatment temperature of 120 °C. The typical TEM image (Fig. 1a) shows that the QDs have uniform cube morphology with an average size of ∼8.2 nm. EDX analysis reveals the composition of QDs as CsPb0.94Mn0.06Cl3 (Fig. S1†). High-resolution TEM (HRTEM) image (Fig. 1b) indicates the single crystal structure and good crystallization of the QDs. The crystal lattices are observed with the interplanar distances of ∼3.98 and ∼2.86 Å, which can be assigned to the (101) and (200) planes of tetragonal CsPbCl3, respectively. XRD pattern confirms that the as-prepared QDs have crystalline structure of tetragonal CsPbCl3 (PDF card no. 18-366), which also indicates that the lightly doping of Mn2+ doesn't change the initial structure of perovskite. Moreover, as compared to pure tetragonal CsPbCl3, a slight peak shift has been observed, which can be attributed to the incorporation of Mn2+ in CsPbCl3. The Mn2+ ions (97 pm, crystal radius) are likely to occupy the position of the isovalent but larger Pb2+ ions (133 pm, crystal radius) and cause the lattice distortion.45
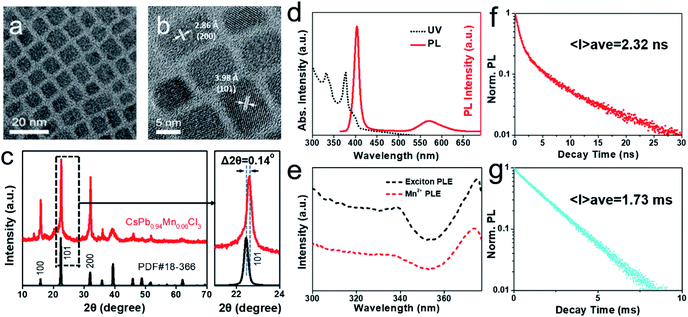 |
| Fig. 1 (a) TEM image, (b) HRTEM image, (c) XRD pattern, (d) UV-vis absorption and PL emission spectra, (e) PLE spectra, (f) exciton PL lifetime and (g) Mn2+ luminescence lifetime of a typical Mn-doped CsPbCl3 QDs (prepared using Mn-to-Pb molar feed ratio of 5 : 1 and reacting at 120 °C for 3 h). | |
Then we studied the optical properties of the as-prepared QDs. The PL excitation (PLE), UV-vis absorption and PL emission spectra are shown in Fig. 1d–e. The UV-vis spectrum (Fig. 1d) shows that the as-prepared QDs have a noticeably strong first exciton absorption peak of 377 nm, which is blue-shifted and exhibits the feature of quantum confinement effect, as compared to the bulk CsPbCl3.57 Under the excitation of UV light at 365 nm, dual emission peaks are observed in PL emission spectrum, which are located at 403 nm and 572 nm, respectively. The peak at 403 nm is attributed to the inherent exciton emission of CsPbCl3 QDs and shows a narrow FWHM (full width at half maximum) of 14 nm. The as-prepared QDs reveals a noticeable Stokes shift of 26 nm, which is larger than that obtained in previous reports.39,45 The larger Stokes shift reduces the self-absorption of QDs, which is benefit to their optical properties. Additional weak peak at 572 nm arises from the incorporation of Mn2+ and displays a relatively broad FWHM of ∼56 nm. The excitation spectra of dual peaks were also tested and shown in Fig. 1e. The excitation spectra of the exciton emission (403 nm) and Mn2+ emission (572 nm) both perform almost coincident curves with the absorption spectrum, which can be explained by the effective energy transfer from the CsPbCl3 host to dopant Mn2+ ions.44,58
To further investigate the mechanism of dual emission, the PL lifetimes of Mn-doped CsPbCl3 QDs were measured and shown in Fig. 1f and g, respectively. The dual emission peaks of the QDs have completely different PL lifetimes: the average PL lifetime of exciton emission peak (403 nm) is 2.32 ns, while the average PL lifetime of Mn2+ emission peak (572 nm) is 1.73 ms. The exciton lifetime originates from the CsPbCl3 host, which can be well fitted to two-exponential decay with a long lifetime component of 6.02 ns and a short lifetime component of 0.58 ns. As has been reported, the PL emission of CsPbCl3 derives from the radiative recombination (generated by the relaxation of excitons) and non-radiative recombination (generated by electronic traps or surface defects),14,59 corresponding to the long lifetime and short lifetime, respectively. Differently, the Mn2+ luminescence lifetime decay curve displays a noticeable single-exponential decay and an extremely long relaxation time, which is related to its unique luminescence mechanism. By combining the spectral analysis with previous report on the luminescence of Mn2+ doped semiconductors, the Mn2+ luminescence mechanism can be explained briefly as follows: the incorporation of Mn2+ provides a new energy transfer pathway from the excited CsPbCl3 host to d electrons of Mn2+, which enhances the internal d–d transition (4T1 to 6A1) of Mn2+ and generates new emission channel.42,60,61
To reveal the effect of solvothermal reaction times on the PL properties of CsPbCl3 QDs with low Mn doping ratio, we prolonged the reaction time to obtained a series of samples. As shown in Fig. S2a and Table S1,† the QDs obtained after 3, 6, 9 h display similar PL emission and their PL quantum yields (PLQYs) remains in the range of 4.8–6%. Their PL decay curves (Fig. S2b, c, Tables S1 and S2†) also display a negligible change. We believe that the earlier arrival of reaction equilibrium results in low doping ratio of Mn2+. Moreover, with the increase of reaction time, the Mn2+ doping ratio and the surface defects of the QDs did not increased notably, resulting in no significant changes in PLQYs and lifetimes. Therefore, the prolonged reaction time contributes little to increasing the doping ratio of Mn2+ into CsPbCl3 QDs.
The low Mn2+ doping ratio of the QDs suggests lower thermodynamics reactivity of Mn2+ ions as compared to Pb2+ ions during the solvothermal reaction. In order to efficiently increase the doping ratio, higher Mn-to-Pb feed ratios were employed during the preparation of Pb/MnCl2 precursor. Here, we increased the Mn-to-Pb feed ratio from 5
:
1 to 7.5
:
1 and 10
:
1 and fixed the other reaction conditions (120 °C for 3 h) to obtain Mn-doped CsPbCl3 QDs with different doping ratios. EDX measurement reveals the compositions of the QDs as CsPb0.94Mn0.06Cl3, CsPb0.85Mn0.15Cl3 and CsPb0.75Mn0.25Cl3 (Fig. S1†), respectively. XRD patterns (Fig. 2a) exhibit a continuous peak shift as the doping ratio increases. A 0.12° shift to a higher angle of (101) plane can be observed, which indicates that the increase in incorporation of Mn2+ aggravates the lattice contraction. In addition, the peaks of hexagonal CsMnCl3 (PDF card no. 25-219) and rhombohedral (i.e., trigonal) CsMnCl3 (PDF card no. 73-227) gradually appear, indicating that a relatively high doping ratio may lead Pb2+ ions sites completely replaced by Mn2+ ions in partial areas. After excess ions replacement, the crystalline structure of the CsPbCl3 host is destroyed and new phases of CsMnCl3 are formed by a spinodal decomposition process.56 The newly generated CsMnCl3 NCs exhibit hexagon morphology with relatively larger size of 16–32 nm (Fig. S3†). In fact, the sample prepared after a prolonged reaction time over 12 h exhibits the phase composition of almost h-CsMnCl3 and r-CsMnCl3 (Fig. S4†). In addition, an extra peak located at ∼25.5° is noticed in the XRD pattern (black triangle in Fig. 2a), which has also been observed in other related works.56 The existence of the extra peak may indicate that at a relatively high doping ratio, Mn2+ ions not only replace the position pf Pb2+ ions, but also have other forms of incorporation, such as occupying the space in the lattice of CsPbCl3 host.39 PL emission spectra (Fig. 2b) of the samples show that as the doping ratio increases, the intensity of the broad Mn2+ emission peak increases significantly, which is attributed to the enhancement in the energy transfer from excitons to Mn2+. The CsPb0.75Mn0.25Cl3 sample shows bright yellow-orange colored emission (Fig. S5†) with a PLQY of over 30%. As doping ratio increases, the exciton emission shows a blue shift, which is related to the size-dependent quantum confinement effect of the QDs. As is shown in the TEM images (Fig. 2c–e), the size of the QDs decreases from 8.2 nm, 6.6 nm to 5 nm.
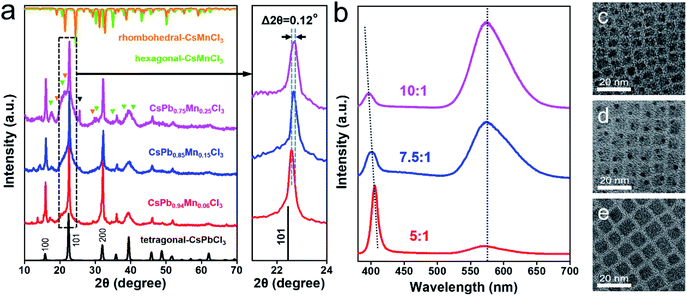 |
| Fig. 2 (a) XRD patterns of Mn-doped CsPbCl3 QDs prepared with Mn-to-Pb molar feed ratios of 10 : 1, 7.5 : 1 and 5 : 1, respectively. (Right) Magnified peak shifts of (101) plane. (b) PL emission spectra of the QDs. (c–e) TEM images of Mn-doped CsPbCl3 QDs prepared with different Mn-to-Pb molar feed ratios: 10 : 1 (c), 7.5 : 1 (d) and 5 : 1 (e). | |
Synthesis and properties of Mn-doped CsPbCl3 NPLs
The morphology of the product can be tuned by changing the amount of organic ligands (OA and OLA). Fig. 3a shows a typical TEM image of the sample obtained by using 2 mL of OA and 2 mL of OLA, while the Mn-to-Pb molar feed ratio was kept at 5
:
1. The as-prepared sample exhibits uniform platelet morphology. The observed square or thin rectangle shapes are consistent to the NPLs lying parallel or perpendicular to the carbon film, respectively. The NPLs have an average lateral length of ∼11 nm and an average thickness of ∼2.3 nm. EDX measurement exposes the NPLs with a composition of CsPb0.65Mn0.35Cl3 (Fig. S6†). As compared with the as-prepared CsPb0.94Mn0.06Cl3 QDs, the NPLs show a much higher Mn doping concentration (∼35%), suggesting that less amount of ligands contributes to the incorporation of Mn2+. High-resolution TEM (HRTEM) image (Fig. 3b) suggests the good crystallinity of NPLs with clearly lattice fringes. The distances between lattice planes of 3.95 Å and 2.78 Å correspond to the (101) and (200) planes of tetragonal CsPbCl3, respectively, which are smaller than that of Mn-doped CsPbCl3 QDs, indicating more incorporation of Mn2+. XRD result (Fig. 3c) reveals the main crystal structure of tetragonal CsPbCl3, and the appearance of CsMnCl3 phase in the product, similar as that of Mn-doped QDs.
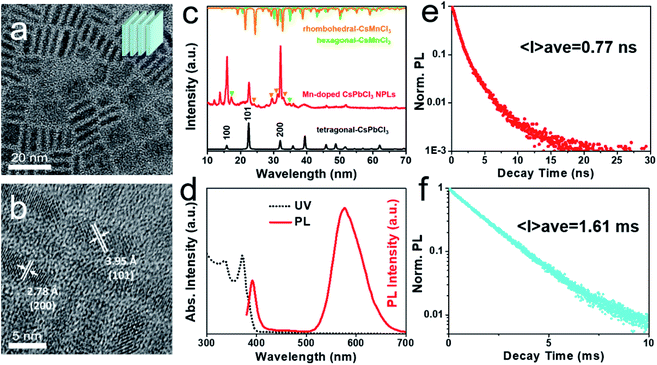 |
| Fig. 3 (a) TEM image, (b) HRTEM image, and (c) XRD pattern of typical Mn-doped CsPbCl3 NPLs (using Mn-to-Pb molar feed ratio of 5 : 1 and reacting for 4.5 h). (d) UV-vis absorption and PL emission spectra, (e) exciton PL lifetime and (f) Mn2+ luminescence lifetime spectrum of the Mn-doped CsPbCl3 NPLs. | |
The UV-vis and PL spectra of Mn-doped CsPbCl3 NPLs (Fig. 3d) display an absorption peak at 371 nm and an exciton emission peak at 392 nm, which are both blue-shifted as compared to that of Mn-doped CsPbCl3 QDs. The blue shift originates from the ultra-small thickness of NPLs, suggesting their size-dependent quantum confinement effect. The Mn2+ emission peak centered at 577 nm is red-shifted compared to that of Mn-doped CsPbCl3 QDs (572 nm), proving stronger Mn–Mn interaction and contributing an orange colored emission.57 The PL decay curves (Fig. 3e and f) of the NPLs also reveal two different lifetimes. The average PL lifetimes of exciton emission and the Mn2+ emission are 0.77 ns and 1.61 ms, respectively. As compared with Mn-doped QDs, the exciton lifetime exhibits similar two-exponential fitting curve, but the lifetime is much shorter than that of QDs (2.32 ns), which mainly related to a higher incorporation of Mn2+ and stronger energy transfer from CsPbCl3 to Mn2+. The Mn2+ luminescence lifetime curve can be better fitted to two-exponential decays than single-exponential decay, indicating that the formation of new CsMnCl3 phase causes heterogeneous environment around Mn2+ ions.56
We further studied the effects of solvothermal reaction times on the optical properties of Mn-doped CsPbCl3 NPLs. PL emission spectra (Fig. 4a) show that as the reaction time increases, the exciton emission peak shifts from 389 nm to 398 nm, which is attributed to size-dependent quantum confinement effect. Thus, we can easily and precisely control the optical properties of Mn-doped CsPbCl3 NPLs via changing the reaction times. As the reaction time increases, the surface defects decreased, resulting in stronger emission of the CsPbCl3 host and the energy transfer to Mn2+. Directly seen from the results, the prolonged reaction time yields an increase in the intensities of dual emission peaks and an increase of PLQY (Fig. 4b) from 10.4% to 22.2%. The HRTEM images of NPLs obtained for different reaction times are shown in Fig. 4c–f. The average thickness of NPLs can be accurately acquired by measuring those stacked perpendicular NPLs. With the reaction time increasing, the thickness of the NPLs changes from 2.21 ± 0.41 nm, to 2.96 ± 0.56 nm (Fig. 4h–k), exhibiting a positive correlation with the shift of exciton emission peak, as shown in Fig. 4g. The result indicates that the thickness and the emission peak position of the NPLs can be tuned precisely by the solvothermal reaction time.
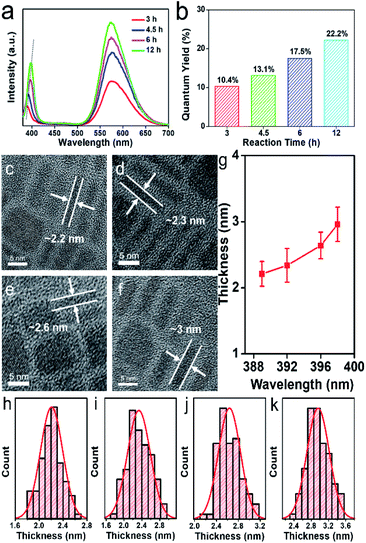 |
| Fig. 4 (a) PL emission spectra and (b) PLQYs of Mn-doped CsPbCl3 NPLs prepared under different reaction times. (c–f) HRTEM images of different NPLs prepared under 3 h (c), 4.5 h (d), 6 h (e) and 12 h (f). (g) Thickness as a function of the exciton emission peak wavelength. (h–k) The corresponding thickness histograms of Mn-doped CsPbCl3 NPLs samples. | |
Tunable morphology and optical properties of Mn-doped CsPbCl3 NCs
By carrying out synthesis processes with different amounts of organic ligands (OA and OLA), Mn-doped CsPbCl3 QDs and NPLs were successfully obtained. Then we gradually changed the amount of ligands for further investigation of ligand-dependent morphology of Mn-doped perovskite NCs. As revealed in Fig. 5a–d, the morphology changes from NPLs to QDs as the amount of ligands increases. Large amount of NPLs with a lateral size of ∼10 nm and a thickness of ∼2.2 nm (Fig. 5a, also shown in Fig. 4c) are obtained when 2 mL of OA and OLA were used. As the amount of ligand increases (3 mL of OA and OLA), ultrathin NPLs with a lateral size of ∼20 nm and a thickness of only ∼2 nm (Fig. 5b and S7†) are formed. To the best of our knowledge, the synthesis of ultrathin Mn-doped CsPbCl3 NPLs with a thickness of only ∼2 nm is challenging, which were not achieved in other works. When the amount of ligands continues to increase (3.5 mL of OA and OLA), a mixed morphology of NPLs and QDs are obtained (Fig. 5c), in which the NPLs grow larger with lateral sizes of 50–100 nm. Finally, when using 4 mL of OA and OLA, only QDs with average size of ∼8.2 nm (Fig. 5d) can be achieved. Typical PL spectra for different samples are shown in Fig. 5e. The exciton emission peaks are determined by the morphology and sizes of the NCs due to the quantum confinement effect, which is consistent with the previous discussion. The broad Mn2+ emission derives from the energy transfer from CsPbCl3 host to Mn2+. Under the same Mn-to-Pb feed ratio, Mn2+ is more likely to be doped into CsPbCl3 NPLs than that of CsPbCl3 QDs, leading to stronger intensity of Mn2+ emission peak.
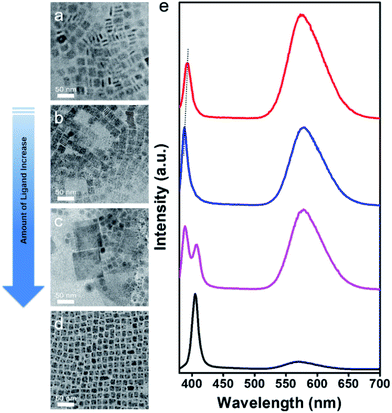 |
| Fig. 5 (a–d) Low magnification TEM images of Mn-doped CsPbCl3 NCs prepared as a function of the amount of ligand. (e) The corresponding PL emission spectra of Mn-doped CsPbCl3 NCs. | |
As we know, the organic ligands (OA and OLA) coordinate with ions and assume the function of slowing and controlling the growth of perovskite NCs.62 Due to the anisotropy of the perovskite NCs, changes in the amount of ligands give rise to different crystal growth orientation. When the amount of ligands is relatively small, two-dimensional NPLs morphology is more likely to be obtained in a relatively slow solvothermal reaction condition owing to the differential binding effect of capping ligands in different directions of the NCs. When the amount of the ligands increases, the binding effect on the NCs in different directions tends to be uniform, thereby forming morphology of QDs (nanocubes). Furthermore, because of the relatively low reactivity of Mn2+ ions, the doping process is followed by the formation of CsPbCl3 NCs. Thus, we believe that more capping ligands prevent CsPbCl3 NCs from effective incorporation of Mn2+, resulting in a lower Mn2+ concentration in CsPbCl3 QDs as compared to that of NPLs prepared under the same reaction parameters.
Conclusions
In summary, we develop a facile and effective single-step solvothermal method on controllable synthesis of Mn-doped CsPbCl3 NCs. By altering the amount of ligands, Mn-doped CsPbCl3 with morphology of QDs or NPLs can be well obtained respectively. The doping of Mn2+ introduces an energy transfer pathway from CsPbCl3 host to dopant, which leads to d–d transitions (4T1 to 6A1) of the Mn2+ and generates Mn2+ luminescence. The relatively low reactivity of Mn2+ results in the maintaining of tetragonal CsPbCl3 crystal structure. The PL intensity, emission peak position and size of the doped QDs and NPLs can be precisely controlled by varying the experimental parameters such as Mn-to-Pb feed ratio and reaction time. Both of the Mn-doped NCs exhibit strong size-dependent quantum confinement effect since the size of QDs and the thickness of NPLs are within the quantum confinement regime. The thinnest thickness of as-prepared Mn-doped CsPbCl3 NPLs reaches ∼2 nm, which is challenging in Mn-doped NPLs. The ultrathin two-dimensional structure and good crystallinity of Mn-doped CsPbCl3 NPLs suggest an excellent application adaptability. The unified solvothermal method provides not only a new approach for the controllable preparation of Mn-doped QDs and NPLs but also new ideas for the investigation of the dope-and-grow process, which can be extended to morphology-controlling engineering of perovskite with different metal dopant and halide composition.
Conflicts of interest
There are no conflicts to declare.
Acknowledgements
This work was supported by the National Natural Science Foundation of China (51972096, 51772075, 51572068, 21806029), the Natural Science Foundation of Hebei Province (E2019202086, E2019202347), and the Program for Changjiang Scholars and Innovative Research Team in University (PCSIRT: IRT17R33).
Notes and references
- L. Protesescu, S. Yakunin, M. I. Bodnarchuk, F. Krieg, R. Caputo, C. H. Hendon, R. X. Yang, A. Walsh and M. V. Kovalenko, Nano Lett., 2015, 15, 3692–3696 CrossRef CAS PubMed.
- X. J. Zhang, H. C. Wang, A. C. Tang, S. Y. Lin, H. C. Tong, C. Y. Chen, Y. C. Lee, T. L. Tsai and R. S. Liu, Chem. Mater., 2016, 28, 8493–8497 CrossRef CAS.
- J. De Roo, M. Ibanez, P. Geiregat, G. Nedelcu, W. Walravens, J. Maes, J. C. Martins, I. Van Driessche, M. V. Koyalenko and Z. Hens, ACS Nano, 2016, 10, 2071–2081 CrossRef CAS PubMed.
- Y. S. Park, S. J. Guo, N. S. Makarov and V. I. Klimov, ACS Nano, 2015, 9, 10386–10393 CrossRef CAS PubMed.
- S. Sun, D. Yuan, Y. Xu, A. Wang and Z. Deng, ACS Nano, 2016, 10, 3648–3657 CrossRef CAS PubMed.
- Z. J. Li, E. Hofman, A. H. Davis, M. M. Maye and W. Zheng, Chem. Mater., 2018, 30, 3854–3860 CrossRef CAS.
- X. Chen, L. Peng, K. Huang, Z. Shi, R. Xie and W. Yang, Nano Res., 2016, 9, 1994–2006 CrossRef CAS.
- D. Yang, Y. Zou, P. Li, Q. Liu, L. Wu, H. Hu, Y. Xu, B. Sun, Q. Zhang and S. T. Lee, Nano Energy, 2018, 47, 235–242 CrossRef CAS.
- A. Swarnkar, A. R. Marshall, E. M. Sanehira, B. D. Chernomordik, D. T. Moore, J. A. Christians, T. Chakrabarti and J. M. Luther, Science, 2016, 354, 92–95 CrossRef CAS PubMed.
- P. Ramasamy, D. H. Lim, B. Kim, S. H. Lee, M. S. Lee and J. S. Lee, Chem. Commun., 2016, 52, 2067–2070 RSC.
- A. Waleed, M. M. Tavakoli, L. L. Gu, S. Hussain, D. Q. Zhang, S. Poddar, Z. Y. Wang, R. J. Zhang and Z. Y. Fan, Nano Lett., 2017, 17, 4951–4957 CrossRef CAS PubMed.
- W. Zhai, J. Lin, C. Li, S. M. Hu, Y. Huang, C. Yu, Z. K. Wen, Z. Y. Liu, Y. Fang and C. C. Tang, Nanoscale, 2018, 10, 21451–21458 RSC.
- J. Butkus, P. Vashishtha, K. Chen, J. K. Gallaher, S. K. K. Prasad, D. Z. Metin, G. Laufersky, N. Gaston, J. E. Halpert and J. M. Hodgkiss, Chem. Mater., 2017, 29, 3644–3652 CrossRef CAS.
- F. Liu, Y. Zhang, C. Ding, S. Kobayashi, T. Izuishi, N. Nakazawa, T. Toyoda, T. Ohta, S. Hayase, T. Minemoto, K. Yoshino, S. Dai and Q. Shen, ACS Nano, 2017, 11, 10373–10383 CrossRef CAS PubMed.
- J. Pan, L. N. Quan, Y. Zhao, W. Peng, B. Murali, S. P. Sarmah, M. Yuan, L. Sinatra, N. M. Alyami and J. J. A. M. Liu, Adv. Mater., 2016, 28, 8718–8725 CrossRef CAS PubMed.
- J. Song, J. Li, X. Li, L. Xu, Y. Dong and H. Zeng, Adv. Mater., 2015, 27, 7162–7167 CrossRef CAS PubMed.
- X. Li, Y. Wu, S. Zhang, B. Cai, Y. Gu, J. Song and H. Zeng, Adv. Funct. Mater., 2016, 26, 2435–2445 CrossRef CAS.
- M. Chen, Y. Zou, L. Wu, Q. Pan, D. Yang, H. Hu, Y. Tan, Q. Zhong, Y. Xu, H. Liu, B. Sun and Q. Zhang, Adv. Funct. Mater., 2017, 27, 1701121 CrossRef.
- J. Pan, Y. Q. Shang, J. Yin, M. De Bastiani, W. Peng, I. Dursun, L. Sinatra, A. M. El-Zohry, M. N. Hedhili, A. H. Emwas, O. F. Mohammed, Z. J. Ning and O. M. Bakr, J. Am. Chem. Soc., 2018, 140, 562–565 CrossRef CAS PubMed.
- B. Jeong, H. Han, Y. J. Choi, S. H. Cho, E. H. Kim, S. W. Lee, J. S. Kim, C. Park, D. Kim and C. Park, Adv. Funct. Mater., 2018, 28, 1706401 CrossRef.
- C. X. Bao, Z. L. Chen, Y. J. Fang, H. T. Wei, Y. H. Deng, X. Xiao, L. L. Li and J. S. Huang, Adv. Mater., 2017, 29, 1703209 CrossRef PubMed.
- Y. W. Wang, Y. H. Zhu, J. F. Huang, J. Cai, J. R. Zhu, X. L. Yang, J. H. Shen, H. Jiang and C. Z. Li, J. Phys. Chem. Lett., 2016, 7, 4253–4258 CrossRef CAS PubMed.
- N. G. Park, M. Gratzel, T. Miyasaka, K. Zhu and K. Emery, Nat. Energy, 2016, 1, 16152 CrossRef CAS.
- Q. A. Akkerman, M. Gandini, F. Di Stasio, P. Rastogi, F. Palazon, G. Bertoni, J. M. Ball, M. Prato, A. Petrozza and L. Manna, Nat. Energy, 2017, 2, 16194 CrossRef CAS.
- Z. L. Chen, Q. F. Dong, Y. Liu, C. X. Bao, Y. J. Fang, Y. Lin, S. Tang, Q. Wang, X. Xiao, Y. Bai, Y. H. Deng and J. S. Huang, Nat. Commun., 2017, 8, 1890 CrossRef PubMed.
- V. A. Vlaskin, C. J. Barrows, C. S. Erickson and D. R. Gamelin, J. Am. Chem. Soc., 2013, 135, 14380–14389 CrossRef CAS PubMed.
- D. Mocatta, G. Cohen, J. Schattner, O. Millo, E. Rabani and U. Banin, Science, 2011, 332, 77–81 CrossRef CAS PubMed.
- Z. J. Li, E. Hofman, A. Blaker, A. H. Davis, B. Dzikovski, D. K. Ma and W. Zheng, ACS Nano, 2017, 11, 12591–12600 CrossRef CAS PubMed.
- W. Zheng, K. Singh, Z. Wang, J. T. Wright, J. van Tol, N. S. Dalal, R. W. Meulenberg and G. F. Strouse, J. Am. Chem. Soc., 2012, 134, 5577–5585 CrossRef CAS PubMed.
- N. Pradhan and X. G. Peng, J. Am. Chem. Soc., 2007, 129, 3339–3347 CrossRef CAS PubMed.
- A. Sahu, M. S. Kang, A. Kompch, C. Notthoff, A. W. Wills, D. Deng, M. Winterer, C. D. Frisbie and D. J. Norris, Nano Lett., 2012, 12, 2587–2594 CrossRef CAS PubMed.
- W. W. Zheng and G. F. Strouse, J. Am. Chem. Soc., 2011, 133, 7482–7489 CrossRef CAS PubMed.
- J. H. Yu, X. Y. Liu, K. E. Kweon, J. Joo, J. Park, K. T. Ko, D. Lee, S. P. Shen, K. Tivakornsasithorn, J. S. Son, J. H. Park, Y. W. Kim, G. S. Hwang, M. Dobrowolska, J. K. Furdyna and T. Hyeon, Nat. Mater., 2010, 9, 47–53 CrossRef CAS PubMed.
- F. P. Zhu, Z. J. Yong, B. M. Liu, Y. M. Chen, Y. Zhou, J. P. Ma, H. T. Sun and Y. Z. Fang, Opt. Express, 2017, 25, 33283–33289 CrossRef CAS.
- R. Begum, M. R. Parida, A. L. Abdelhady, B. Murali, N. M. Alyami, G. H. Ahmed, M. N. Hedhili, O. M. Bakr and O. F. Mohammed, J. Am. Chem. Soc., 2017, 139, 731–737 CrossRef CAS PubMed.
- C. H. Bi, S. X. Wang, Q. Li, S. V. Kershaw, J. J. Tian and A. L. Rogach, J. Phys. Chem. Lett., 2019, 10, 943–952 CrossRef CAS PubMed.
- Z. J. Yong, S. Q. Guo, J. P. Ma, J. Y. Zhang, Z. Y. Li, Y. M. Chen, B. B. Zhang, Y. Zhou, J. Shu, J. L. Gu, L. R. Zheng, O. M. Bakr and H. T. Sun, J. Am. Chem. Soc., 2018, 140, 9942–9951 CrossRef CAS PubMed.
- S. Das Adhikari, S. K. Dutta, A. Dutta, A. K. Guria and N. Pradhan, Angew. Chem., Int. Ed., 2017, 56, 8746–8750 CrossRef CAS PubMed.
- H. Liu, Z. Wu, J. Shao, D. Yao, H. Gao, Y. Liu, W. Yu, H. Zhang and B. Yang, ACS Nano, 2017, 11, 2239–2247 CrossRef CAS PubMed.
- D. Parobek, Y. Dong, T. Qiao and D. H. Son, Chem. Mater., 2018, 30, 2939–2944 CrossRef CAS.
- K. Xu and A. Meijerink, Chem. Mater., 2018, 30, 5346–5352 CrossRef CAS PubMed.
- D. Parobek, B. J. Roman, Y. Dong, H. Jin, E. Lee, M. Sheldon and D. H. Son, Nano Lett., 2016, 16, 7376–7380 CrossRef CAS PubMed.
- K. Xu, C. C. Lin, X. Xie and A. Meijerink, Chem. Mater., 2017, 29, 4265–4272 CrossRef CAS PubMed.
- J. Zhu, X. Yang, Y. Zhu, Y. Wang, J. Cai, J. Shen, L. Sun and C. Li, J. Phys. Chem. Lett., 2017, 8, 4167–4171 CrossRef CAS PubMed.
- W. Liu, Q. Lin, H. Li, K. Wu, I. Robel, J. M. Pietryga and V. I. Klimov, J. Am. Chem. Soc., 2016, 138, 14954–14961 CrossRef CAS PubMed.
- G. G. Huang, C. L. Wang, S. H. Xu, S. F. Zong, J. Lu, Z. Y. Wang, C. G. Lu and Y. P. Cui, Adv. Mater., 2017, 29, 1700095 CrossRef PubMed.
- X. Yuan, S. H. Ji, M. C. De Siena, L. L. Fei, Z. Zhao, Y. J. Wang, H. B. Li, J. L. Zhao and D. R. Gamelin, Chem. Mater., 2017, 29, 8003–8011 CrossRef CAS.
- W. van der Stam, J. J. Geuchies, T. Altantzis, K. H. W. van den Bos, J. D. Meeldijk, S. Van Aert, S. Bals, D. Vanmaekelbergh and C. D. Donega, J. Am. Chem. Soc., 2017, 139, 4087–4097 CrossRef CAS PubMed.
- T. Lei, M. L. Lai, Q. Kong, D. Y. Lu, W. Lee, L. T. Dou, V. Wu, Y. Yu and P. D. Yang, Nano Lett., 2018, 18, 3538–3542 CrossRef CAS PubMed.
- M. Saliba, T. Matsui, K. Domanski, J. Y. Seo, A. Ummadisingu, S. M. Zakeeruddin, J. P. Correa-Baena, W. R. Tress, A. Abate, A. Hagfeldt and M. Gratzel, Science, 2016, 354, 206–209 CrossRef CAS PubMed.
- G. C. Pan, X. Bai, D. W. Yang, X. Chen, P. T. Jing, S. N. Qu, L. J. Zhang, D. L. Zhou, J. Y. Zhu, W. Xu, B. Dong and H. W. Song, Nano Lett., 2017, 17, 8005–8011 CrossRef CAS PubMed.
- Q. S. Hu, Z. Li, Z. F. Tan, H. B. Song, C. Ge, G. D. Niu, J. T. Han and J. Tang, Adv. Opt. Mater., 2018, 6, 1700864 CrossRef.
- J. S. Yao, J. Ge, B. N. Han, K. H. Wang, H. B. Yao, H. L. Yu, J. H. Li, B. S. Zhu, J. Z. Song, C. Chen, Q. Zhang, H. B. Zeng, Y. Luo and S. H. Yu, J. Am. Chem. Soc., 2018, 140, 3626–3634 CrossRef CAS PubMed.
- W. Zhai, J. Lin, Q. L. Li, K. Zheng, Y. Huang, Y. Z. Yao, X. He, L. L. Li, C. Yu, C. Liu, Y. Fang, Z. Y. Liu and C. C. Tang, Chem. Mater., 2018, 30, 3714–3721 CrossRef CAS.
- W. J. Mir, M. Jagadeeswararao, S. Das and A. Nag, ACS Energy Lett., 2017, 2, 537–543 CrossRef CAS.
- Z. J. Li, E. Hofman, A. H. Davis, A. Khammang, J. T. Wright, B. Dzikovski, R. W. Meulenberg and W. Zheng, Chem. Mater., 2018, 30, 6400–6409 CrossRef CAS.
- D. D. Zhang, S. W. Eaton, Y. Yu, L. T. Dou and P. D. Yang, J. Am. Chem. Soc., 2015, 137, 9230–9233 CrossRef CAS PubMed.
- E. Hofman, R. J. Robinson, Z. J. Li, B. Dzikovski and W. W. Zheng, J. Am. Chem. Soc., 2017, 139, 8878–8885 CrossRef CAS.
- K. F. Wu, G. J. Liang, Q. Y. Shane, Y. P. Ren, D. G. Kong and T. Q. Lian, J. Am. Chem. Soc., 2015, 137, 12792–12795 CrossRef CAS.
- S. Mahamuni, A. D. Lad and S. Patole, J. Phys. Chem. C, 2008, 112, 2271–2277 CrossRef CAS.
- D. J. Norris, N. Yao, F. T. Charnock and T. A. Kennedy, Nano Lett., 2001, 1, 3–7 CrossRef CAS.
- A. Z. Pan, B. He, X. Y. Fan, Z. K. Liu, J. J. Urban, A. P. Alivisatos, L. He and Y. Liu, ACS Nano, 2016, 10, 7943–7954 CrossRef CAS PubMed.
Footnote |
† Electronic supplementary information (ESI) available. See DOI: 10.1039/c9ra08289a |
|
This journal is © The Royal Society of Chemistry 2019 |
Click here to see how this site uses Cookies. View our privacy policy here.