DOI:
10.1039/C9RA07262D
(Paper)
RSC Adv., 2019,
9, 36366-36373
Retracted Article: The protective effect of propofol on ionizing radiation-induced hematopoietic system damage in mice†
Received
10th September 2019
, Accepted 26th October 2019
First published on 8th November 2019
Abstract
The hematopoietic system is highly sensitive to ionizing radiation (IR), and IR can cause injury to hematopoietic stem cells (HSCs); the main reason for this may be elevated reactive oxygen species (ROS) levels. Propofol is an anesthetic drug commonly used in clinical practice. The chemical structure of propofol is similar to that of vitamin E, and propofol has an antioxidant capacity. Therefore, in this work the effect of using propofol to protect against IR-induced hematopoietic system injury is evaluated. The data suggested that when the irradiated mice were treated with 20 mg kg−1 of propofol, the survival rate of lethally irradiated mice increased significantly, furthermore, the radiation-induced decrease of white blood cells (WBCs), red blood cells (RBCs), hemoglobin (HGC) and platelets (PLT) in peripheral blood is improved significantly. In addition, propofol could also increase the irradiated HSC and hematopoietic progenitor cell (HPC) frequencies, improving the self-renewal and differentiation abilities of HSCs and HPCs in irradiated mice. Next the ROS levels in HSCs and HPCs were measured, and the results showed that propofol could effectively decrease the ROS levels in these cells. The underlying ROS-scavenging mechanisms are further explored, and the results show that the Nrf2 pathway plays an important role in propofol's radiation protective effects, however, propofol can also increase the proliferation of the Nrf2 inhibitor-treated Lineage− cells after exposure to 4 Gy radiation. The data suggest that propofol has a radio-protective effect against IR-induced hematopoietic system damage through reducing cellular ROS in HSCs and HPCs partly through the Nrf2 pathway.
1 Introduction
The hematopoietic system is highly sensitive to ionizing radiation (IR) and it has been reported that IR at a very low dose (about 0.5 Gy) could cause injury to the hematopoietic system.1,2 Reactive oxygen species (ROS) are the main reason for radiation-induced hematopoietic system injury, because normally, hematopoietic stem/progenitor cells (HSPCs) reside in a low oxygen environment, so that elevated ROS may damage the self-renewal and differentiation ability of HSPCs in irradiated mice. It has been found that ROS-scavenging compounds such as Metformin and Resveratrol can alleviate radiation-induced HSPC injury.3,4
Propofol (2,6-diisopropylphenol) is an anesthetic drug often used in clinical practice that works rapidly after intravenous administration to the human body. Propofol has many advantages such as few side effects, control of anesthesia, rapid onset, and quick recovery. Consequently, it has been widely used for clinical anesthesia induction and maintenance. In addition to anesthesia, it has been reported that propofol has anti-anxiety, anti-oxidation, immune regulation, nervous system protection, antiemetic, and analgesic effects,5–8 which significantly improve the clinical application of propofol. The chemical structure of propofol is similar to that of vitamin E, and its phenols give the antioxidant activity. Therefore, in patients who require surgery after clinical stress or tumor radiotherapy, propofol anesthesia may be able to reduce their oxidative stress appropriately. However, it is unclear whether propofol can protect against IR-induced hematopoietic system damage.
In this study, the radio-protective effect of propofol on mice who had hematopoietic system damage induced by whole-body irradiation (WBI) was explored. The results suggested that propofol effectively protected mice against WBI-induced hematopoietic system injury, furthermore, propofol could also significantly improve the self-renewal and differentiation ability of the irradiated mice. The Nrf2-mediated ROS scavenging contributed to this radiation protective effect of propofol. This research provides the basis for expanding the clinical application of propofol and deserves further study.
2 Materials and methods
2.1 Experimental animals
All the male ICR mice used in the experiment were purchased from the Beijing Vital River Experimental Animal Co., Ltd. Male C57BL/6 (CD45.1) mice were purchased from the College of Medicine, Peking University, and male C57BL/6 (CD45.1/CD45.2) mice were bred in the animal house of the North China University of Science and Technology. The Animal Care and Ethics Committee of North China University of Science and Technology approved the use of animals for these experiments, which complied with the Guide for the Care and Use of Laboratory Animals and the National Institutes of Health Guide for the Care and Use of Laboratory Animals.
2.2 Antibodies
Biotin conjugated anti-mouse CD4 (Ref. 100403, clone GK1.5), biotin conjugated anti-mouse CD8 (Ref. 100703, clone 53-6.7), biotin anti-mouse/human CD45R/B220 (Ref. 103203, clone RA3-6B2), biotin anti-mouse/human CD11b (Ref. 101204, clone M1/70), biotin anti-mouse Gr1 (Ref. 108403, clone RB6-8C5), Biotin anti-mouse Ter-119 (Ref. 116203, clone TER-119), APC anti-mouse CD117 (Ref. 105811, clone 2B8), PE anti-mouse Sca1 (Ref. 122507, clone E13-161.7), and PerCP streptavidin (Ref. 405213, no clone) were purchased from BioLegend (San Diego, CA, USA). Anti-Nrf2 (Ref. ab62353, clone EP1808Y), anti-HO1 (Ref. ab13248, clone HO-1-1), anti-NQO1 (Ref. ab213239, clone EPR21230), goat anti-rabbit H&L IgG FITC (Ref. ab6717, no clone) and recombinant anti-β-actin antibody (Ref. ab179467, clone EPR16769) were purchased from Abcam (Cambridge, UK, and USA).
2.3 Whole-body irradiation (WBI) and propofol administration
There are five groups in the 30 day survival experiment: WBI, WBI + vehicle (normal saline), WBI + 5 mg kg−1 propofol, WBI + 10 mg kg−1 propofol, and WBI + 20 mg kg−1 propofol, and a sublethal radiation dose of 7.5 Gy and a lethal radiation dose of 8 Gy were used in this experiment. The MultiRad 160 X-ray irradiation system (Faxitron, AZ, USA) was used at a dose rate of 1.32 Gy min−1 was used in all the experiments. The dose rate of the machine is verified by an authorized inspector from the Disease Control and Prevention Department every year. The spleen colony-forming experiment was divided into four groups: control, 20 mg kg−1 propofol, 6 Gy WBI, and 6 Gy WBI + 20 mg kg−1 propofol, and the spleens were harvested 10 d after irradiation. There were six groups in the other experiments: control, 20 mg kg−1 propofol, 2 Gy WBI, 2 Gy WBI + 20 mg kg−1 propofol, 4 Gy WBI, 4 Gy WBI + 20 mg kg−1 propofol, and all the mice were sacrificed 15 d after irradiation. A dose of 200 μL propofol or vehicle (normal saline) was administered to the mice by intraperitoneal injection and the irradiated mice received propofol or vehicle 30 min before WBI and then given a daily dose after WBI for 7 d.
2.4 Peripheral blood cell and bone marrow mononuclear cell (BMNC) counts
Blood from a mouse heart was collected into a heparin anticoagulant tube 15 d after radiation. A portion (100 μL) of blood was used for counting the peripheral blood cells with a Hemolyzer (Pulang Biotechnology Co., Ltd., Nanjing, China). Bone marrow cells were flushed from both the tibias and femurs with sterile PBS, and the red blood cells were removed using lysis buffer, and then the number of BMNCs was counted.
2.5 Flow cytometer analysis
In total, 5 × 106 BMCs were stained firstly with a mixture of biotin-conjugated Ter119, B220, Grl, CD11b, CD4, and CD8, and then stained with PerCP-conjugated streptavidin, PE-conjugated Sca1, APC-conjugated c-kit. A BD FACSCalibur flow cytometer (BD Bioscience, San Jose, CA, USA) was used for the detection of hematopoietic stem/progenitor cells (HSPCs), FlowJo software was used for the data analysis.
2.6 Competitive bone marrow transplantation
The recipient mice were exposed to 9 Gy WBI the day before the bone marrow transplantation. A mixture of 1 × 106 BMCs (CD45.2, donor) and 2.5 × 105 CD45.1/45.2-derived BMCs (competitor) was then transplanted into recipient mice (CD45.1) through tail vein injection. Peripheral blood was collected four months after transplantation, CD45.1 and CD45.2 antibodies were used to detect the percentage of donor-derived cells.
2.7 Colony-forming units of granulocytes and macrophages (CFU-GM)
The cell numbers used in this experiment was 1 × 104, 4 × 104, and 1 × 105 BMCs in the control, with 2 Gy or 4 Gy irradiation of each group. The BMCs were cultured in M3534 methylcellulose medium (MethoCult, STEMCELL Technologies, Vancouver, Canada). Following the manufacturer's instructions, the CFU-GM colonies in each group were counted after 5 d.
2.8 Colony-forming units in the spleen (CFU-S)
The spleens of the mice were harvested 10 d after radiation and immersed in picric acid for 2 h, and then the number of nodules on the spleen surface were counted.
2.9 Intracellular reactive oxygen species detection
The BMCs were stained with Lineage− Sca1+ c-kit+ (LSK) cells and HPC markers as described previously and then incubated with a 2,7-dichlorodihydrofluorescein diacetate probe (10 μM, Beyotime, Jiangsu, China) in a water bath for 20 min. The ROS level was determined by measuring the mean fluorescence intensity (MFI) of FITC in both LSKs and HPCs.
2.10 Bone marrow Lineage− cell sorting and immuno-fluorescence staining
The BMCs were first stained with mixed Lineage antibodies (including biotin-conjugated Ter119, B220, CD3, Gr1, CD11b antibodies) and then with stained microbeads (Miltenyi Biotec, Teterow, Germany) which can be conjugated with biotin. The Lineage− cells were then sorted in a QuadroMACS™ Separator (Miltenyi Biotec, Teterow, Germany). The sorted cells were fixed and permeabilized with fixation and permeabilization buffer (BD Bioscience, San Jose, CA, USA) following the manufacturer's instructions, and then stained with Nrf2 (1
:
200) antibody and FITC-labelled secondary antibody. A fluorescence microscope (Leica Microsystems GmbH, Wetzlar, Germany) was used to observe the nuclear translocation of Nrf2 in the cells.
2.11 Western blot analysis
Lineage− cells were lysed in RIPA buffer (Solarbio, Beijing, China) and with phenylmethylsulfonyl fluoride (Sigma, St. Louis, MO, USA). The protein samples were quantified first, and then the same amount protein was loaded into 10% SDS-PAGE, and after the protein was transferred to PVDF membranes (Thermo Scientific, Massachusetts, MA, USA), the membranes were incubated with antibodies against β-actin (1
:
1000), Nrf2 (1
:
1000), HO1 (1
:
1000), NQO1 (1
:
1000). The images of the proteins were captured using a ChemiDoc XRS system (BioRad) and the relative gray value was evaluated with Image J software.
2.12 Lineage− cells culture in vitro
Lineage− cells were isolated as described previously, and then propofol (25 μM, 50 μM, 100 μM, 200 μM, 400 μM) was added to the medium 30 min before irradiation and then cultured for 18 h. To inhibit Nrf2, all-trans retinoic acid (ATRA, 10 μM, 20 μM, 40 μM) (Sigma, St. Louis, MO, USA) or ML385 (1 μM, 2 μM, 4 μM) (Selleck, Houston, TX, USA) was added to the medium 1 h before irradiation. Cells were stained with PI, used for detecting live cells, and the cell cycle, as described previously.9 Determination of cell apoptosis was measured using a BD apoptosis detection kit following the manufacturer's instructions. In brief, 1 × 106 Lineage− cells were incubated with 100 μL of binding buffer containing 5 μL of Annexin V and 5 μL of PI for 15 min at room temperature, and then 200 μL of binding buffer was added to the sample for detection of the cell apoptosis.
2.13 Statistical analysis
All the data were statistically analyzed with GraphPad Prism 7.0 software. Data in the survival experiment were analyzed for statistical significance using a log-rank test, and the multiple tests for differences between two groups were performed. The other results were examined with the Mann–Whitney U test when comparing two groups. The difference was statistically significant at p < 0.05.
3 Results
3.1 Propofol elevates the 30 day survival rate of irradiated mice
According to a previous publication,10 7.5 Gy WBI is the lethal dose for ICR mice, so 7.5 Gy and 8 Gy radiation was used in the 30 day survival experiment. As shown in Fig. 1, after exposure to 7.5 Gy and 8 Gy irradiation, the survival rates in WBI groups were 30% and 0%, and there was no increase in the group in which 5 mg kg−1 of propofol was administered to the mice. When the irradiated mice were treated with 10 mg kg−1 of propofol, the 30 day survival rates were 40% and 0% in the 7.5 Gy and 8 Gy irradiation groups, respectively. When the mice were treated with 20 mg kg−1 propofol, the survival rates were 70% and 30% in the 7.5 Gy and 8 Gy irradiation groups, respectively. The previous results suggest that 20 mg kg−1 of propofol can protect against WBI-induced injury in mice.
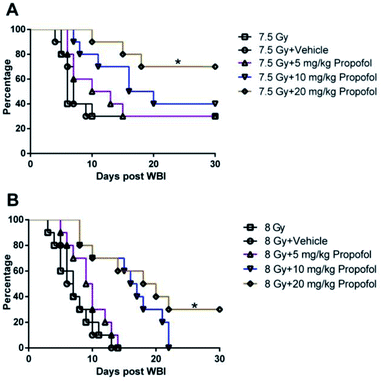 |
| Fig. 1 Propofol increases the survival rate of (A) sub-lethally and (B) lethally irradiated mice. Survival rates were analyzed using a log-rank test (n = 10), then multiple tests for differences between two groups were performed. *p < 0.05 compared with the 7.5 Gy or 8 Gy group. | |
3.2 Propofol alleviates myelosuppression after mice were exposed to WBI
Myelosuppression is the most common symptom in WBI-induced hematopoietic system injury, and presented as leukocyte, erythrocyte, and platelet reduction in peripheral blood.11,12 To determine if propofol can protect WBI-induced myelosuppression in peripheral blood, the numbers of white blood cells (WBCs), red blood cells (RBCs), platelets (PLTs) were counted and the concentration of hemoglobin (HGB) in peripheral blood was measured 15 d after 2 Gy and 4 Gy irradiation. As shown in Fig. 2, compared with the control group, there was a decrease in the number of WBCs, RBCs, PLTs, and the concentration of HGB, and propofol administration increased the blood cell counts as mentioned previously. These results suggested that propofol can alleviate WBI-induced myelosuppression in mice.
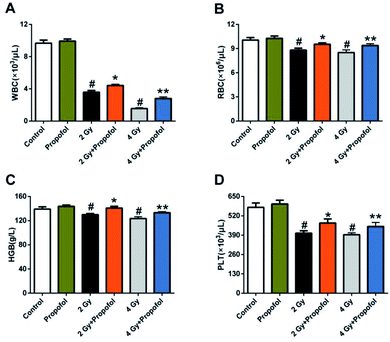 |
| Fig. 2 Propofol mitigates myelosuppression in irradiated mice. The bar graphs show the number of (A) WBCs; and (B) RBCs; the concentration of (C) HGB; and the number of (D) PLTs in peripheral blood. Data are presented as the mean ± SEM (n = 10), #p < 0.05 vs. control, *p < 0.05 vs. 2 Gy, **p < 0.05 vs. 4 Gy. | |
3.3 Propofol attenuates the decrease in BMCs caused by WBI
To explore the radio-protective effect of propofol on the number of BMCs, the number and frequency of BMCs, LSKs, and HPCs in the femur were determined. As shown in Fig. 3, there were decreased BMC, LSK and HPC counts and the percentages of LSK and HPC after the mice were exposed to 2 Gy and 4 Gy WBI were also decreased, and so propofol effectively increased the cell numbers and percentages. Therefore, the results suggested that propofol increases the number and frequency of irradiated BMCs and HSPCs.
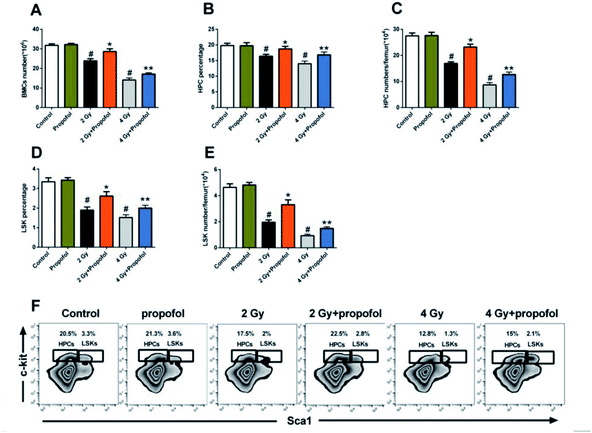 |
| Fig. 3 Propofol promotes the recovery of the BMC number in irradiated mice. (A) Number of BMCs; (B) percentage of HPCs; (C) number of HPCs per femur; (D) percentage of LSKs; (E) number of LSKs per femur; (F) representative flow scatter plots of the LSK and HPC identification. Data are presented as the means ± SEM (n = 10). #p < 0.05 vs. control, *p < 0.05 vs. 2 Gy, **p < 0.05 vs. 4 Gy. | |
3.4 Propofol elevates the proliferative and reconstitution ability of the irradiated mice HSPCs
To determine the radio-protective effect of propofol on the HSPCs' self-renewal and reconstitution ability, the effects on CFU-GM, CFU-S, and bone marrow transplantation were performed in this study. As shown in Fig. 4A, there was a decreased number of CFU-GM in the 2 Gy and 4 Gy WBI groups, and use of propofol can improve this damaged colony-forming ability. Bone marrow transplantation is the gold standard to evaluate the reconstitution ability of HSCs, so the bone marrow transplantation experiment was performed in this study. After mice were exposed to 4 Gy WBI, the engraftment levels of HSC, reflected as donor-derived cells (CD45.2 positive) in peripheral blood, decreased significantly, and propofol treatment increased the reconstitution ability of the donor-derived cells (Fig. 4B and C). In addition, a spleen colony-forming experiment was also performed, and as shown in Fig. 4D and E, no colonies formed in the non-irradiated mice spleen, however, when mice were exposed to 6 Gy irradiation, the number of CFU-S increased significantly, and propofol treatment increased the number of CFU-S in the irradiated mouse spleens. The previous results suggest that propofol promotes recovery of self-renewal and reconstitution ability in irradiated mice HSPCs.
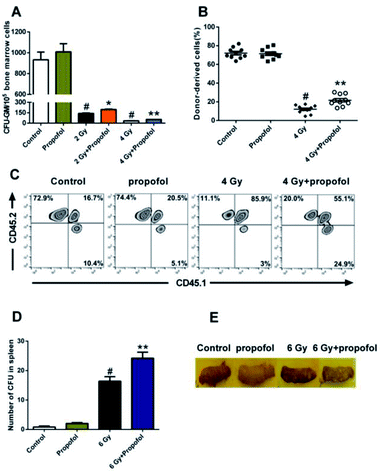 |
| Fig. 4 Propofol improves the self-renewal capacity of irradiated HSPCs. (A) The number of CFU-GM; (B) the percentage of CD45.2+ cells (donor-derived cells) in peripheral blood cells; (C) representative flow scatter plots showing the engraftment of the donor-derived cells; (D) the number of CFU-S; (E) representative images of the nodules formed on the spleen. Data are presented as the means ± SEM (n = 6 in panel A, n = 10 in panel B, n = 5 in panel D), #p < 0.05 vs. control, *p < 0.05 vs. 2 Gy, **p < 0.05 vs. 4 Gy (**p < 0.05 vs. 6 Gy in panel D). | |
3.5 Propofol decreases the percentage of death and apoptosis in irradiated Lineage− cells
To determine the level at which propofol protected against radiation-induced injury, cell death, apoptosis and proliferation were detected using a series of experiments in vitro. As suggested by Yoon et al.13, the doses of propofol used in our experiment were 25, 50, 100, 200, and 400 μM first, as shown in Fig. 5A, and propofol at a dose of 100–200 μM increased the percentage of live cells, and therefore, a dose of 100 μM was used in the following experiments. As shown in Fig. 5C and D, when Lineage− cells were exposed to 4 Gy radiation, the apoptotic cells increased significantly 18 h after irradiation, and propofol decreased the percentage of apoptotic cells. Then, the cell cycle in Lineage− cells was determined, and as shown in Fig. 5B, the number of proliferative cells (S/G2/M phase) decreased after exposure to radiation. However, it seems that propofol did not affect radiation-induced cell cycle arrest. Taken together, the previous data suggested that propofol protects against radiation-induced Lineage− cell damage by reducing cell death and apoptosis.
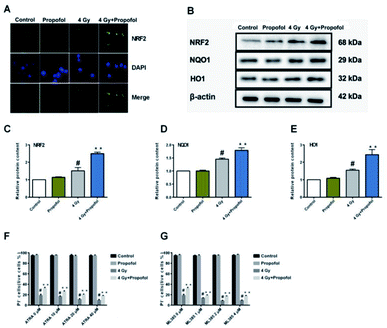 |
| Fig. 5 Propofol inhibits IR-induced cell death and apoptosis. Propofol at concentrations of 25 μM, 50 μM, 100 μM, 200 μM, and 400 μM was added to the culture medium 30 min before Lineage− cells were exposed to 4 Gy, and then cell death, apoptosis and cell cycle analyses were performed. (A) The percentage of live cells; (B) the percentage of proliferative (S/G2/M phase) cells; (C) the percentage of apoptotic cells; (D) representative flow scatter plots of cell apoptosis. Data are presented as means ± SEM (n = 5), #p < 0.05 vs. control, **p < 0.05 vs. 4 Gy. | |
3.6 Propofol decreases ROS levels in HSPCs after mice were exposed to WBI
ROS has been considered as the main reason for WBI-induced hematopoietic system injury, and consequently, it was necessary to know whether propofol could scavenge ROS in HSPCs. As shown in Fig. 6, there were increased ROS levels in 2 Gy and 4 Gy irradiated LSKs and HPCs, and propofol reduced these ROS levels. The previous data indicated that propofol could protect against IR-induced hematopoietic system damage by scavenging cellular ROS.
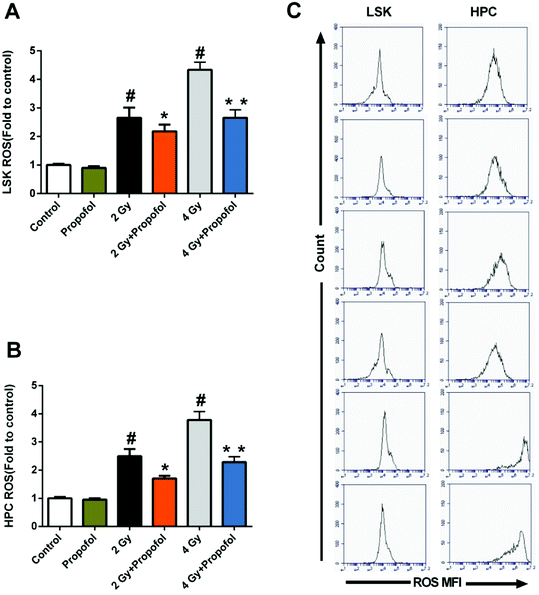 |
| Fig. 6 Propofol decreases ROS levels in irradiated HSPCs. (A) ROS levels in LSK cells; (B) ROS levels in HPC cells; (C) representative flow images of cellular ROS. Data are presented as the means ± SEM (n = 5), #p < 0.05 vs. control, *p < 0.05 vs. 2 Gy, **p < 0.05 vs. 4 Gy. | |
3.7 Propofol exerts antioxidant ability partly by activating the Nrf2 signaling pathway
The Nrf2 is a core factor in the regulation of endogenous redox homeostasis,14 and it has been reported that propofol can reduce the ROS level in transplanted livers and then alleviate liver damage15 through Nrf2 pathways. To investigate the mechanism of propofol's radio-protective effect, Nrf2 expression at the protein level and the targeted downstream proteins in mouse Lineage− cells were determined. As shown in Fig. 7A, Nrf2 was transferred to the Lineage− cell nucleus after irradiation, and there was more Nrf2 accumulated in the propofol-treated irradiated cells. Next the relative expression of Nrf2 and downstream proteins, NQO1 and HO1 was determined, and these proteins were up-regulated after mice were exposed to 4 Gy irradiation, and further up-regulated after propofol treatment (Fig. 7B–E). These data showed that propofol might exert an antioxidant effect by activating the Nrf2 pathway. To further illustrate the effect of Nrf2 mediation on propofol's radiation protection, ATRA and ML385 were used to first inhibit Nrf2 and then then the radiation-induced cell injury in vitro was determined. As described in the work of Kim et al.16, 20 μM ATRA down-regulated the expression of Nrf2 in ovarian cancer cells, therefore, the concentrations of ATRA used in this experiment were 10 μM, 20 μM, and 40 μM. The ML385 is a novel and specific Nrf2 inhibitor with an IC50 of 1.9 μM, and thus, the concentrations used in this study were 1 μM, 2 μM, and 4 μM. Lineage− cells were isolated to do these experiments, and as shown in a ESI figure,† the purity of the Lineage− cells was 94%. Propofol could still increase the percentage of the retinoic acid-treated and ML385-treated cells after exposure to 4 Gy radiation (Fig. 7F and G), and the results, as mentioned previously, suggested that Nrf2 is not the unique mechanism that mediated propofol's radio-protective effect.
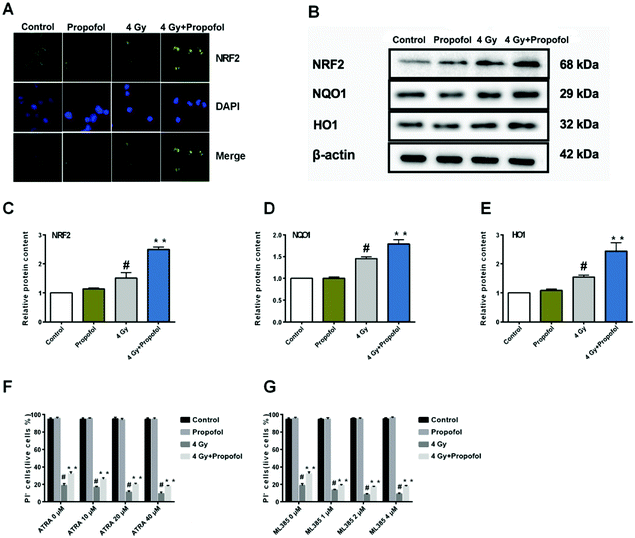 |
| Fig. 7 Propofol up-regulates the Nrf2 pathway in irradiated mouse Lineage− cells. (A) Representative images showing Nrf2 localization in Lineage− cells; (B) representative WB images showing Nrf2, HO1 and NQO1 expression; (C) relative quantification of NRF2 in Lineage− cells; (D) relative quantification of HO1 in Lineage− cells; (E) relative quantification of NQO1 in Lineage− cells. Data are presented as the means ± SEM (n = 3); (F) percentages of live cells in ATRA and radiation-treated Lineage− cells; (G) percentages of live cells in ML385 and radiation-treated Lineage− cells (n = 5); #p < 0.05 vs. control, **p < 0.05 vs. 4 Gy. | |
4 Discussion
The hematopoietic system was sensitive to ionizing radiation, and a dose higher than 0.5 Gy can damage HSPCs, which ultimately induces hematopoietic system aging and leukemia.1 Propofol is widely used as a clinical anesthetic, but its non-anesthetic effects have also been reported. Studies have shown that propofol can inhibit ephedrine-induced oxidative damage, mitochondrial function decline, and neurotoxicity in the rat brain.8 Propofol can also decrease ROS levels in a myocardial ischemia-reperfusion injury model in vitro and alleviate myocardial injury caused by postreperfusion.17,18 Moreover, propofol attenuates intestinal ischemia-reperfusion injury by scavenging ROS,7 improves Parkinson's disease by activating antioxidant enzyme activity, and inhibits lipid peroxidation in heart, liver, kidney, and lung tissue.19 In summary, the anti-oxidative effect of propofol protects against a variety of tissue damage caused by oxidative stress. However, it is still unknown if propofol can protect against hematopoietic damage caused by IR.
The results of this work showed that propofol could protect against WBI-induced hematopoietic system damage, increase the 30 day survival rate of lethally irradiated mice, alleviate myelosuppression, and improve the irradiated HSC self-renewal and reconstitution ability. However, results of secondary bone marrow transplantation assay (data not shown) indicated that, propofol increased the percentage of donor-derived cells, but it was very limited and there was no significant difference between the radiation and propofol treated group, which suggested that propofol could not improve the long-term self-renewal ability of irradiated HSCs. The potent dose of propofol in the experiments was 20 mg kg−1, when converted to humans, and the effective dose was approximately 1.63 mg kg−1;20 and this dose is very close to the dose of 2 mg kg−1 used in humans for anesthesia. Zeller et al. reported that, after iv injection of 40 mg kg−1 of propofol, there was the anesthetic-induced respiratory and heart rate depression in wild-type mice.21 Propofol has many advantages such as few side effects, control of anesthesia, rapid onset, and quick recovery. Amifostine is the only drug approved by the FDA for use in clinical practice to protect radiation-induced injury, however, there are severe side effects such as hypotension, fatigue, and somnolence, which leads to drug termination in 15–20% of all patients.22 Thus, compared to amifostine, propofol may be more suitable for use as a radio-protective drug. However, it is not known if propofol can work on radiation-induced injury in different tissues and organs in mice or the effect in other animal species and humans. Consequently, further work is needed to demonstrate the radio-protective effects of propofol.
In this study, propofol strongly inhibited ROS production in irradiated LSKs and HPCs. However, the recovery of number and reconstitution in HSCs and HPCs was limited. Radiation may induce a combined effect, including direct and indirect injury to HSCs and HPCs. Although propofol could scavenge ROS significantly, it might not prevent other kinds of damage, and propofol could prevent cell death and apoptosis, but not the recovery of HSPCs.
The underlying radio-protective mechanisms were explored further, and consistent with previous studies,7,17,18 propofol decreased the ROS levels in LSKs and HPCs. It has been reported that propofol can alleviate transplanted liver damage15 through the Nrf2 pathways. Therefore, protein expression in the Nrf2 pathway was determined, and the results suggested that propofol can scavenge ROS by up-regulating Nrf2, HO1 and NQO1 proteins. However, it seems that Nrf2 is not the only pathway through which propofol worked. When the Nrf2 was blocked using the inhibitor ATRA and ML385 in vitro, propofol could also partially protect against radiation-induced injury in Lineage− cells. Therefore, further work is needed to explore the underlying mechanisms.
5 Conclusions
In conclusion, this research shows that propofol can protect WBI-induced hematopoietic system injury by regulating Nrf2 and the downstream proteins. Propofol protects HSCs against IR, but the question remains as to whether propofol increases the incidence of radiation-induced tumors. It has been reported that propofol can inhibit tumor growth in a series of cancer cell lines, such as gastric cancer,23 colorectal cancer,24 pancreatic cancer,25 prostate cancer26 and cervical cancer,27 and thus, propofol is likely to reduce the tumor incidence in irradiated mice while alleviating radiation-induced hematopoietic system injury. Therefore, propofol is a promising new type of radio-protective drug and deserves to be explored further.
Conflicts of interest
There are no conflicts to declare.
Abbreviations
IR | Ionizing radiation |
HSPCs | Hematopoietic stem/progenitor cells |
ROS | Reactive oxygen species |
WBI | Whole-body irradiation |
HSCs | Hematopoietic stem cells |
HPCs | Hematopoietic progenitor cells |
CFU-GM | Colony-forming units of granulocytes and macrophages |
CFU-S | Colony-forming units in the spleen |
WBCs | White blood cells |
RBCs | Red blood cells |
PLTs | Platelets |
HGB | Hemoglobin |
ATRA | All-trans retinoic acid |
BMNCs | Bone marrow mononuclear cells |
LSK | Lineage− Sca1+ c-kit+ |
Acknowledgements
This study was supported by the Key Project Plan of Medical Science Research in Hebei Province (20180774).
Notes and references
- L. Shao, Y. Luo and D. Zhou, Antioxid. Redox Signaling, 2014, 20, 1447–1462 CrossRef CAS.
- J. Zhang, X. Xue, X. Han, Y. Li, L. Lu, D. Li and S. Fan, Oxid. Med. Cell. Longevity, 2017, 2017, 8241678 Search PubMed.
- G. Xu, H. Wu, J. Zhang, D. Li, Y. Wang, Y. Wang, H. Zhang, L. Lu, C. Li, S. Huang, Y. Xing, D. Zhou and A. Meng, Free Radical Biol. Med., 2015, 87, 15–25 CrossRef CAS.
- H. Zhang, Z. Zhai, Y. Wang, J. Zhang, H. Wu, Y. Wang, C. Li, D. Li, L. Lu, X. Wang, J. Chang, Q. Hou, Z. Ju, D. Zhou and A. Meng, Free Radical Biol. Med., 2013, 54, 40–50 CrossRef CAS.
- T. Kambara, T. Inada, K. Kubo and K. Shingu, Immunopharmacol. Immunotoxicol., 2009, 31, 117–126 CrossRef CAS.
- T. Inada, K. Kubo and K. Shingu, Immunopharmacol. Immunotoxicol., 2009, 31, 150–157 CrossRef CAS.
- X. Gan, D. Xing, G. Su, S. Li, C. Luo, M. G. Irwin, Z. Xia, H. Li and Z. Hei, Oxid. Med. Cell. Longevity, 2015, 2015, 167014 Search PubMed.
- M. Shokrzadeh, E. Zamani, M. Mehrzad, Y. Norian and F. Shaki, Toxicol. Int., 2015, 22, 92–99 CAS.
- X. Liu, B. Zhang, Y. Guo, Q. Liang, C. Wu, L. Wu, K. Tao, G. Wang and J. Chen, PLoS One, 2012, 7, e37096 CrossRef CAS.
- D. Li, L. Lu, J. Zhang, X. Wang, Y. Xing, H. Wu, X. Yang, Z. Shi, M. Zhao, S. Fan and A. Meng, Int. J. Mol. Sci., 2014, 15, 10541–10553 CrossRef CAS.
- X. L. Xue, X. D. Han, Y. Li, X. F. Chu, W. M. Miao, J. L. Zhang and S. J. Fan, Stem Cell Res. Ther., 2017, 8, 7 CrossRef.
- X. Han, X. Xue, Y. Zhao, Y. Li, W. Liu, J. Zhang and S. Fan, Int. J. Mol. Sci., 2017, 18, 1–20 Search PubMed.
- J. Y. Yoon, C. W. Baek, E. J. Kim, B. S. Park, S. B. Yu, J. U. Yoon and E. N. Kim, J. Dent. Anesth. Pain. Med., 2017, 17, 37–46 CrossRef.
- Q. Ma, Annu. Rev. Pharmacol. Toxicol., 2013, 53, 401–426 CrossRef CAS.
- M. Ge, W. Yao, Y. Wang, D. Yuan, X. Chi, G. Luo and Z. Hei, J. Surg. Res., 2015, 196, 373–381 CrossRef CAS PubMed.
- D. Kim, B. H. Choi, I. G. Ryoo and M. K. Kwak, Cell Death Dis., 2018, 9, 896 CrossRef.
- X. Yu, X. Sun, M. Zhao, Y. Hou, J. Li, J. Yu and Y. Hou, Int. Immunopharmacol., 2018, 54, 267–274 CrossRef CAS.
- Y. Wang, X. Qi, C. Wang, D. Zhao, H. Wang and J. Zhang, Biomed. Rep., 2017, 6, 69–74 CrossRef CAS.
- E. Romuk, W. Szczurek, P. Nowak, I. Kwiecien, D. Stolecka and E. Birkner, Postepy Hig. Med. Dosw., 2015, 69, 661–667 CrossRef.
- A. B. Nair and S. Jacob, J. Basic Clin. Pharm., 2016, 7, 27–31 CrossRef.
- A. Zeller, M. Arras, A. Lazaris, R. Jurd and U. Rudolph, FASEB J., 2005, 19, 1677–1679 CrossRef CAS.
- M. I. Koukourakis and E. Maltezos, Anticancer Drugs, 2006, 17, 133–138 CrossRef CAS.
- W. Zhang, Y. Wang, Z. Zhu, Y. Zheng and B. Song, Int. J. Biol. Macromol., 2018, 120, 975–984 CrossRef CAS.
- D. Liu, X. Sun, Y. Du and M. Kong, Med. Sci. Monit., 2018, 24, 6119–6128 CrossRef CAS PubMed.
- X. Chen, Q. Wu, L. You, S. Chen, M. Zhu and C. Miao, Eur. J. Pharmacol., 2017, 795, 150–159 CrossRef CAS.
- K. Tatsumi, A. Hirotsu, H. Daijo, T. Matsuyama, N. Terada and T. Tanaka, Eur. J. Pharmacol., 2017, 809, 242–252 CrossRef CAS.
- H. Li, Y. Lu, Y. Pang, M. Li, X. Cheng and J. Chen, Biomed. Pharmacother., 2017, 86, 324–333 CrossRef CAS.
Footnote |
† Electronic supplementary information (ESI) available. See DOI: 10.1039/c9ra07262d |
|
This journal is © The Royal Society of Chemistry 2019 |
Click here to see how this site uses Cookies. View our privacy policy here.