DOI:
10.1039/C9RA05868K
(Paper)
RSC Adv., 2019,
9, 30760-30767
Effects of nanobubble water on the growth of Lactobacillus acidophilus 1028 and its lactic acid production†
Received
29th July 2019
, Accepted 2nd September 2019
First published on 30th September 2019
Abstract
Nanobubble water (NBW) has been applied in various fields due to the unique properties of nanobubbles (NBs) including long-term stability, negative zeta potential and generation of free radicals. In this study, the performance of four kinds of NBW from different gases (air, N2, H2, and CO2) in addition to deionized water (DW) were investigated and compared in terms of the growth of the probiotic Lactobacillus acidophilus 1028. The NB density, size distribution, zeta potential, pH and dissolved oxygen (DO) of the NBW were firstly investigated. Results indicate that N2-NBW had the highest absolute value of zeta potential and NB density (−25.3 ± 5.43 mV and 5.73 ± 1.0 × 107 particles per mL, respectively), while the lowest was detected in CO2-NBW (−6.96 ± 2.36 mV and 3.39 ± 1.73 × 107 particles per mL, respectively). With the exception of CO2-NBW, all the other types of NBW showed promotion effects on the growth of the strain at the lag and logarithmic phases. Among them, N2-NBW demonstrated the best performance, achieving the highest increase ratio of 51.1% after 6 h cultivation. The kinetic models (Logistic and Gompertz) indicate that the culture with N2-NBW had the shortest lag phase and the maximum specific growth rate when compared to the H2-NBW and DW groups under the same cultivation conditions. Preliminary analysis on the mechanisms suggested that these effects were related to the properties (zeta potential and density) of the NBs, which might affect the transport of substances. This study suggests that NBW has the potential for promoting the production efficiency of probiotics via fermentation.
Introduction
Probiotics that exist in a variety of environments from the human gastrointestinal tract to dairy products can confer a health benefit to the host when administered in adequate amounts.1–3 With increasing disposable income and lifestyle changes, customers' growing awareness of the role of probiotics in health maintenance contributes a lot to the amplification of the probiotics market. It has been valued at approximately USD 40.09 billion in 2017 and is expected to generate revenue of around USD 65.87 billion by the end of 2024.4 Thus, it is urgent to improve the yield of probiotics to satisfy the increasing demand of the market. From ancient fermentation foods to the current industrial production of probiotic products, they are almost exclusively produced through fermentation technologies.5 Microbial fermentation is a process in which the strain, culture medium, reactor and culture condition combine and interact with each other. The optimization strategies of probiotics cultivation usually focus on these four aspects to obtain higher biomass or metabolites. Many trials have been attempted in the selection and cultivation of acid/bile-tolerant strains to enhance their survival rate under simulated gastrointestinal environment,6–8 increase the level of inoculum or co-culture to eliminate antagonistic effects of substances.9,10 Besides, there are numerous researches focusing on amelioration of the components of the medium to promote the growth of probiotics11,12 and reduce the cost.13,14 Lactic acid is a major end product of the metabolism of many probiotics, which would inhibit the strain growth with its concentration increase in the batch fermentation.15 Fed-batch and continuous culture systems individually or combined with extractive fermentation approaches can be applied to partially overcome this kind of products inhibition problems to obtain high-cell-density cultivation.15,16 Researchers always paid more attention to the medium pH,17 temperature, dissolved oxygen (DO) and fermentation time during the optimization of operation condition parameters, in order to increase the survival rate of lactic acid bacteria and achieve the best economic benefits.18,19
It is worth noting that water, the major solvent for liquid fermentation is an important growth factor for microorganisms. Water is not only an integral part of biomolecules structure organization but also an essential factor for their functioning. It is inappropriate to discuss biological processes without assessing the role of water.20 However, the effects of water on the growth of probiotics have rarely been addressed. Up to the present, previous reports mainly tried to alter water activity and examined the influence on the growth and metabolism of probiotics instead of improving the properties of water itself.21,22
Nanobubble water (NBW) is produced by mixing the gas with water through different kinds of nanobubble (NB) generators, resulting in the introduced gas existing in the form of suspended nanoscale bubbles in the water. The most promising discovery of NBW is its physiological activity and potential application in biological fields. Recently, many works reported that the application of some kinds of NBW increased the seed germination rate,23–25 promoted the growth of plants,26,27 accelerated the mouse and shellfish growth,27 and inhibited tumour cell development individually or cooperated with other substances.28–30 According to the literature review, the effect of NBW on microorganisms is the major aspect of its biological application, which still has rarely been reported. The available one is relating to the treatment with pathogenic bacteria.29,31
In summary, taking the physiological activities of NBW and the mounting demand of probiotics market into consideration, it is meaningful to examine the effects of NBW on the growth of probiotics. In this study, the properties of four gas types (air, N2, H2 and CO2) NBW were investigated including the NB density, size distribution, zeta potential, pH and DO. Thereafter, the medium with and without NBW addition were applied to explore its effects on the growth of Lactobacillus acidophilus 1028 (LA1028) through evaluating the strain growth, lactic acid production and glucose consumption. To the best of our knowledge, this is the first trial of NBW effects on the growth of probiotics, aiming to amplify the application of NBW in the field of food and medicine.
Materials and methods
Generation of NBW
1.5 L deionized water (DW) was first added into a transparent 2.0 L plastic beaker, which could be recycled through the micro- and nano-bubble generator (HACK FB11, Japan) with the introduction of air (in the laboratory room), CO2, N2, and H2 (with a purity of 99.999%, Taiyo Nippon Sanso Co. Ltd., Japan) individually. And the produced NBW was labelled as Air-NBW, CO2-NBW, N2-NBW, and H2-NBW, respectively. The generator blended the water and gas with high speed to produce NB as previously described.32 To avoid possible impurities from the equipment, the generator was washed with water exchanged as described before the production of test NBW.33 The outlet pressure was kept at 0.25 ± 0.2 MPa to maintain the milky state of the water during a 20 min production duration. After being produced, the beaker with the NBW was statically placed on the table to let the milky water become transparent with visible most microbubbles disappeared while the NB still in the water (as shown in the data below).
NBW properties
The produced NBW was fully filled into a 6 mL glass screw jar and then the bubble size and density were measured with the nanoparticle tracking analysis method using the equipment named NanoSight (NanoSight-LM10, MALVERN, UK). Zeta potential was evaluated by the zeta potential analyser (NanoZS, MALVERN, UK). pH and dissolved oxygen (DO) of NBW were detected by the corresponding meters (METTLER TOLEDO FE20 and HACK HQ40d) respectively. Each test was performed in triplicate.
Strain and medium
Lactobacillus acidophilus 1028 (LA1028) was obtained from the Japan Collection of Microorganisms (JCM). The composition of culture medium was MRS medium (ESI Table S1†).
Strain culture and experimental design
The stock culture of LA1028 kept frozen at −80 °C was activated. Then the bacteria were incubated on the agar plate at 37 °C for 48 h to get the single colony. One colony was chosen to inoculate in the MRS broth and static cultivation at 37 °C for 24 h. After that, 1 mL was inoculated into 100 mL fermentation MRS medium and cultivated at 37 °C for 24 h. The samples were collected in a certain time interval. Biomass was indicated by the optical density at 600 nm (OD600) using the spectrometer (UV1800, Shimadzu, Japan).
Previous report shows that the NB density decreased in 0.02 MPa vacuum pump and water bath at 30 °C.34 To avoid the high temperature and pressure effects on the density of NB in water during the autoclave process, the components of fermentation medium were sterilized separately. The NBW was filtered through the 0.45 μm mixed cellulose ester syringe filter, which was termed as the NB density of filtered NBW as 100%. The sterilized DW was added to the filtered NBW at different volume ratios (30%, 60% and 90%) to make the test water with different NB densities. The concentrated solutions of casein peptone, glucose, beef extract and yeast extract were separately prepared, then sterilized and added into the final medium to get the proper concentration, respectively. The inorganic salts in the MRS medium were mixed together to prepare the concentrated solution and filtered through 0.45 μm syringe filter before being added to the final medium. The growth of LA1028 in the MRS medium with addition of DW and different volume ratios of the four kinds of NBW were recorded.
Lactic acid and glucose concentration
Lactic acid was quantified using the spectrometer method described elsewhere with some modifications.35 A solution of 0.2% iron(III) chloride (FeCl3·6H2O) was firstly prepared. The calibration curve was obtained using lactic acid as standard reagent. 10 g L−1 of lactic acid solution was diluted using DW to 5 g L−1, 2.5 g L−1, 1.25 g L−1, and 0.625 g L−1, respectively, with DW as control. 100 μL solution was vortexed with 4 mL of 0.2% FeCl3·6H2O for 10 s, and then statically stood for reaction for 15 min. The absorbance of the solution was measured at 390 nm by using UV-1800 (Shimadzu, Japan). The samples were measured as the calibration curve method. Glucose concentration was quantified with the dinitro salicylic acid (DNS) method.
Kinetic models
Predictive modelling is promising for the dramatically changing quantitative food microbiology. Models are used to describe the microorganism behaviour under different physical or chemical conditions such as temperature, pH and water activity.36,37 Among the various models applied to the fitting of growth curve, the Logistic and Gompertz model are widely used as the three-parameter model is simpler and easier to use. Their estimates have more degrees of freedom, and it is very important that all the three parameters can be given a biological meaning.37 In this study, the following modified Logistic equation (eqn (1)) and Gompertz equation (eqn (2)) were employed: | 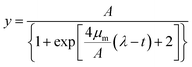 | (1) |
| 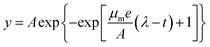 | (2) |
where y is the OD600 value at time t, A is the maximum OD600 value during the fermentation time, μm (h−1) is the maximum specific growth rate, t (h) is the duration of the test, and λ (h) is the length of lag phase that is defined as the delayed period of the strain adapts to the new environment and starts to reproduce. The calculated correlation coefficients (R2) were compared to indicate which kinetic model is the best fitting to the experimental results.
Statistical analysis
All the fermentation experiments were performed in triplicate. The data were presented as mean ± SD and used for results and discussion. All statistical analyses were performed through one-way analysis of variance (ANOVA) with Fisher's least significant difference (LSD) test by using SPSS 19.0. Significant difference was assumed at p < 0.05, while p < 0.01 denoted highly significant difference.
Results and discussion
Nanobubble (NB) and nanobubble water (NBW) properties
The NB density, size and zeta potential, pH and DO of NBW were detected as shown in Table 1. No bubble was detected in DW. The NB density ranged between (1.45–6.73) × 107 particles per mL, with their size distribution at 150–400 nm. The N2-NBW has the highest NB density, which is significantly different from the CO2-NBW and Air-NBW (p < 0.05), while no significant difference in NB density was found between N2-NBW and H2-NBW. These observations were probably brought about by the different solubility of the test gases in the water. As it is known, among the four kinds of gases, CO2 has the highest solubility in water under the same condition, which is followed by air, H2 and N2. Therefore, the higher solubility the gas has, the more volume of gas would dissolve into the water under the same constant gas flowrate during the generation. In addition, bubble breakage and coalescence were also observed during the NB production by the generator, into which the low-pressure gas was injected with water to form NBW by the mechanical vibration and pressure reduction.32,38 The gas from the broken bubbles would continue to dissolve into the water if unsaturated or to mix with water through the generator to produce NB. In this context, less CO2-NB was formed during the operation cycle compared with other three kinds of gases.
Table 1 The NB density, size, zeta potential, pH and DO of NBW prepared with different gasesa
|
DW |
Air-NBW |
N2-NBW |
H2-NBW |
CO2-NBW |
Data are expressed as mean ± SD, and the different letters (a, b, c, d) indicate significant difference at 5% level (p < 0.05). n. d., not detectable.
|
NB density (×107 particle per mL) |
n.d. |
3.59 ± 1.14b |
5.73 ± 1.0a |
4.73 ± 0.35ab |
3.39 ± 1.73b |
NB size (nm) |
n.d. |
199.7 ± 22.1b |
313.0 ± 52.1a |
206.0 ± 26.5b |
230.7 ± 81.2ab |
Zeta potential (mV) |
−0.985 ± 2.28d |
−13.2 ± 1.85b |
−25.3 ± 5.43a |
−16.2 ± 6.35b |
−6.96 ± 2.36c |
pH |
6.97 |
5.48 |
6.58 |
5.15 |
3.92 |
DO (mg L−1) |
8.98 |
6.96 |
1.86 |
3.52 |
5.53 |
Except for the long-term stability of NB in the liquid, one of the most attractive properties of NB is its negatively charged surface. From Table 1, the DW zeta potential is nearly zero while all the four kinds of NBW have negative zeta potential that is in agreement with many reports.33,38–41 The N2-NBW has the highest absolute value of zeta potential ((−25.3 ± 5.43) mV), indicating its highly significant difference from other three types of NBW (p < 0.01). Air-NBW and H2-NBW had similar zeta potentials, (−13.2 ± 1.85) mV and (−16.2 ± 6.35) mV, respectively, while the lowest absolute value of zeta potential, i.e. (−6.96 ± 2.36) mV was detected in the CO2-NBW. Several pervious works claimed that the pH, ion concentration or surfactants could effectively affect the zeta potential value of NBW.39–41 As only different gas was used in the production of the four kinds of NBW and the equipment parameters kept constant during the production, their different zeta potential could be attributed to the different gas type applied. Soluble CO2 in the water would form carbonic acid, which can partially dissociate to give H+ resulting in significantly decreased pH in the water. Previous works on the effects of pH on zeta potential of NB indicate that the absolute value of NB zeta potential would reduce with the decrease of pH value.32,40–45 This might be the main reason for the lowest zeta potential in the CO2-NBW. Overall, the different properties of the four kinds of NBW are associated with the gas type, which is especially affected by the gas solubility.
Effects of NBW type and volume percentage in medium on the growth of LA1028
Fig. 1 shows the growth of LA1028 by using different NBW at different volume percentages in the culture medium. The results show that addition of 30%, 60%, and 90% of Air-NBW could improve the growth of LA1028 during cultivation from 6 h to 18 h (Fig. 1A). According to the statistical analysis, significant difference (p < 0.05) in LA1028 growth was observed between the 90% Air-NBW group and the DW group after cultivation for 6 h, increasing by 21.5% in terms of OD600 value; while the addition of 30% and 60% Air-NBW showed little improvement effect at the same time. However, during the cultivation from 9 h to 15 h, the addition of Air-NBW had obvious improvement effect on LA1028 growth, increasing by 21.6%, 23.8% and 29.7% at 15 h under 30%, 60%, and 90% Air-NBW addition, respectively. Probably the growth of bacteria entered into their stationary phase after 21 h cultivation, little difference was noticed among the test groups.
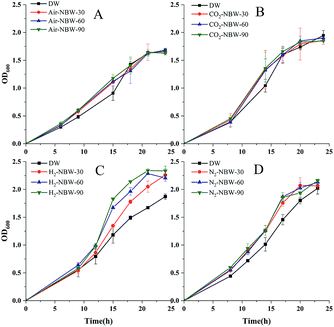 |
| Fig. 1 Growth of LA1028 under the addition of different gas NBW at different volume percentages. (A) Air-NBW, (B) CO2-NBW, (C) H2-NBW, and (D) N2-NBW. Y in NBW-Y denotes the volume percentage of NBW in the test was Y%. | |
Fig. 1B shows the effects of CO2-NBW addition on the stain growth during 24 h cultivation. As it can be seen, almost the same growth trend was observed between the CO2-NBW and Air-NBW tests. In addition, no significant difference was detected among the CO2-NBW test groups during the whole growth phase.
The effect of H2-NBW addition on the strain growth is shown in Fig. 1C. During the initial 9 h cultivation, no significant difference was observed among the test groups; while the strain growth started to accelerate from 12 h on, which continued to increase till 21 h. After cultivation for 21 h, no difference was noticed among the H2-NBW groups, while their strain growth was significantly higher than the DW group. Obviously, the H2-NBW showed better improvement effect on the strain growth, which followed a volume percentage dependent manner. The highest increase ratios under 30%, 60% and 90% of H2-NBW addition were 22.6%, 41.6% and 54.7% in comparison to the DW group, respectively.
N2-NBW also showed promotion effect on the strain growth as shown in Fig. 1D. The N2-NBW group's growth showed highly significant difference (p < 0.01) from the DW group at the beginning of strain growth, which is different from those in Air-NBW and H2-NBW addition tests. However, there was no significant difference between the N2-NBW and DW group tests at stationary phase (after 20 h). Addition of 90% N2-NBW exhibited a highly significant increase (p < 0.01) compared with the 30% N2-NBW addition, which also obviously improved in comparison to the 60% N2-NBW addition after cultivation for 8 h. After that, the N2-NBW addition tests reflected almost similar stain growth till the end of cultivation. Compared with the DW group, the N2-NBW test groups were averagely increased by 23.2%, 25.2% and 29.5% during the period from 8 h to 20 h cultivation when 30%, 60% and 90% of N2-NBW were added into the culture medium, respectively.
The above results show that except CO2-NBW, other three kinds of NBW promoted the growth of LA1028 in a volume percentage dependent manner. More specifically, this promotion effect occurred at the lag and logarithmic phases.
Comparison of effects on the growth and metabolism of LA1028 between N2-NBW and H2-NBW
Taking the effects of the four kinds of NBW on the growth of LA1028 into consideration, H2-NBW and N2-NBW were chosen to conduct this specific study, in which the effects of 90% NBW addition on growth, glucose consumption and lactic acid production of LA1028 were examined.
Fig. 2A show the effects of H2-NBW and N2-NBW addition at 90% volume percentage on the growth of LA1028. The NBW group tests showed growth promotion compared with the DW group, in which N2-NBW demonstrated a better performance than H2-NBW. A highly significant difference (p < 0.01) in the growth was discerned between the tests of N2-NBW and DW addition from 6 h to 15 h. After that, although the OD600 value of N2-NBW group tests were still higher than the DW group, their gap became smaller along with the fermentation progressed and finally to almost no difference at the stationary phase.
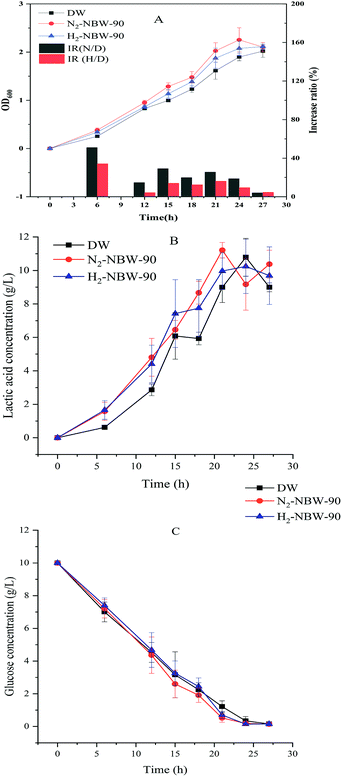 |
| Fig. 2 Comparison between N2-NBW and H2-NBW effects on the growth of LA1028 under the same condition (90% volume percentage). (A) The growth curve, (B) lactic acid concentration, (C) glucose concentration. IR, increase ratio; H/D, H2-NBW/DW test; N/D, N2-NBW/DW test. | |
The N2-NBW group tests achieved the highest increase ratio of 51.1% at 6 h compared with the DW group. Addition of H2-NBW also promoted the growth of LA1028 at 6 h and 15 h (p < 0.05). After that, however, almost no difference was observed between the H2-NBW and DW groups. The H2-NBW group tests obtained the highest increase ratio of 34.2% at 6 h compared with the DW group.
As for the two NBW addition tests, the highest increase ratios were acquired at 6 h, with significantly increased growth of the strain in comparison to the DW group before 15 h, proving again that the effect of NBW addition on the growth of LA1028 mainly occurs at the lag and logarithmic phases. Besides, the bacteria at lag phase mainly adapt to the new environment and prepare enzymes or energy for logarithmic phase, which involves metal ion accumulation as previously described.46 The existence of NB in the water would influence this preparation process as it possesses negative zeta potential. As Table 1 shows, there is no significant difference in NB density between N2-NBW and H2-NBW. Therefore, the significantly higher absolute value of zeta potential of N2-NBW than H2-NBW might be the major reason for their different promotion effects under the same test condition.
Fig. 2B demonstrates the changes of lactic acid concentration during the cultivation. It is observed that NBW addition groups have higher lactic acid production than the DW group during the cultivation, and a significant improvement (p < 0.05) in strain growth was observed between N2-NBW and DW groups from 6 h to 21 h (except at 15 h); while H2-NBW group showed the promotion effect on strain growth only at 6 h. No significant difference in the lactic acid concentration was noticed among the test groups after fermentation for 21 h. As seen, the promotion effects of NBW on the lactic acid production also mainly occurred at the lag and logarithmic phases. From the observation and the strain growth curve, the lag phase period was less than 6 h. The lactic acid concentrations in the DW group at 6 h were significantly lower (p < 0.05) than those in the N2-NBW and H2-NBW groups (0.62 ± 0.12 g L−1, 1.58 ± 0.54 g L−1 and 1.66 ± 0.56 g L−1, respectively).
Theoretically, one mole of glucose can be converted into two moles of lactic acid in homolactic fermentation, and the energy produced during the process can be used for bacterial growth and metabolism. Since the molar mass of glucose is twice that of lactic acid, the concentration of the glucose consumed should be equal to the concentration of lactic acid produced. However, no significant difference was detected in glucose concentration among the test groups during the lag phase and logarithmic phase as shown in Fig. 2C. The carbon source materials used by the strain during the lag phase are mainly converted to the necessary enzymes and energy to supply for the physiological and regulatory processes responsible for adaptation to the new environment.46 These results indicate that with the accumulation of lactic acid at the lag phase, the NBW groups probably produce more energy than the DW group so that the strain growth and reproduction are accelerated. That is, the NBW group could adapt to the new environment faster than the DW group.
Kinetic analysis of the growth of LA1028 in N2-NBW and H2-NBW
The relevant parameters obtained by fitting the modified Logistic and Gompertz models are shown in Table 2. The values of R2 calculated from the Logistic and Gompertz models are both higher than 0.96 and almost equal to each other, indicating that the experimental data well fitted to these two models under the test conditions. From the results of the models, no significant difference has been found in the maximum OD600 value (A); while the duration of lag phase (λ) of N2-NBW group is highly significant shorter than other groups (p < 0.01). In addition, the maximum specific growth rate (μm) of N2-NBW group is significantly higher than the DW group (p < 0.05), while no significant difference is found between N2-NBW and H2-NBW groups. The duration of lag phase (λ) and the maximum specific growth rate (μm) can respectively represent the status of strain growth during the lag phase and logarithmic phase. Therefore, the predicted values from the modified Logistic and Gompertz models also demonstrate that NBW addition could mainly affect the lag phase and logarithmic phase growth of LA1028, and N2-NBW may have the best promotion effect on its growth.
Table 2 Parameters estimated from the modified Logistic and Gompertz models based on OD600 values from the LA1028 fermentation with and without NBW additiona
Condition |
DW |
N2-NBW |
H2-NBW |
Data in the table are expressed as mean ± SD, and different letters (a, b) indicate significant difference at 5% level (p < 0.05).
|
Logistics model
|
A
|
2.33 ± 0.16a |
2.38 ± 0.82a |
2.40 ± 0.12a |
λ (h) |
5.72 ± 0.16a |
5.10 ± 0.16b |
5.60 ± 0.11a |
μ
m (h−1) |
0.11 ± 0.01b |
0.13 ± 0.01a |
0.12 ± 0.14ab |
R
2
|
0.9807 |
0.9654 |
0.9810 |
![[thin space (1/6-em)]](https://www.rsc.org/images/entities/char_2009.gif) |
Gompertz model
|
A
|
2.90 ± 0.30a |
2.72 ± 0.19a |
2.90 ± 0.38a |
λ (h) |
4.55 ± 0.10a |
3.90 ± 0.14b |
4.35 ± 0.14a |
μ
m (h−1) |
0.10 ± 0.06b |
0.12 ± 0.01a |
0.11 ± 0.01ab |
R
2
|
0.9859 |
0.9638 |
0.9809 |
Analysis on the mechanisms
The promotion effects of NBW were mainly observed at lag and logarithmic phases of the strain growth, which was demonstrated by the strain growth, lactic acid production and kinetic analysis. However, what's the mechanism involved in this phenomenon?
The process of strain cultivation is very complex. Currently there is no effective and stable detection methods to determine the change of properties and quantities of NB during strain cultivation because the high conductivity of medium would interfere with the measurements.47 Besides, many particles smaller than NB are also co-existing in the medium. In this study, a preliminary analysis was conducted based on the observed phenomena and theories.
At first, can NB be stably present in the fermentation system? Although the composition of the MRS medium is complex, it can be mainly classified into inorganic salts/acids and organic substances. The colloidal stability of NB in the presence of pH, inorganic salts and normal organic matters has been thoroughly studied and reported. It has been clarified that the NBs have high stability under high ionic strength and surfactant concentration condition.41 Compared with the ionic strength in the experiment (300 mM NaCl), MRS medium has a lower ionic strength (eqn (S1)†). Therefore, theoretically the salts in the MRS medium have less effects on the NB stability. Besides, the organic matters adsorption on the surface of NB could act as a “skin” that enhances its stability as reported elsewhere.48 Moreover, most of the organic components that have charge in the MRS medium mainly belong to proteins, polypeptides and amino acids which normally possess negative charge because of their isoelectric point49 lower than the initial pH 6.5 in the MRS medium. Thus, theoretically the NB could stably present in the MRS medium at the beginning of the cultivation.
Then, Why the promotion effects of NB on the growth of LA 1028 were observed at the lag and logarithmic phases? The possible mechanisms are illustrated in Fig. 3.
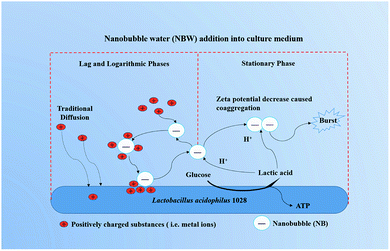 |
| Fig. 3 The possible mechanisms for the promotion effects of NBW on the growth of LA 1028. | |
NB could act as carriers to accelerate the metal ions adsorption onto activated carbon as described elsewhere.50 In their study the tested NB had a negative zeta potential of (−8.6 ± 1) mV. In our study, all the 4 kinds of NBW exhibited negative zeta potential and had the higher absolute value than the reported value (except CO2-NBW) as shown in Table 1. From this viewpoint, the NB in water can attract the positively charged substances such as metal ions in the medium. We propose that the existing NB in the water could offer a new way for the substances to transport into bacteria in addition to the traditional diffusion. It has been described that most Gram-negative and Gram-positive bacterial cell surface possesses net negative electrostatic charge.51 This means that there are repulsive forces between the NB and LA 1028 cell surface. However, once positively charged particles or metal ions are adsorbed on the surface of the NB, the absolute value zeta potential of the NB will decrease, possibly breaking the repulsion equilibrium with the LA 1028 to transfer the adsorbed substances onto the bacterial surface for utilization. After these positively charged substances are detached from the surface of the NB, the repulsive force between the surface of the LA 1028 and NB will repel NB into the solution. The NB works just as a “bus” as described elsewhere.50 Therefore, when the pH is not decreasing to affect the NB to stably present in the solution, the processes of adsorption and release will recirculate and accelerate the strain growth and reproduction. However, the NB stability will be reduced with the accumulation of lactic acid, because the H+ ions could significantly decrease the absolute value of zeta potential that will induce the NB coaggregation and breakage. Thus, the decrease in NB density has limited effects on the stationary phase.
Conclusions
In this study, the effects of four kinds of NBW on the growth of LA1028 were investigated. Results indicate that the gas itself determinates the properties of NBW, which is especially affected by the gas solubility. Besides, the promotion effects of NBW were mainly observed at lag and logarithmic phases of the strain growth, which were manifested by the strain growth, lactic acid production and kinetic analysis. Then, the mechanisms were preliminarily analysed, suggesting that the NBW properties including NB zeta potential, density are probably responsible for the promotion effects on the growth of LA1028. The actual mechanisms should be further investigated. Results from this study indicate that NBW has the potential for promoting the productivity of probiotics in the cultivation, which provides a new prospective for the application of NBW.
Conflicts of interest
There are no conflicts to declare
Acknowledgements
The first author, Mr Zitao Guo would like to thank Ms Di Wang, Ms Xiaojing Yang, Mr Qili Hu, Mr Lingquan Kong and Mr Hanlin Han for their kind help during his preliminary experiments.
References
- N. Anjum, S. Maqsood, T. Masud, A. Ahmad, A. Sohail and A. Momin, Crit. Rev. Food Sci. Nutr., 2014, 54, 1241–1251 CrossRef CAS PubMed.
- B. Sanchez, S. Delgado, A. Blanco-Miguez, A. Lourenco, M. Gueimonde and A. Margolles, Mol. Nutr. Food Res., 2017, 61(1), 1613–4125 CrossRef.
- F. A. M. Klaver, F. Kingma and A. H. Weerkamp, Neth. Milk Dairy J., 1993, 47, 151–164 Search PubMed.
- Probiotics Market by Ingredient Type (Bacteria and Yeast), by Function (Regular Use, Preventive Healthcare, and Therapeutic), by Application (Food and Beverages, Dietary Supplements, and Animal Feed), and by End-user (Human Probiotics and Animal Probiotics): Global Industry Perspective, Comprehensive Analysis and Forecast, 2018–2024, https://www.zionmarketresearch.com/report/probiotics-market, accessed 2019/06/22, 2019.
- C. Lacroix and S. Yidirim, Curr. Opin. Biotechnol., 2007, 18, 176–183 CrossRef CAS.
- Y. J. Oh and D. S. Jung, LWT–Food Sci. Technol., 2015, 63, 437–444 CrossRef CAS.
- S. Plessas, C. Nouska, A. Karapetsas, S. Kazakos, A. Alexopoulos, I. Mantzourani, P. Chondrou, M. Fournomiti, A. Galanis and E. Bezirtzoglou, Food Chem., 2017, 226, 102–108 CrossRef CAS.
- I. Presti, G. D'Orazio, M. Labra, B. La Ferla, V. Mezzasalma, G. Bizzaro, S. Giardina, A. Michelotti, F. Tursi, M. Vassallo and P. Di Gennaro, Appl. Microbiol. Biotechnol., 2015, 99, 5613–5626 CrossRef CAS.
- K. Kailasapathy and J. Chin, Immunol. Cell Biol., 2000, 78, 80–88 CrossRef CAS.
- A. S. Hole, I. Rud, S. Grimmer, S. Sigl, J. Narvhus and S. Sahlstrom, J. Agric. Food Chem., 2012, 60, 6369–6375 CrossRef CAS.
- H. L. Zeng, Y. X. Zheng, Y. Lin, C. C. Huang, S. Lin, B. D. Zheng and Y. Zhang, Food Chem., 2018, 268, 134–142 CrossRef CAS.
- L. Polari, P. Ojansivu, S. Makela, C. Eckerman, B. Holmbom and S. Salminen, J. Agric. Food Chem., 2012, 60, 11037–11043 CrossRef CAS.
- R. Shalini, G. Abinaya, P. Saranya and U. Antony, LWT–Food Sci. Technol., 2017, 83, 68–78 CrossRef CAS.
- S. Y. Li, L. Chen, T. Yang, Q. Wu, Z. J. Lv, B. J. Xie and Z. D. Sun, J. Agric. Food Chem., 2013, 61, 2506–2512 CrossRef CAS.
- E. J. Aguirre-Ezkauriatza, J. M. Aguilar-Yanez, A. Ramirez-Medrano and M. M. Alvarez, Bioresour. Technol., 2010, 101, 2837–2844 CrossRef CAS.
- O. Aydogan, E. Bayraktar and U. Mehmetoglu, Sep. Sci. Technol., 2011, 46, 1164–1171 CrossRef CAS.
- K. Hetenyi, A. Nemeth and B. Sevella, Chem. Eng. Process., 2011, 50, 293–299 CrossRef CAS.
- M. Hujanen, S. Linko, Y. Y. Linko and M. Leisola, Appl. Microbiol. Biotechnol., 2001, 56, 126–130 CrossRef CAS.
- C. Schiraldi, V. Adduci, V. Valli, C. Maresca, M. Giuliano, M. Lamberti, M. Carteni and M. De Rosa, Biotechnol. Bioeng., 2003, 82, 213–222 CrossRef CAS.
- M. Chaplin, Nat. Rev. Mol. Cell Biol., 2006, 7, 861–866 CrossRef CAS.
- S. Passot, S. Cenard, I. Douania, I. C. Trelea and F. Fonseca, Food Chem., 2012, 132, 1699–1705 CrossRef CAS.
- M. P. Rascon, K. Huerta-Vera, L. A. Pascual-Pineda, A. Contreras-Oliva, E. Flores-Andrade, M. Castillo-Morales, E. Bonilla and I. Gonzalez-Morales, LWT–Food Sci. Technol., 2018, 92, 490–496 CrossRef CAS.
- S. Liu, S. Oshita, Y. Makino, Q. Wang, Y. Kawagoe and T. Uchida, ACS Sustainable Chem. Eng., 2016, 4, 1347–1353 CrossRef CAS.
- S. Liu, S. Oshita, S. Kawabata, Y. Makino and T. Yoshimoto, Langmuir, 2016, 32, 11295–11302 CrossRef CAS PubMed.
- S. Liu, S. Oshita, S. Kawabata and T. Dang Quoc, Langmuir, 2017, 33, 12478–12486 CrossRef CAS.
- A. K. A. Ahmed, X. N. Shi, L. K. Hua, L. Manzueta, W. H. Qing, T. Marhaba and W. Zhang, J. Agric. Food Chem., 2018, 66, 5117–5124 CrossRef CAS.
- K. Ebina, K. Shi, M. Hirao, J. Hashimoto, Y. Kawato, S. Kaneshiro, T. Morimoto, K. Koizumi and H. Yoshikawa, PLoS One, 2013, 8(6), e56339 Search PubMed.
- R. Asada, K. Kageyama, H. Tanaka, H. Matsui, M. Kimura, Y. Saitoh and N. Miwa, Oncol. Rep., 2010, 24, 1463–1470 CAS.
- S. Hayakumo, S. Arakawa, M. Takahashi, K. Kondo, Y. Mano and Y. Izumi, Sci. Technol. Adv. Mater., 2014, 15(5), 055003 CrossRef.
- J. Owen, C. McEwan, H. Nesbitt, P. Bovornchutichai, R. Averre, M. Borden, A. P. McHale, J. F. Callan and E. Stride, PLoS One, 2016, 11(12), e0168088 CrossRef.
- F. Kawara, J. Inoue, M. Takenaka, N. Hoshi, A. Masuda, S. Nishiumi, H. Kutsumi, T. Azuma and T. Ohdaira, Digestion, 2014, 90, 10–17 CrossRef CAS.
- D. Wang, X. J. Yang, C. X. Tian, Z. F. Lei, N. Kobayashi, M. Kobayashi, Y. Adachi, K. Shimizu and Z. Y. Zhang, Bioresour. Technol., 2019, 273, 63–69 CrossRef CAS.
- F. Y. Ushikubo, T. Furukawa, R. Nakagawa, M. Enari, Y. Makino, Y. Kawagoe, T. Shiina and S. Oshita, Colloids Surf., A, 2010, 361, 31–37 CrossRef CAS.
- S. Liu, Y. Kawagoe, Y. Makino and S. Oshita, Chem. Eng. Sci., 2013, 93, 250–256 CrossRef CAS.
- L. N. Borshchevskaya, T. L. Gordeeva, A. N. Kalinina and S. P. Sineokii, J. Anal. Chem., 2016, 71, 755–758 CrossRef CAS.
- R. L. Buchanan, R. C. Whiting and W. C. Damert, Food Microbiol., 1997, 14, 313–326 CrossRef.
- M. H. Zwietering, I. Jongenburger, F. M. Rombouts and K. Vantriet, Appl. Environ. Microbiol., 1990, 56, 1875–1881 CAS.
- J. N. Meegoda, S. Aluthgun Hewage and J. H. Batagoda, Environ. Eng. Sci., 2018, 35, 1216–1227 CrossRef CAS.
- T. Uchida, S. Liu, M. Enari, S. Oshita, K. Yamazaki and K. Gohara, Nanomaterials, 2016, 6(2), 2079–4991 CrossRef.
- S. Hamamoto, T. Takemura, K. Suzuki and T. Nishimura, J. Contam. Hydrol., 2018, 208, 61–67 CrossRef CAS.
- A. K. A. Ahmed, C. Z. Sun, L. K. Hua, Z. B. Zhang, Y. H. Zhang, T. Marhaba and W. Zhang, Environ. Eng. Sci., 2018, 35, 720–727 CrossRef.
- S. Calgaroto, K. Q. Wilberg and J. Rubio, Miner. Eng., 2014, 60, 33–40 CrossRef CAS.
- E. Ruckenstein, Colloids Surf., A, 2013, 423, 112–114 CrossRef CAS.
- C. Oliveira and J. Rubio, Int. J. Miner. Process., 2011, 98, 118–123 CrossRef CAS.
- N. Nirmalkar, A. W. Pacek and M. Barigou, Langmuir, 2018, 34, 10964–10973 CrossRef CAS.
- M. D. Rolfe, C. J. Rice, S. Lucchini, C. Pin, A. Thompson, A. D. S. Cameron, M. Alston, M. F. Stringer, R. P. Betts, J. Baranyi, M. W. Peck and J. C. D. Hinton, J. Bacteriol., 2012, 194, 686–701 CrossRef CAS.
- S. Bhattacharjee, J. Controlled Release, 2016, 235, 337–351 CrossRef CAS.
- Y. M. Kou Sugano and S. Inazato, Japanese Journal of Multiphase Flow, 2017, 31, 299–306 CrossRef.
- H. X. Liu, R. S. Zhang, X. J. Yao, M. C. Liu, Z. D. Hu and B. T. Fan, J. Chem. Inf. Comput. Sci., 2004, 44, 161–167 CrossRef CAS.
- G. Z. Kyzas, G. Bomis, R. I. Kosheleva, E. K. Efthimiadou, E. P. Favvasa, M. Kostoglou and A. C. Mitropoulos, Chem. Eng. J., 2019, 356, 91–97 CrossRef CAS.
- W. W. Wilson, M. M. Wade, S. C. Holman and F. R. Champlin, J. Microbiol. Methods, 2001, 43, 153–164 CrossRef CAS.
Footnote |
† Electronic supplementary information (ESI) available. See DOI: 10.1039/c9ra05868k |
|
This journal is © The Royal Society of Chemistry 2019 |
Click here to see how this site uses Cookies. View our privacy policy here.