DOI:
10.1039/C9RA05198H
(Paper)
RSC Adv., 2019,
9, 27904-27910
Nepenthes-inspired multifunctional nanoblades with mechanical bactericidal, self-cleaning and insect anti-adhesive characteristics†
Received
8th July 2019
, Accepted 24th August 2019
First published on 4th September 2019
Abstract
In order to reduce the widespread threat of bacterial pathogen diseases, mechanical bactericidal surfaces have been widely reported. However, few of these nanostructured surfaces were investigated from a sustainable perspective. In this study, we have prepared, inspired by the slippery zone of Nepenthes, a multifunctional nanostructured surface with mechanical bactericidal, self-cleaning and insect anti-adhesive characteristics. First, a nanoblade-like surface made of Zn–Al layered double hydroxides was prepared for achieving faster bactericidal rate and wider bactericidal spectrum (2.10 × 104 CFU cm−2 min−1 against Escherichia coli and 1.78 × 103 CFU cm−2 min−1 against Staphylococcus aureus). Then the self-cleaning and insect anti-adhesive properties were tested on the fluorosilane-modified nanoblades, leaving little cell debris remaining on the surface even after 4 continuous bactericidal experiments, and showing a slippery surface for ants to slide down in 3 s. This study not only discovers a new nature-inspired mechanical bactericidal nanotopography, but also provides a facile approach to incorporate multiple functions into the nanostructured surface for practical antibacterial applications.
Introduction
Microbial contamination is one of the most serious problems in medical and commercial applications.1 Traditional antimicrobial coatings using natural antibiotics2,3 and artificial fungicides4,5 are meant to prevent the adhesion and proliferation of bacteria. However, they fail to prevent secondary contamination6,7 nor antimicrobial resistance (AMR),8 which promotes the search for new bactericidal strategies. Recently, a series of novel nanostructured surfaces have been reported based on a mechano-responsive bactericidal mechanism, inspired by the nanotopographies found in Nature.1,9–13
Ivanova et al. first discovered the structure of bactericidal nanorod array from the surface of cicada wing,14,15 which ruptures the cell membrane via direct contact. This mechanically-based bactericidal action is both non-toxic and does not induce drug resistance, and so attracted much attention.16–20 Previously reports in this field mainly focus on the discovery of new natural micro–nano bactericidal structures1,10–13 the correlation between the morphology of nanorod array and bactericidal performance,13,21,22 and the study of the physical bactericidal mechanism.23,24 However, the impact of the cell debris left on the mechanical bactericidal surface has yet been investigated systematically, from the perspective of a sustainable and effective antibacterial surfaces.20 In fact, cell debris will accumulate continuously on the surface along with the progress of the sterilization process, which affects the effective contact between bacteria and bactericidal nanostructures and reduces the bactericidal performance.21 In addition, since cellular debris can also contaminate surfaces and serves as a source of nutrients for living bacteria cells,25 it is essential to quickly and effectively remove the cell debris from the protected surfaces. Among the few studies involving the removal of cellular debris, none of them applies the mechano-responsive bactericidal mechanism, but they either rely on the deformation of smart materials or photocatalysis to remove the cellular debris.26–28 Besides, these methods need extra help such as light sources for photocatalysis28 or specific stimuli for smart materials26,27,29 that are not that convenient in practical applications, which leaves the question mark for whether there is a simple approach to remove the cell debris from the bactericidal nanostructured surfaces.
Fortunately, nature provides the answer. Nepenthes pitcher plant has been well studied for its slippery surface.30–33 The existing researches on surface lubrication of Nepenthes pitcher mainly include two aspects. (1) The microstructure with radial ridges on the pitcher rim (peristome) can realize the self-transport of liquid and form a film on the surface.34 Thus, insect “aquaplaning effect” is achieved.30 Ultra-slippery coatings with a similar principle of low surface functionalization of lubricants have been artificially imitated.35 On this basis, Joanna Aizenberg et al. report that using lubricants effectively prevents bacteria from adhering and growing on substrate.36,37 Lynn's group further selected lubricants with bactericidal activity that confer bactericidal properties on surfaces.38 The surface nanostructure plays the dual functions of maintaining and releasing the lubricant at the same time, which helps to realize the long-term sterilization of the surface. However, the bactericidal properties of the surface are still achieved by chemical fungicides, which can still cause problems such as drug resistance. (2) The lubrication of insect feet against the epicuticular wax crystals on the slippery zone.39 However, these studies did not involve the interaction between the inner surface of pitcher plant and bacteria. In this study, we found the slippery zone possessing a weak but still effective mechanical bactericidal activity, which is yet reported in literature. Inspired by this idea, we report a multifunctional nanostructured surface with mechanical bactericidal, self-cleaning and insect anti-adhesive characteristics, expecting to promote the development of mechanical bactericidal surfaces for practical and sustainable applications fighting against threats from pathogen and vector-borne diseases.
Experimental section
Materials
Aluminum alloy sheet (AAS, 5A06), pure ethanol and sodium carbonate (Na2CO3) were purchased from Chang Zheng (Chengdu). Zinc nitrate hexahydrate (Zn(NO3)2·6H2O) and hexamethylenetetramine (HMTA, (CH2)6N4) were purchased from Aladdin (Shanghai). All the chemicals used in this work were analytical grade. Deionized water was used as the hydrothermal growth solution.
Preparation of pitcher plant sheets
The pitcher plant Nepenthes alata specimens were purchased from San Sheng, a local flower market in Chengdu. The slippery zone sheets (1 cm × 1 cm) were carefully cut out from Nepenthes alata pitchers. To fabricate collapsed natural nanoblade (CNNB) surfaces, NNB surfaces were covered with a cover glass and adding pressure by a counterweight (100 g) for 10 min.
Hydrothermal growth of ANBs
The chemical bath deposition (CBD) was used to construct Zn–Al layered double hydroxides NBs on AAS. A piece of AAS substrate (1 cm × 1 cm) was first immersed into an 80 mL hydrothermal growth solution which contained Na2CO3 (1 mL, 1 mM), and equimolar (40 mL, 10 mM) Zn(NO3)2·H2O and HMTA in aqueous solution at 50, 70 and 90 °C for 30 min. Then the substrate was cleaned with ethanol and deionized water several times to remove any organic residue. To fabricate compacted artificial nanoblade (CANB) sheets, ANBs sheet were also covered with a cover glass and adding pressure by a counterweight (100 g) and compact for 10 min.
Characterization
To characterize the ANBs surface topographies, field emission scanning electron microscopy (FE-SEM, JSM-7001F, JEOL) was used at a voltage of 20 kV. A nanoblade scraped from the substrate was characterized by transmission electron microscopy (TEM, JEM-2100, JEOL). X-ray diffraction patterns were recorded by an X-ray diffractometer (XRD, Philips X' Pert PRO), with Cu Kα radiation (λ = 1.5418 Å).
Bactericidal properties of NNBs and ANBs
The bactericidal properties of the wax NNBs and Zn–Al layered double hydroxides ANBs against Escherichia coli (E. coli) and Staphylococcus aureus (S. aureus) cells were evaluated using the sticking membrane method referring to the JIS Z 2801 (Japanese Industrial Standard).10,40 Using this method, the selected bacterial suspension (E. coli strain [ATCC 25922] and S. aureus strain [ATCC 6538], purchased from Guang Dong Detection Center of Microbiology, China) was activated on an agar culture medium (AOBOX Co., Ltd. Beijing) overnight at 37 °C and proliferated in a nutrient broth medium at 37 °C for 3 h. The bacterial cell suspension was then diluted by broth medium to proper concentration (NNBs: E. coli, 2.1 × 105 CFU mL−1 to 2.9 × 105 CFU mL−1; ANBs: E. coli, 2.2 × 106 CFU mL−1 to 2.9 × 106 CFU mL−1 and S. aureus, 1.3 × 105 CFU mL−1 to 2.6 × 105 CFU mL−1) to afford a standardized suspension. All samples (slippery zone sheet, aluminum alloy sheet, ANBs sheets, ACNBs sheets and PE membrane) were cut into 1 cm × 1 cm and immersed into 75% ethanol for 1 min and dried in an ultra-clean cabinet prior to use. 100 μL of standardized bacterial cell suspension was added onto each sample surface and incubated at 37 °C in dark for 10 min, 60 min, and 120 min, respectively. All the height of the drops is accuracy controlled to avoid the interference of initial droplet rate. Each sample was thoroughly washed with 1 mL sterilized physiological saline. The bacterial cell suspensions were subsequently diluted in sterile physiological saline for suited concentration. Cell counts were obtained by spreading 100 μL of bacterial cell suspension onto agar plates. CFU on each agar plate were counted after incubation at 37 °C for 18 h, and the number of CFU per cm2 was calculated. Each test is carried out in dark conditions and repeated five times.
Interactions between bacterial cells and NBs
The E. coli strain was activated on an agar culture medium at 37 °C overnight, followed by proliferation in 30 mL of nutrient broth medium at 37 °C for 3 h. 100 μL of bacterial suspension was then added onto each surface. After incubation for 10 min, samples were fixed in 2.5% glutaraldehyde for 12 h at 4 °C, followed by ethanol gradient dehydration. The samples were soaked for 15 min in each step. After drying overnight at room temperature, bacterial cell topographies were observed by FE-SEM.
In the self-cleaning experiment, each sample was washed by simulated rain (20 μL s−1, 10 s) after 4 times bactericidal experiments. Then the SEM observation and the contact angle measurements were carried out.
Bacterial cell integrity on NBs
Confocal laser scanning microscope (CLSM 510 Meta laser scanning confocal microscope, Carl Zeiss) was used to observe bacterial cells on the NB surfaces. E. coli cells were stained with the fluorescent nucleic acid dye kit containing SYTO-9 and PI (LIVE/DEAD BacLight Bacterial Viability Kit, Invitrogen). Prior to microscopic observation, 1 mL of bacterial cell suspension was stained with 1.5 μL of a PI and SYTO-9 mixture (1
:
1, v/v), followed by incubation for 15 min. Then the testing samples were immersed into stained bacterial cell suspension and flat on the aseptic bench, followed by covering with a sterilized cover slip. After incubating for another 1 min, the samples were observed under CLSM. All the steps were proceeded in dark at room temperature.
Preparation of grafted-ANBs
The HD-ANBs sheets were immersed into (heptadecafluoro-1,1,2,2-tetrahydrodecyl) trimethoxy silane isopropanol solution (1.5%, v/v) for 10 min. Then the samples were transferred to 60 °C oven for 5 min. The grafted-ANBs were prepared.
Insect anti-adhesive experiments
To evaluate the insect anti-adhesive performance of NNB and ANB surface, insect resistant crawling experiments were adopted. Pheidole jucunda ants were utilized. For NNB surface, the slippery zone of Nepenthes alata were cut into proper size and wrapped around two test tubes. Five ants were placed into the bottom of the vertical device and watch their crawling behaviour within 3 min. The number of the ants reaching the upper of the device was recorded. For ANB and grafted-ANB surfaces, the ants were placed on to tested sheets which were pre-placed at an inclination of 45°. Then the inclination of the sheets was gradually adjusted to 60°, the climbing performance of ants on the surface was observed in this process.
Results and discussion
The mechanical bactericidal performance of natural nanoblades
As a typical tropical insectivorous plant, Nepenthes pitcher plant grows in high temperature and humidity (annual average temperature: 25 °C, RH > 75%). Because of its insect-catching properties, the traps often contain dead insect and digestive juices dissolved with small molecular nutrients. Therefore, the internal environment is very suitable for bacterial growth. These potential pathogen contamination factors all require that the pitchers have certain antibacterial properties. So first, we focus on the insect aquaplaning surface of the peristome,30,41 but the surface doesn't exhibit bactericidal performance (Fig. S1†). However, the slippery zone below the peristome inside pitches (Fig. 1a) is also slippery for successful trapping and shows bactericidal performance against E. coli (Fig. 1b). The CLSM also indicate the integrity of E. coli cells (shows red fluorescence) were destroyed by Nepenthes slippery zone (NSZ, Fig. 1b). As shown in Fig. 1c, NSZ surface is covered by numerous blade-like waxy crystals,42 and these natural nanoblades (NNBs, average thickness: 78.4 nm, density: 4.1 × 108 sheet per cm2) seems killing E. coli cells by tearing their cell membrane (Fig. S3c†). Furthermore, a compacted natural nanoblades (CNNBs) were also adopted in bactericidal experiment as control (Fig. S3†). The E. coli cells are able to maintain their natural integrity on a flat CNNBs surface (Fig. S3b†), which demonstrates that the nanostructures play an important role in the killing of bacteria, and the chemical composition of the NSZ has little effect on the bacteria. Besides, the production of reactive oxygen species (ROS) is also independent with bactericidal performance. Both of the H2O2 and ·O2− produced by NNBs and CNNBs were similar respectively under experimental conditions (Fig. S2a and b†). Thus, the bactericidal activity of NNB surfaces is merely based on the mechanical bactericidal mechanism.
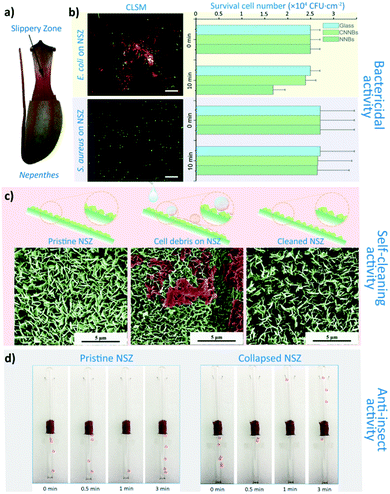 |
| Fig. 1 The bactericidal activity, self-cleaning property and insect anti-adhesion of Nepenthes slippery zone. (a) Pitcher of Nepenthes; (b) Confocal Laser Scanning Microscope (CLSM) images and bactericidal performance statistical results of E. coli and S. aureus on NSZ surface. The data are expressed as mean ± S.D. of three replicates; (c) SEM images of pristine NSZ, E. coli debris on NSZ and contaminated NSZ surfaces after washing; (d) Pheidole jucunda ants climbing performance on NSZ and CNSZ surfaces within 3 min. | |
The self-cleaning and insect anti-adhesive performance of natural nanoblades
The NSZ surface also shows a good self-cleaning property against cell debris. As shown in Fig. 1c, the bacterial cell debris on the NSZ surface could be completely removed by a simple washing, mimicking the rain dropping in nature (20 μL s−1, 10 s). In this case, the NNBs can be re-exposed to ensure the bactericidal performance is sustainable. The insect anti-adhesive experiment (Fig. 1d) could initially show that the NSZ surface were slippery to ant feet, for the ants were unable to crawl to the upper region of the apparatus within 3 minutes. This is due to the detachable property of the epicuticular wax crystals under the weight load of an insect foot.42 As mentioned above, the NSZ surface has the multifunctional properties of bacteria killing, self-cleaning and insect anti-adhesion.
Although NSZ has the desired multifunctional properties, there are three problems with the NNBs structure. First, the cell morphology as shown in Fig. S3f and f′† indicated that S. aureus cells could maintain their integrity on NNBs surface, which suggests NNBs have no bactericidal effect on S. aureus. The bactericidal experiments show a narrow bactericidal spectrum (Fig. 1b), this may due to the higher tip-width of the NNBs. Second, after the self-cleaning experiment, the density of NNBs decreased, which indicates that the wax crystals are brittle and low mechanical properties (Fig. 1c). Third, there are studies showing that both of the NNBs and lunate cells of NSZ play major role in insects attachment.39,43 In other words, due to the anisotropic structure and distribution of the lunate cells, the insect attachment efficiency is also greatly affected by the orientation of the Nepenthes alata pitchers. And the brittle wax crystals also impact the sustainable insect anti-adhesive function. These problems heavily affect the practical application for insect control and bactericidal purposes.
Preparation and characterization of artificial nanoblades
To address the weak mechanical strength of NNBs and to achieve superior mechanical bactericidal performance for higher bactericidal activity and wider bactericidal spectrum, we adopted a simple hydrothermal method to fabricate the artificial nanoblades (ANBs). We designed the ANBs so as to inherit and advance the merits of NSZ from three aspects: bactericidal activity, self-cleaning and insect anti-adhesion to prevent the carried pathogens, the cell debris and the insect vectors. By optimizing the hydrothermal growth conditions and growth time, a series of ANBs with different density were prepared, namely low density ANBs, medium density ANBs and high density ANBs (LD-ANBs, MD-ANBs and HD-ANBs, Fig. S4a–d†). The ANBs topography statistical results were shown in Fig. S4e and f,† suggests a successful imitation of natural nanoblade structure. HD-ANBs had minimum average blade width (29.3 ± 5.7 nm) and highest density (4.4 ± 0.2 × 109 slice per cm2). The degradation rate of HMTA increases as reaction temperature increases. Therefore, under high temperature, a mass of precursor was generated and participate reaction in initial reaction system. Thereby the growth rate of ANBs was higher and resulted in thicker ANBs. In addition, many ANBs crystals were combined during growth process, caused density decrease (Fig. S4b†). With the increase of growth temperature, the height of ANBs also increased Fig. S4b.† Thus, thicker and low density ANBs were obtained under higher growth temperature. According to the density, the ANBs were intitule as high density ANBs (HD-ANBs, Fig. 2b, Fig. S4d†), medium density ANBs (MD-ANBs, Fig. S4c†) and low density ANBs (LD-ANBs, Fig. S4b†). Structure of ANBs was characterized by X-ray diffraction (XRD). The patterns of the XRD show diffraction peaks at 11.6° and 23.4° to (003) and (006) planes respectively, clearly showing the formation of the hcp LDH phase.44 The peak at 23.4° is probably due to the presence of CO3-LDH, which were indexed to a typical Zn–Al layered double hydroxide (Zn6Al2(OH)16CO3·4H2O, JCPDS card, no. 038-0486) structure (Fig. S5a†).45 The structure of LDHs was further characterized by TEM. After scraped from the substrate and ultrasonicated in ethanol, the sheet-like nanoblades were also observed (Fig. S5b†). EDS spectrum (Fig. S5c†) showed that the nanoblades were mainly composed of zinc, aluminium, and oxygen elements, indicating Al from the AAS participated in the growth of nanoblades under the hydrothermal conditions.
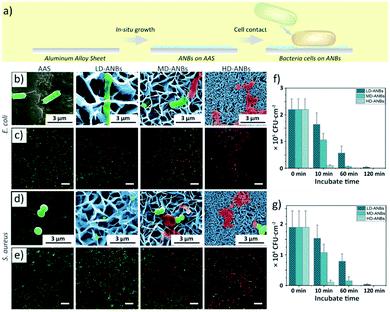 |
| Fig. 2 The mechanical bactericidal activity of ANBs. (a) Schematic diagram of ANBs construction and in contact with bacteria; (b–e) interaction of E. coli and S. aureus cells with ANBs observed by SEM and CLSM, contact time: 10 min. (f and g) The bactericidal experiment statistical results of E. coli and S. aureus on ANBs surface. The data are expressed as mean ± S.D. of three replicates. Scale bar 25 μm. | |
The bactericidal performance of artificial nanoblades
The interaction of ANBs with bacteria cells were shown in Fig. 2b–e. There is a significant correlation between the extent of cell destruction and the tip-width of the ANBs. The E. coli and S. aureus cell keeps their integrity shape and shows green fluorescence on LD-ANBs and AAS, which indicates the surface have no lethal effect against these two bacteria. However, cell debris was found on the thinner ANB surface, where the integrity of cell debris was negatively correlated with tip-width. Bactericidal experiments were also carried out to evaluate the ANBs bactericidal properties. A trend between the density and thickness of the ANBs and bactericidal results was observed (Fig. 2f, g and S6†). With the decrease of blade thickness and the increase of array density, the bactericidal performance of the surface increases. The tip-width of the nano-blade determines its efficiency in puncturing bacterial cells, while the NBs density determines the number of damage sites of a single bacterial cell. The HD-ANBs with thinner tip-width shows a fastest bactericidal rate (2.10 ± 0.36 × 104 CFU cm−2 min−1 against E. coli and 1.78 ± 0.47 × 103 CFU cm−2 min−1 against S. aureus) and a wider bactericidal spectrum (against both of two bacteria) within 10 min contact. Such a simple method could imitate and surpass the bactericidal performance of NNBs. To the best of our knowledge, the ROS and Zn2+ from ANBs may also contribute to the bactericidal properties. However, in the yield statistics of ·OH, ·O2− and Zn2+, no obvious differences among three ANBs samples could be observed (Fig. S7 and Table S1†). That is, the bactericidal activity of the ANBs is also independent of the chemical product or composition. The results of bactericidal research of ZnAl LDHs showed that the compound did not have any bactericidal properties.46 Besides, related reports on the waterborne polyurethane surface filled with 1 wt% ZnAl LDHs of sterilization performance can kill 2.64 × 104 bacteria within 24 h.47 The bactericidal rate is much lower than NBs surface, therefore, it can be inferred that even though ZnAl LDHs has bactericidal property, it does not have obvious effect during the experimental period (10 min).
The self-cleaning and insect anti-adhesive performance of the artificial nanoblades
Since the physical bactericidal properties are based on the direct contact between bacteria and cell debris will form accordingly on ANBs, a continuous bactericidal experiment was carried out in order to evaluate the impact of cell debris on ANBs. As shown in Fig. 3b and c, cell debris was accumulated in the gaps of the ANBs after 4 times experiment, due to the higher surface energy. The effectiveness of surface bactericidal activity is also affected in a negative way (Fig. 3d and e), this may be attributed to the accumulation of bacterial debris on the surface which affects the morphology of nanoblade (Fig. 3b and c). Due to the presence of hydrophilic compounds such as peptidoglycan and protein on the cell wall, same results were obtained in the water contact angle experiment. The surface of ANBs contaminated by the bacterial debris shows hydrophilic behaviour, with a lower contact angle of about 10–20° (Fig. 3b and c). In order to mimic the low surface energy of NSZ, the HD-ANBs was grafted with (heptadecafluoro-1,1,2,2-tetrahydrodecyl) trimethoxy silane. The grafted HD-ANB surface has little cell debris remained on the surface after 4 times bactericidal experiments (Fig. 3b and c), which indicates that the bacterial debris is difficult to adhere on the grafted HD-ANBs surface, and demonstrates a good self-cleaning property. Furthermore, because the tips of grafted-ANBs were kept exposed, the surface remains super hydrophobic (Fig. 3b and c) and the bactericidal activity is consistently sustainable (Fig. 3d and e). Thus, the simulation of self-cleaning performance is realized and the sustainable application of mechanical bactericidal performance is guaranteed.
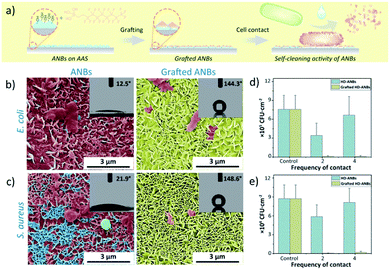 |
| Fig. 3 The self-cleaning and sustainable bactericidal performance of grafted HD-ANBs. (a) Schematic diagram of ANBs grafting and self-cleaning of surface bacteria debris; (b and c) SEM images of HD-ANBs and grafted HD-ANBs contact with E. coli cells and S. aureus for 4 times continuously. (d and e) The bactericidal performance statistical results of E. coli and S. aureus on HD-ANBs and grafted HD-ANBs surface for 2 and 4 times. Contact time: 10 min. The data are expressed as mean ± S.D. of three replicates. | |
In addition to the self-cleaning properties, surface fluorination can significantly increase the anti-climbing performance of the surface. The insect anti-adhesive experiment was also carried out and shown in Fig. 4. The adhesive attachment of the ants, beetles and locusts (adhesive pads) achieve wet adhesion to surfaces through terminal secretion.48–52 According to the study of insect adhesion theory, insects show higher adhesion on smooth surfaces and lower adhesion on micro-rough surfaces than on nano-rough ones.53–55 So that the Pheidole jucunda ants can crawl freely on the smooth AAS surface (Fig. 4a). On HD-ANBs surface (tip-width 30 nm), ants can stay on the same position for 20 seconds, indicating that the surface can prevent insects from crawling to some extent (Fig. 4b). Therefore, we speculate that, the blade structure on the surface of ANBs could not only effectively reduce the contact area between the adhesive pad and the substrate,41,52,56 but also the pore structure between the blades with high surface energy could quickly absorb the secretions of the adhesive pad, affecting the formation of effective adhesion between the ants feet and the surface.57 For grafted-ANBS surfaces, due to the low surface energy of the blade surface after fluorination, the adhesion pad exudates have difficulty in wet adhering the surface, which reduces the force of insect feet on the surface. In the meantime, because the ANBs surface does not have any micron level lunate cells, the anti-climbing performance of the surface shows isotropy. Therefore, as shown in Fig. 4c, it is difficult for ants to stay on grafted HD-ANBs surfaces, which slide off the surface in just three seconds. This indicates that the HD-ANBs is a premium insect anti-adhesive surface.
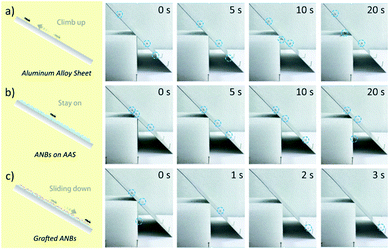 |
| Fig. 4 The insect anti-adhesive performance of grafted ANBs. Pheidole jucunda ants crawling on (a) AAS, (b) HD-ANBs and (c) Grafted HD-ANBs. | |
Conclusions
In summary, in order to prevent the accumulated bacterial debris affect to the mechano-bactericidal performance, we learn from nature. The mechanical bactericidal activity and self-cleaning performance of Nepenthes slippery zone was first found. Inspired by the pitcher plant, the bactericidal nanoblades structure was mimicked by using a simple hydrothermal method. Furthermore, compared with the NSZ surfaces, thinner, denser and higher mechanical properties nanoblades were prepared, with higher bactericidal rate and wider sterilization spectrum performance. After a simple fluorination treatment, the ANBs surface successfully mimic the self-cleaning and insect anti-adhesive performance of NSZ surface. Such multifunctional surface has a promising potential to be applied in sustainable applications avoiding the abuse of sterilizing agent, thereby not only inhibiting the pernicious environment pollution but also preventing high hazard vector-borne diseases.
Conflicts of interest
There are no conflicts to declare.
Acknowledgements
This work was financially supported by National Natural Science Foundation of China (No. 51772251), the Science and Technology Planning Project of Sichuan Province (No. 2017RZ0032 and No. 2018GZ0462), and the Fundamental Research Funds for the Central Universities (No. 2019XJ02).
Notes and references
- Y. Yuan and Y. G. Zhang, Nanomed Nanotechnol, 2017, 13, 2199–2207 CrossRef CAS PubMed.
- K. Glinel, A. M. Jonas, T. Jouenne, J. Leprince, L. Galas and W. T. Huck, Bioconjugate Chem., 2009, 20, 71–77 CrossRef CAS PubMed.
- W. X. Lei, X. C. Chen, M. Hu, H. Chang, H. Xu, K. F. Ren and J. Ji, J. Mater. Chem. B, 2016, 4, 6358–6365 RSC.
- W. He, Y. Zhang, J. Li, Y. Gao, F. Luo, H. Tan, K. Wang and Q. Fu, Sci. Rep., 2016, 6, 32140 CrossRef CAS PubMed.
- B. Wang, Z. Ye, Y. Tang, Y. Han, Q. Lin, H. Liu, H. Chen and K. Nan, Int. J. Nanomed., 2017, 12, 111–125 CrossRef CAS PubMed.
- A. Tello, B. Austin and T. C. Telfer, Environ. Health Perspect., 2012, 120, 1100–1106 CrossRef PubMed.
- L. Norgrove, Eur. J. Soil Biol., 2007, 43, S303–S310 CrossRef CAS.
- A. P. Magiorakos, A. Srinivasan, R. B. Carey, Y. Carmeli, M. E. Falagas, C. G. Giske, S. Harbarth, J. F. Hindler, G. Kahlmeter, B. Olsson-Liljequist, D. L. Paterson, L. B. Rice, J. Stelling, M. J. Struelens, A. Vatopoulos, J. T. Weber and D. L. Monnet, Clin. Microbiol. Infect., 2012, 18, 268–281 CrossRef CAS PubMed.
- V. T. H. Pham, V. K. Truong, D. E. Mainwaring, Y. Guo, V. A. Baulin, M. Al Kobaisi, G. Gervinskas, S. Juodkazis, W. R. Zeng, P. P. Doran, R. J. Crawford and E. P. Ivanova, J. Mater. Chem. B, 2014, 2, 2819–2826 RSC.
- G. Yi, Y. Yuan, X. Li and Y. Zhang, Small, 2018, 14, e1703159 CrossRef PubMed.
- E. P. Ivanova, J. Hasan, H. K. Webb, G. Gervinskas, S. Juodkazis, V. K. Truong, A. H. Wu, R. N. Lamb, V. A. Baulin, G. S. Watson, J. A. Watson, D. E. Mainwaring and R. J. Crawford, Nat. Commun., 2013, 4, 2838 CrossRef PubMed.
- L. E. Fisher, Y. Yang, M. F. Yuen, W. Zhang, A. H. Nobbs and B. Su, Biointerphases, 2016, 11, 011014 CrossRef PubMed.
- A. Elbourne, V. E. Coyle, V. K. Truong, Y. M. Sabri, A. E. Kandjani, S. K. Bhargava, E. P. Ivanova and R. J. Crawford, Nanoscale Adv., 2019, 1, 203–212 RSC.
- J. Hasan, H. K. Webb, V. K. Truong, S. Pogodin, V. A. Baulin, G. S. Watson, J. A. Watson, R. J. Crawford and E. P. Ivanova, Appl. Microbiol. Biotechnol., 2013, 97, 9257–9262 CrossRef CAS PubMed.
- E. P. Ivanova, J. Hasan, H. K. Webb, V. K. Truong, G. S. Watson, J. A. Watson, V. A. Baulin, S. Pogodin, J. Y. Wang, M. J. Tobin, C. Lobbe and R. J. Crawford, Small, 2012, 8, 2489–2494 CrossRef CAS PubMed.
- A. Elbourne, R. J. Crawford and E. P. Ivanova, J. Colloid Interface Sci., 2017, 508, 603–616 CrossRef CAS PubMed.
- J. Hasan, R. J. Crawford and E. P. Ivanova, Trends Biotechnol., 2013, 31, 295–304 CrossRef CAS PubMed.
- J. Meng, P. Zhang and S. Wang, Chem.–Asian J., 2014, 9, 2004–2016 CrossRef CAS PubMed.
- A. Tripathy, P. Sen, B. Su and W. H. Briscoe, Adv. Colloid Interface Sci., 2017, 248, 85–104 CrossRef CAS PubMed.
- N. Lin, P. Berton, C. Moraes, R. D. Rogers and N. Tufenkji, Adv. Colloid Interface Sci., 2018, 252, 55–68 CrossRef CAS PubMed.
- K. Nowlin, A. Boseman, A. Covell and D. LaJeunesse, J. R. Soc. Interface, 2015, 12, 20140999 CrossRef PubMed.
- M. N. Dickson, E. I. Liang, L. A. Rodriguez, N. Vollereaux and A. F. Yee, Biointerphases, 2015, 10, 021010 CrossRef PubMed.
- C. D. Bandara, S. Singh, I. O. Afara, A. Wolff, T. Tesfamichael, K. Ostrikov and A. Oloyede, ACS Appl. Mater. Interfaces, 2017, 9, 6746–6760 CrossRef CAS PubMed.
- S. Pogodin, J. Hasan, V. A. Baulin, H. K. Webb, V. K. Truong, T. H. Phong Nguyen, V. Boshkovikj, C. J. Fluke, G. S. Watson, J. A. Watson, R. J. Crawford and E. P. Ivanova, Biophys. J., 2013, 104, 835–840 CrossRef CAS PubMed.
- G. Amy, Desalination, 2008, 231, 44–51 CrossRef CAS.
- Z. Cao, L. Mi, J. Mendiola, J. R. Ella-Menye, L. Zhang, H. Xue and S. Jiang, Angew. Chem., Int. Ed. Engl., 2012, 51, 2602–2605 CrossRef CAS PubMed.
- L. Mi, M. T. Bernards, G. Cheng, Q. Yu and S. Jiang, Biomaterials, 2010, 31, 2919–2925 CrossRef CAS PubMed.
- J. Meng, P. Zhang, F. Zhang, H. Liu, J. Fan, X. Liu, G. Yang, L. Jiang and S. Wang, ACS Nano, 2015, 9, 9284–9291 CrossRef CAS PubMed.
- B. Fang, Y. Jiang, V. M. Rotello, K. Nüsslein and M. M. Santore, ACS Nano, 2014, 8, 1180–1190 CrossRef CAS PubMed.
- H. F. Bohn and W. Federle, Proc. Natl. Acad. Sci. U. S. A., 2004, 101, 14138–14143 CrossRef CAS PubMed.
- L. Gaume, S. Gorb and N. Rowe, New Phytol., 2002, 156, 479–489 CrossRef.
- L. Wang, S. Dong and Q. Zhou, J. Bionic Eng., 2016, 13, 373–387 CrossRef.
- D. S. Luthe, F. Buch, W. E. Kaman, F. J. Bikker, A. Yilamujiang and A. Mithöfer, PLoS ONE, 2015, 10, e0118853 CrossRef PubMed.
- H. Chen, P. Zhang, L. Zhang, H. Liu, Y. Jiang, D. Zhang, Z. Han and L. Jiang, Nature, 2016, 532, 85–89 CrossRef CAS PubMed.
- T. S. Wong, S. H. Kang, S. K. Tang, E. J. Smythe, B. D. Hatton, A. Grinthal and J. Aizenberg, Nature, 2011, 477, 443–447 CrossRef CAS PubMed.
- N. MacCallum, C. Howell, P. Kim, D. Sun, R. Friedlander, J. Ranisau, O. Ahanotu, J. J. Lin, A. Vena, B. Hatton, T.-S. Wong and J. Aizenberg, ACS Biomater. Sci. Eng., 2014, 1, 43–51 CrossRef.
- J. Chen, C. Howell, C. A. Haller, M. S. Patel, P. Ayala, K. A. Moravec, E. Dai, L. Liu, I. Sotiri, M. Aizenberg, J. Aizenberg and E. L. Chaikof, Biomaterials, 2017, 113, 80–92 CrossRef CAS PubMed.
- M. J. Kratochvil, M. A. Welsh, U. Manna, B. J. Ortiz, H. E. Blackwell and D. M. Lynn, ACS Infect. Dis., 2016, 2, 509–517 CrossRef CAS PubMed.
- I. Scholz, M. Buckins, L. Dolge, T. Erlinghagen, A. Weth, F. Hischen, J. Mayer, S. Hoffmann, M. Riederer, M. Riedel and W. Baumgartner, J. Exp. Biol., 2010, 213, 1115–1125 CrossRef CAS PubMed.
- Japanese Industrial Standard: JIS Z 2801: 2000, http://www.techstreet.com/standards/jis-z-2801-2000?product_id=1233140, accessed: August 2001.
- H. Peisker and S. N. Gorb, J. Exp. Biol., 2010, 213, 3457–3462 CrossRef PubMed.
- L. Gaume, P. Perret, E. Gorb, S. Gorb, J. J. Labat and N. Rowe, Arthropod Struct. Dev., 2004, 33, 103–111 CrossRef CAS PubMed.
- P. F. Zhang, H. W. Chen and D. Y. Zhang, Journal of Bionic Engineering, 2015, 12, 79–87 CrossRef.
- X. Duan and D. G. Evans, in Layered Double Hydroxides, Struct. Bond, Springer, 2006, vol. 119, pp. 193–223 Search PubMed.
- J. Liu, X. Huang, Y. Li, K. M. Sulieman, X. He and F. Sun, J. Phys. Chem. B, 2006, 110, 21865–21872 CrossRef CAS PubMed.
- M. A. Djebbi, A. Elabed, Z. Bouaziz, M. Sadiki, S. Elabed, P. Namour, N. Jaffrezic-Renault and A. B. H. Amara, Int. J. Pharm., 2016, 515, 422–430 CrossRef CAS PubMed.
- W.-D. Zhang, Y.-M. Zheng, Y.-S. Xu, Y.-X. Yu, Q.-S. Shi, L. Liu, H. Peng and Y. Ouyang, J. Nanosci. Nanotechnol., 2013, 13, 409–416 CrossRef CAS PubMed.
- S. Ishii, Appl. Entomol. Zool., 1987, 22, 222–228 CrossRef.
- T. Eisner and D. J. Aneshansley, Proc. Natl. Acad. Sci. U. S. A., 2000, 97, 6568–6573 CrossRef CAS PubMed.
- W. Federle, E. L. Brainerd, T. A. McMahon and B. Holldobler, Proc. Natl. Acad. Sci. U. S. A., 2001, 98, 6215–6220 CrossRef CAS PubMed.
- W. Votsch, G. Nicholson, R. Muller, Y. D. Stierhof, S. Gorb and U. Schwarz, Insect Biochem. Mol. Biol., 2002, 32, 1605–1613 CrossRef CAS PubMed.
- M. W. England, T. Sato, M. Yagihashi, A. Hozumi, S. N. Gorb and E. V. Gorb, Beilstein J. Nanotechnol., 2016, 7, 1471–1479 CrossRef CAS PubMed.
- A. Peressadko and S. N. Gorb, J. Adhes., 2010, 80, 247–261 CrossRef.
- E. Gorb and S. Gorb, Entomol. Exp. Appl., 2009, 130, 222–228 CrossRef.
- J. M. Bullock and W. Federle, J. Exp. Biol., 2009, 212, 1876–1888 CrossRef PubMed.
- E. Gorb, K. Haas, A. Henrich, S. Enders, N. Barbakadze and S. Gorb, J. Exp. Biol., 2005, 208, 4651–4662 CrossRef CAS PubMed.
- E. V. Gorb, N. Hosoda, C. Miksch and S. N. Gorb, J. R. Soc. Interface, 2010, 7, 1571–1579 CrossRef CAS PubMed.
Footnote |
† Electronic supplementary information (ESI) available. See DOI: 10.1039/c9ra05198h |
|
This journal is © The Royal Society of Chemistry 2019 |