DOI:
10.1039/C9RA05085J
(Paper)
RSC Adv., 2019,
9, 28783-28792
Star-poly(ε-caprolactone) as the stationary phase for capillary gas chromatographic separation†
Received
4th July 2019
, Accepted 7th September 2019
First published on 13th September 2019
Abstract
This work presents the separation performance of star-poly(ε-caprolactone) (star-PCL) as the stationary phase for capillary gas chromatography (GC). The statically coated star-PCL column showed a column efficiency of 3345 plates per m and moderate polarity. Importantly, the star-PCL column exhibited high selectivity and resolving capability for more than a dozen mixtures covering a wide-ranging variety of analytes and isomers. Among them, the star-PCL column displayed advantageous resolving capability over the commercial DB-1701 column for aromatic amine isomers such as toluidine, chloroaniline and bromoaniline. Moreover, it was applied for the determination of isomer impurities in real samples, showing good potential in GC applications.
1. Introduction
Capillary gas chromatography (GC) has been widely used in petrochemical, environmental, food and pharmaceutical fields because of its inherent advantages of simplicity, high resolution, high selectivity, high sensitivity, short analysis time and low cost.1–5 In GC, the high-resolution separation of analytes mainly depends on the stationary phase, which is thus recognized to be the core of chromatographic analysis. Development of novel chromatographic stationary phases is a hot topic in GC. Hence, materials such as metal–organic frameworks, porous organic frameworks, covalent organic frameworks, graphene, and ionic liquids have all been investigated as GC stationary phases for molecular separations.6–11 The ideal GC stationary phase has the following excellent properties, such as high selectivity, good solubility and thermal stability, low glass transition temperature.12 Currently, polysiloxanes are the most common stationary phases in GC separations.13–15 The commercial polysiloxane columns achieved good resolution of most analytes in GC. However, few of them are capable of separating the tough isomer mixtures with the similar chemical nature and polarity. For these challenging analytical tasks, our group is constantly exploring new chromatographic separation materials with special selectivity in GC.16–19
Poly(ε-caprolactone) (PCL) is a saturated aliphatic polyester consisting of hexanoate repeating units.20 Its repeating unit contains five non-polar methylene (–CH2–) and one polar ester group (–COOC–), which makes the molecular chains relatively regular. PCL exhibits several unique features including hydrophobicity, semi-crystallinity, thermal stability, biocompatibility and flexible mechanical properties.20–23 It is widely applied in biomedical and pharmaceutical fields.24 Until now, however, only two reports on using linear PCL as stationary phases for chromatographic separations are available.25,26 According to the above researches, PCL as stationary phases have excellent chromatographic separation performance, especially for the separation of isomers with close nature. Therefore, their potential in chromatography is well worth being explored. The PCL glass and melting temperatures are about −60 °C and 55 °C, respectively.27 At room temperature, PCL is soluble in aromatic solvents and in chlorine solvents (dichloromethane and trichloromethane).28 Moreover, PCL forms blends with a wide variety of polymers conferring interesting and versatile physicochemical and mechanical properties to the new devices, such as three-dimensional architecture, porosity and stability in different media.29–33 In recent years, with the development of polymerization technology, more and more polymers with different specific functions and applications have been designed and synthesized.34–36 Among them, the star polymers have attracted attention due to their unique topological and physicochemical properties.37–39 Star polymers have at more than three linear polymer chains of approximately similar lengths joined together at one end of each chain to a chemically-bonded core.40 Star polymers, possessing better physicochemical properties than their linear counterparts, have, for example, lower glass-transition temperature, smaller viscosity, and higher number of functional end groups.41 With unique 3D structure and simple synthesis method, star-poly (ε-caprolactone) (star-PCL) has been widely concerned in polymer synthesis.42 Usually, star-PCL are synthesized from polyhydroxy alcohols as initiators, which constitute the core of the stars.42,43 Star-PCL is characteristic of 3D structure, abundant hydroxyl functional groups, high thermal stability, good solubility in organic solvents, and low glass transition temperature. Its unique structures and physicochemical properties inspired us to explore its potential as stationary phase for GC separations.
Herein, we present the investigation of star-PCL as stationary phase for GC separations (Scheme 1). First, the star-PCL capillary column was fabricated by static coating method and characterized for its column efficiency and polarity. Then, it was investigated for its separation performance and retention behaviours by utilizing more than a dozen mixtures covering a wide range of analytes and isomers. Simultaneously, for the separation of some challenging isomers, one commercial polysiloxane DB-1701 with close polarity were applied as the references. Finally, the star-PCL column was applied for the determination of isomer impurities in real samples.
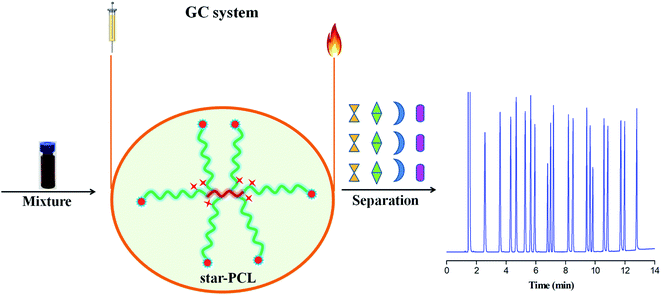 |
| Scheme 1 The star-PCL capillary column for GC separation. | |
2. Experimental
2.1. Materials and equipment
All the reagents and solvents employed were commercially available and were used as received without further purification. All the analytes were of analytical grade and dissolved in dichloromethane. Untreated fused-silica capillary tubing (0.25 mm, i.d.) was purchased from Yongnian Ruifeng Chromatogram Apparatus Co., Ltd. (Hebei, China). The commercial capillary column DB-1701 (30 m × 0.25 mm, i.d., 0.25 μm film thickness, 14% cyanopropylphenyl 86% dimethyl polysiloxane) was purchased from Agilent Technologies.
An Agilent 7890A gas chromatograph equipped with a split/splitless injector, a flame ionization detector (FID) and an autosampler was used for GC separations. All the separations were performed under the following GC conditions: nitrogen of high purity (99.999%) as carrier gas, injection port at 250 °C, split ratio at 60
:
1, FID detector at 250 °C. Oven temperature programs for the GC separations were individually provided in their figure captions. 1H NMR spectra were recorded on a Bruker Biospin 400 MHz instrument using TMS as the internal standard. IR spectra were recorded on a Bruker Platinum ART Tensor II FT-IR spectrometer.
2.2. Synthesis of the star-PCL stationary phase
The star-PCL was synthesized by the procedures described in Fig. 1.42 ε-Caprolactone (1.0 g, 9.0 mmol), D-Mannitol (31.8 mg, 0.18 mmol) and Sn(Oct)2 (30 mg, 0.08 mmol) were added to a 50 mL round-bottom flask. The mixture was stirred for 30 min at 25 °C under nitrogen and subsequently heated to 140 °C for 10 h. The resulting polymer was dissolved in THF (10 mL), precipitated in cold ethanol (20 mL) and dried under vacuum to obtain the white solid product (92% yield). Mp 50.4–53.1 °C; 1H NMR (400 MHz, CDCl3) δ: 4.06 (t, J = 6.8 Hz, 34H), 3.65 (t, J = 6.4 Hz, 2H), 2.31 (t, J = 7.6 Hz, 36H), 1.65 (dtt, J = 10.4, 6.8, 3.6 Hz, 75H), 1.46–1.32 (m, 36H); IR (KBr, cm−1): 1044.59(C–O), 1175.49(C–O), 1239.36(C–O), 1720.98(C
O), 2864.62(CH2), 2943.36(CH2). The NMR (Fig. S1†) and FT-IR (Fig. S2†) spectra of star-PCL were provided in the ESI.†
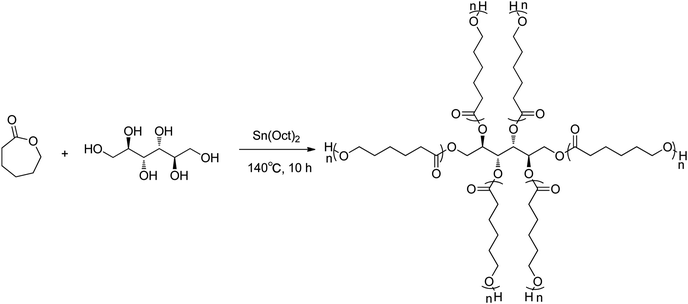 |
| Fig. 1 Synthesis of star-PCL. | |
2.3. Fabrication of the star-PCL capillary column
The star-PCL capillary column was fabricated by static coating method. Before coating, one bare fused-silica capillary column (10 m × 0.25 mm, i.d.) was pretreated with a saturated solution of sodium chloride in methanol for the inner surface roughening of the capillary column. The roughening procedure was performed with reference to the methods in ref. 21 and 44. Then, the solution was removed and the column was conditioned up to 200 °C and held for 3 h under nitrogen atmosphere. After the pretreatment, the column was statically coated with the solution of the star-PCL stationary phase in dichloromethane (0.25%, w/v) at room temperature. After the column was filled with the coating solution and sealed at one end, the solvent was evaporated at a steady speed from the other end under vacuum. At last, the column was conditioned from 40 °C to 180 °C at 1 °C min−1 and held at 180 °C for 7 h under nitrogen. The as-prepared star-PCL column was used for the following work.
3. Results and discussion
3.1. Characterization of the star-PCL column
The inherent thermal stability of the star-PCL stationary phase was evaluated by thermal gravimetric analysis (TGA). As shown in Fig. 2a, the star-PCL stationary phase showed about 5% weight loss at 273 °C, suggesting its good thermal stability and feasibility as the stationary phase for GC separations. To overall appraise the efficiency of the star-PCL column, its Golay curve was determined by measuring the height equivalent to a theoretical plate (HETP) of n-dodecane at different flow rates at 120 °C and the results are illustrated in Fig. 2b. As shown, the HETP attained the minimum of 0.29 mm at 0.30 mL min−1 for n-dodecane, corresponding to the column efficiency of 3345 plates per m. The high column efficiency can be attributed to the good solubility of the star-PCL stationary phase in the solvent for column fabrication, facilitating its uniform coating on the capillary wall. Moreover, the polarity of the star-PCL stationary phase was evaluated by McReynolds constants of five probe solutes, i.e., benzene (X′), 1-butanol (Y′), 2-pentanone (Z′), 1-nitropropane (U′) and pyridine (S′).45 Table 1 lists the general polarity and average polarity of the star-PCL stationary phase in comparison to the commercial DB-1701 stationary phase, suggesting its moderate polarity as GC stationary phase. In addition, the SEM images of the cross section and the inner coating of the star-PCL capillary column are shown in Fig. 2c and d, respectively, showing the uniform coating of the star-PCL stationary phase on the capillary column.
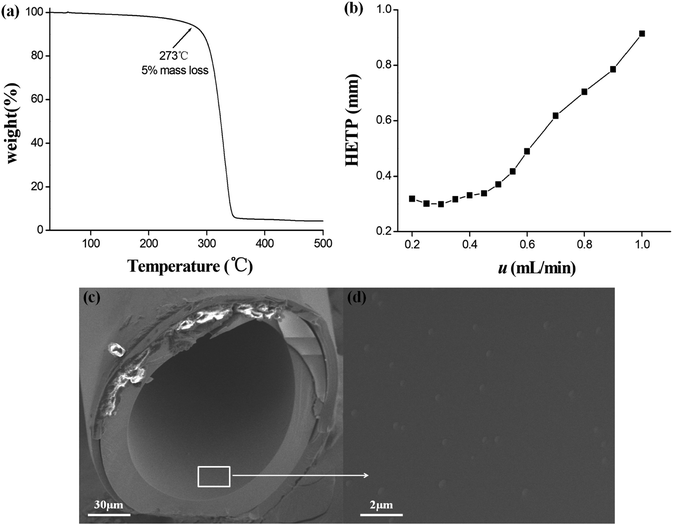 |
| Fig. 2 (a) TGA curves of the star-PCL stationary phase; (b) Golay curve of the star-PCL capillary column determined by n-dodecane at 120 °C; (c) and (d) SEM images showing the cross section and the inner coating of the star-PCL capillary column, respectively. | |
Table 1 McReynolds constants of the star-PCL and commercial columna
Stationary phases |
X′ |
Y′ |
Z′ |
U' |
S′ |
General polarity |
Average polarity |
X′, benzene; Y′, 1-butanol; Z′, 2-pentanone; U′, 1-nitropropane; S′, pyridine. Temperature: 120 °C. |
Star-PCL |
176 |
315 |
277 |
359 |
349 |
1476 |
295 |
DB-1701 |
308 |
395 |
379 |
358 |
280 |
1720 |
344 |
3.2. Separation capability and retention behaviours
The separation performance and retention behaviours of star-PCL column was evaluated by the mixture of 20 analytes of diverse types, fifteen groups of structural or positional isomers, and six groups of cis-/trans-isomers. Also, the DB-1701 column was employed for the separation.
To examine the separation performance of the star-PCL stationary phase for a more complex mixture, the mixture of 20 analytes of diverse types with varying polarity was employed and separated on the star-PCL column. Meanwhile, the commercial DB-1701 column was also used as reference for the evaluation. As can be seen from Fig. 3, the star-PCL column achieved baseline resolution (R > 1.5) for all the analytes whereas the DB-1701 column partially overlapped two pairs of the analytes, namely sec-butylbenzene/1,2,4-trimethylbenzene (peaks 5/6) and 2-nonanone/n-dodecane (peaks 9/7). Regarding the elution order, star-PCL column differs greatly from the polysiloxane column. Observably, compared to the DB-1701 column, the star-PCL column explicitly prolonged the retention for alcohols (1-octanol, 1-nonanol, 1-decanol, 1-undecanol, 1-decanol) and halogenated benzenes (1,4-dichlorobenzene, 1,2-dichlorobenzene, 1,3-dibromobenzene), suggesting their stronger interactions with these analytes through H-bonding and dipole–dipole interactions. Except for halogenated benzenes and alcohols, other analytes are eluted on the star-PCL column in the order of boiling points, thereby indicating that van der Waals interactions have a dominant role between star-PCL phase and analytes. In addition, the halogenated benzenes, alcohols and adjacent analytes are eluted on the star-PCL column against in the order of boiling point, such as n-dodecane/1,4-dichlorobenzene (peak 7/8, 215 °C/174 °C), 2-nonanone/1,2-dichlorobenzene (peak 9/10, 192 °C/180.4 °C), n-tetradecane/1,2,3-trichlorobenzene/1-nonanol (peak 12/13/14, 254 °C/218.5 °C/213 °C), n-hexadecane/1-undecanol (peak 17/18, 286.8 °C/241 °C), and 1-bromododecane/1-dodecanol (peak 19/20, 276 °C/260 °C). Subsequent halogenated benzenes or alcohols elution can be attributed to their strong H-bonding and dipole–dipole interactions with the OH groups of star-PCL stationary phase, as stated above. The results obtained above demonstrate the high-resolution performance and unique retention behaviours of the star-PCL stationary phase for analytes of diverse types with varying polarity.
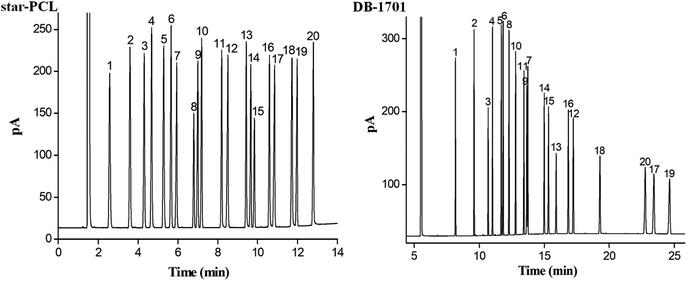 |
| Fig. 3 Separations of the mixture of 20 analytes of diverse types on the star-PCL and DB-1701 capillary columns. Peaks: (1) toluene, (2) ethylbenzene, (3) methyl hexanoate, (4) n-propylbenzene, (5) sec-butylbenzene, (6) 1,2,4-trimethylbenzene, (7) n-dodecane, (8) 1,4-dichlorobenzene, (9) 2-nonanone, (10) 1,2-dichlorobenzene, (11) 1-octanol, (12) n-tetradecane, (13) 1,2,3-trichlorobenzene, (14) 1-nonanol, (15) 1,3-dibromobenzene, (16) 1-decanol, (17) n-hexadecane, (18) 1-undecanol, (19) 1-bromododecane, (20) 1-dodecanol. Temperature program: 40 °C (1 min) to 160 °C at 10 °C min−1, flow rate at 0.6 mL min−1. | |
The above results proved the high resolving ability and different retention of star-PCL column for aromatic and aliphatic analytes. On this basis, we also investigated its capability for separations of diverse types of isomers, which is important for their analysis in chemical and environmental samples.44–47 As shown in Fig. 4a–j, the star-PCL column achieved baseline resolution (R > 1.5) of the structural or positional isomers ranging from nonpolar to polar nature with sharp peaks. Fig. 4a–c exhibits the separations of alkylated benzenes and naphthalenes isomers, such as propylbenzene, trimethylbenzene, and dimethylnaphthalene. The high resolution for these aromatic isomers demonstrated the extraordinary distinguishing capability of the star-PCL stationary phase through its slightly different dipole moments and polarizability with the analytes. Also, star-PCL column achieved excellent separations for the halogenated benzene isomers of dibromobenzene (Fig. 4d), dichlorobenzene (Fig. 4e), trichlorobenzene (Fig. 4f) and nitrochlorobenzene (Fig. 4g). All the isomers, especially the critical pairs of m-/p-dibromobenzene and m-/p-dichlorobenzene with b.p. difference less than 1 °C, were completely resolved, evidencing its high distinguishing capability for the analytes of high resemblance via their delicate differences in the strength of H-bonding and dipole–dipole interactions. Afterwards, its separation capability for the isomers of H-bonding type (benzaldehydes, alcohols and phenols) was explored. The separation results for nitrobenzaldehyde, butanol, and carvacrol/thymol are presented in Fig. 4h–j, respectively. Noteworthy, the star-PCL column achieved complete separation of the above isomers that are liable to severe peak-tailing and difficult to be well resolved, and sharp symmetrical peaks were obtained, suggesting its high-resolution performance and good column inertness.
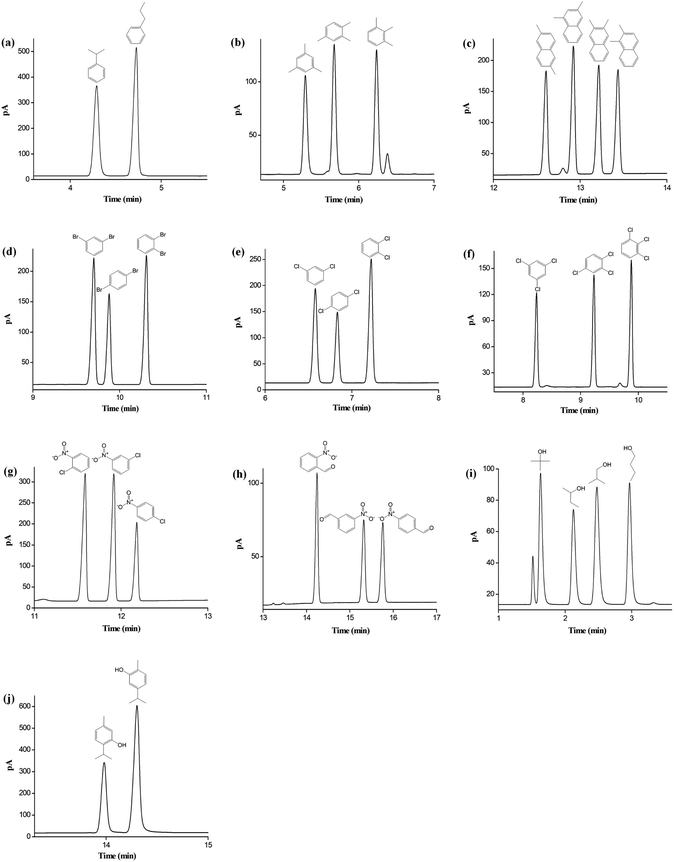 |
| Fig. 4 Separations of isomer mixtures of (a) propylbenzene, (b) trimethylbenzene, (c) dimethylnaphthalene, (d) dibromobenzene, (e) dichlorobenzene, (f) trichlorobenzene, (g) nitrochlorobenzene, (h) nitrobenzaldehyde, (i) butanol, and (j) carvacrol/thymol on the star-PCL column. Temperature program for all isomer mixtures: 40 °C (1 min) to 160 °C at 10 °C min−1, flow rate at 0.6 mL min−1. | |
Fig. 5 presents the separations of the challenging isomers consisting of xylenols, dichlorobenzaldehydes, and aromatic amines on the star-PCL column in comparison to the DB-1701 column. As shown, the star-PCL column well resolved all the analytes and showed advantageous separation capability over the DB-1701 column, especially for aromatic aniline isomers. Notably, some of the analytes partially overlapped or even coeluted on the DB-1701 column and showed different elution order. Compared to the DB-1701 stationary phase, the star-PCL stationary phase exhibits prolonged retention trend for 3,5-dimethylphenol and 3,5-dichlorobenzaldehyde over the adjacent analytes. The 3,5-dimethylphenol and 3,5-dichlorobenzaldehyde have larger van der Waals surface areas and thus exhibit stronger van der Waals interactions with the star-PCL stationary phase with the unique 3D conformation. Aromatic amine is a relatively important pollutant in the field of environmental analysis.48,49 The o-toluidine and p-chloroaniline are listed in 24 types of carcinogenic aromatic amine by the European Union. The affective separation of aniline isomers is a challenge in the analysis field, and GC is a common analytic method.50,51 However, aniline isomers often have to be derivated for effective separation, which is caused by the close physicochemical properties. As shown in Fig. 5, the star-PCL column achieved the baseline resolution (R > 1.5) for the toluidine, chloroaniline and bromoaniline isomers whereas the commercial polysiloxane column co-eluted or partially overlapped three analyte pairs, namely o-toluidine/p-toluidine, m-chloroaniline/p-chloroaniline and m-bromoaniline/p-bromoaniline.
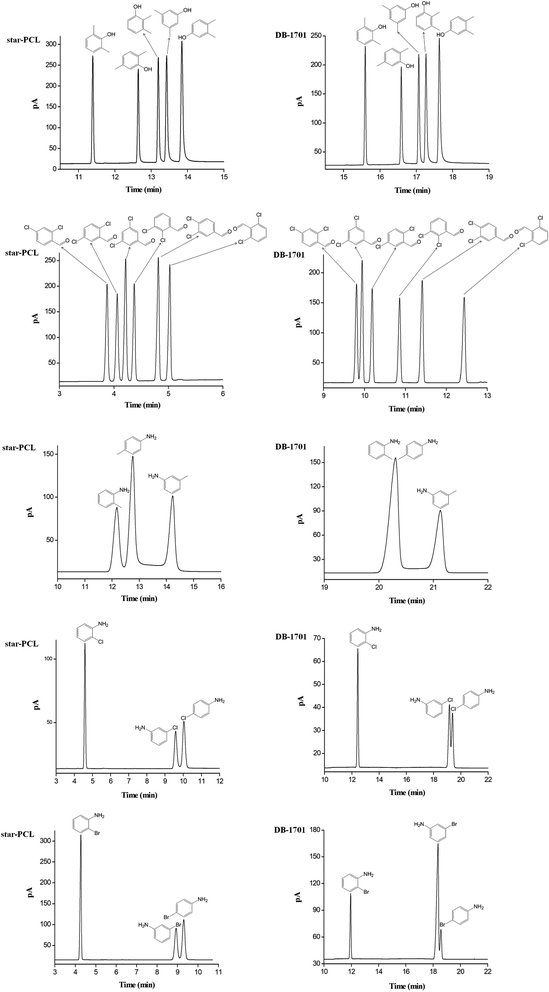 |
| Fig. 5 GC separations of dimethylphenol, dichlorobenzaldehyde, toluidine, chloroaniline and bromoaniline isomers on the star-PCL column in comparison to the DB-1701 column. Temperature programs for dimethylphenol: 40 °C (1 min) to 160 °C at 10 °C min; for dichlorobenzaldehyde: 120 °C (1 min) to 160 °C at 10 °C min; for toluidine: 100 °C; for chloroaniline: 125 °C; for bromoaniline: 140 °C. Flow rate at 0.6 mL min−1. | |
Afterward, the resolving ability of the star-PCL column was examined by the six cis-/trans-isomers containing aliphatic and aromatic isomers, including 1,3-dichloropropene, 1,2,3-trichloropropene, 2,5-dimethoxytetrahydrofuran, nerolidol, nerol/geraniol, and decahydronaphthalenes. As illustrated in Fig. 6, the star-PCL column also achieves high resolving ability for cis-/trans-isomers in a short time. Overall, the above results demonstrate the high resolving capability of the star-PCL stationary phase for diverse types of isomers with slight differences in structures and properties.
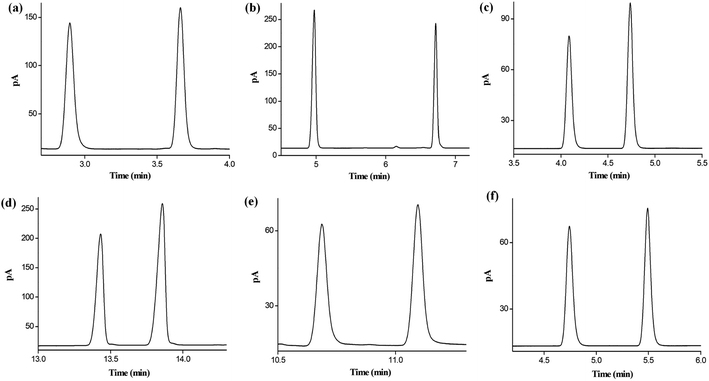 |
| Fig. 6 Separations of cis-/trans-isomers of (a) 1,3-dichloropropene, (b) 1,2,3-trichloropropene, (c) 2,5-dimethoxytetrahydrofuran, (d) nerolidol, (e) nerol/geraniol, and (f) decahydronaphthalene on the star-PCL column. Temperature program for all isomer mixtures: 40 °C (1 min) to 160 °C at 10 °C min−1, flow rate at 0.6 mL min−1. | |
3.3. Column thermal stability
Column thermal stability of star-PCL column was investigated by the separations of the trimethylbenzene, dichlorobenzene and trichlorobenzene isomers after the column was conditioned at each temperatures (180–240 °C at the increment of 20 °C) for 2 h.25,52 Fig. 7 illustrates the effect of column conditioning temperatures on the retention times of analytes. Notably, the retention times did not show dramatic decrease (RSD < 0.7%) over the temperature range up to 220 °C. Accordingly, the star-PCL column is suggested to be used below 220 °C so as to take full advantage of its separation performance and extend column life.
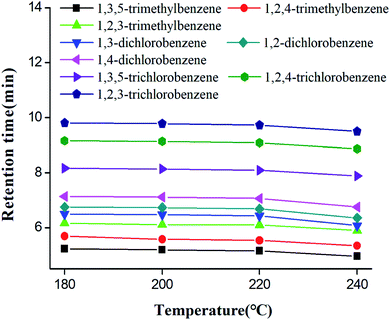 |
| Fig. 7 Thermal stability of star-PCL capillary column after the column was conditioned up to each of the indicated temperatures for 2 h. | |
3.4. Applications for the determination of isomer impurities in real samples
The star-PCL capillary column was employed to determine the possible isomer impurities in commercial reagent samples. Fig. 8 shows the results for the samples of nerol, geraniol, cis-decahydronaphthalene, and trans-decahydronaphthalene. As indicated, the star-PCL column well resolved the isomer impurities from the main component in each sample. Table 2 provides their assay results by the peak area normalization method. For cis-decahydronaphthalene, the contents of the major component and the isomer impurity were 98.70% and 1.29%, respectively. For trans-decahydronaphthalene, the corresponding contents were 99.54% and 0.45%. The results were in good agreement with the label purity for each sample.
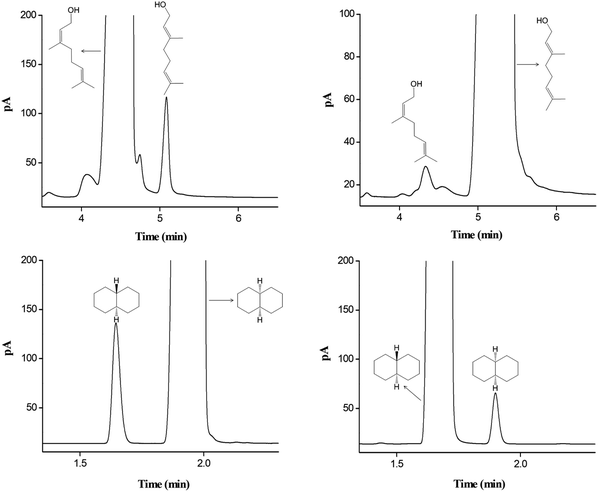 |
| Fig. 8 Applications of the star-PCL column for the determination of minor isomer impurities in the real samples of nerol, geraniol, cis-decahydronaphthalene, and trans-decahydronaphthalene, respectively. | |
Table 2 Applications of star-PCL column for the determination of isomer impurities in the real samples
Samples |
Labeled purity |
Measured purity |
Isomer impurity |
Content |
cis-Decahydronaphthalene |
98% |
98.70% |
trans-Decahydronaphthalene |
1.29% |
trans-Decahydronaphthalene |
98% |
99.54% |
cis-Decahydronaphthalene |
0.45% |
4. Conclusion
This work presents the example of exploring star-PCL material as stationary phase for GC separations. The star-PCL stationary phase has unique structure and favorable physicochemical features. Used as GC stationary phase, it exhibits good separation performance and column inertness for different types of analytes and their isomers (nonpolar/polar, aliphatic/aromatic). Compared with the polysiloxane DB-1701 column, the star-PCL column shows high resolution and different elution order for certain analytes. Particularly, it delayed the elution of alcohols and halogenated benzenes probably due to its stronger H-bonding and dipole–dipole interactions with these analytes. Importantly, it exhibited high selectivity and resolving capability for the tough isomers such as dichlorobenzaldehyde, toluidine, chloroaniline and bromoaniline, showing advantages over the polysiloxane column. Its high resolving capability mainly stems from the cooperative effect of its H-bonding, dipole–dipole, and dispersion in combination with its unique conformation. Considering that the maximum temperature of the star-PCL column is limited, PCL materials of higher thermal stability are needed to be further investigated and used to separate high boiling analytes. This work may encourage more research and applications of PCL materials in separation science.
Conflicts of interest
There are no conflicts to declare.
Acknowledgements
The work was supported by the National Natural Science Foundation of China (No. 21705072), Colleges and Universities in Henan Province Key Science and Research Project (No. 17A150039), Natural Science Foundation of Liaoning Province (20180550016).
References
- B. J. Pollo, G. L. Alexandrino, F. Augusto and L. W. Hantao, TrAC, Trends Anal. Chem., 2018, 105, 202–217 CrossRef CAS.
- A. M. Muscalu and T. Górecki, TrAC, Trends Anal. Chem., 2018, 106, 225–245 CrossRef CAS.
- M. V. Russo, P. Avino, L. Perugini and I. Notardonato, RSC Adv., 2015, 5, 37023–37043 RSC.
- C. Citti, D. Braghiroli, M. A. Vandelli and G. Cannazza, J. Pharm. Biomed. Sci., 2018, 14, 565–579 CrossRef.
- I. Špánik and A. Machyňáková, J. Sep. Sci., 2018, 41, 163–179 CrossRef.
- C. S. Hawes, Y. Nolvachai, C. Kulsing, G. P. Knowles, A. L. Chaffee, P. J. Marriott, S. R. Batten and D. R. Turner, Chem. Commun., 2014, 50, 3735–3737 RSC.
- A. Kewley, A. Stephenson, L. Chen, M. E. Briggs, T. Hasell and A. I. Cooper, Chem. Mater., 2015, 27, 3207–3210 CrossRef CAS.
- H. L. Qian, C. X. Yang and X. P. Yan, Nat. Commun., 2016, 8, 12104 CrossRef.
- X. Liang, X. Hou, J. H. M. Chan, Y. Guo and E. F. Hilder, TrAC, Trends Anal. Chem., 2018, 98, 149–160 CrossRef CAS.
- H. Nan and J. L. Anderson, TrAC, Trends Anal. Chem., 2018, 105, 367–379 CrossRef CAS.
- C. F. Poole and N. Lenca, J. Chromatogr. A, 2014, 1357, 87–109 CrossRef CAS.
- K. Dettmer-Wilde and W. Engewald, Practical Gas Chromatography-A Comprehensive Reference, Springer, New York, 2014, pp. 80–82 Search PubMed.
- C. Ruiz-Samblás, A. González-Casado and L. Cuadros-Rodríguez, Crit. Rev. Food Sci. Nutr., 2015, 55, 1618–1631 CrossRef.
- M. V. Russo, P. Avino and E. Veschetti, RSC Adv., 2015, 5, 10418–10423 RSC.
- N. E. Heshka, J. M. Choy and J. Chen, J. Chromatogr. A, 2017, 1530, 241–246 CrossRef CAS.
- T. Sun, H. Chen, X. Qiao, L. Ma, S. Hu and X. Liu, RSC Adv., 2018, 8, 34102–34109 RSC.
- J. Peng, T. Sun, L. Wu, M. Qi and X. Huang, RSC Adv., 2017, 7, 45408–45415 RSC.
- T. Sun, M. Qi and R. Fu, J. Sep. Sci., 2015, 38, 821–824 CrossRef CAS.
- T. Sun, L. Tian, J. Li, M. Qi, R. Fu and X. Huang, J. Chromatogr. A, 2013, 1321, 109–118 CrossRef CAS.
- M. Irfan, A. M. Bhayo, S. G. Musharraf and M. I. Malik, J. Sep. Sci., 2018, 41, 3352–3359 CrossRef CAS.
- M. Avella, F. Bondioli, V. Cannillo, E. D. Pace, M. E. Errico, A. M. Ferrari, B. Focher and M. Malinconico, Compos. Sci. Technol., 2006, 66, 886–894 CrossRef CAS.
- S. D. Mousavi, F. Maghsoodi, F. Panahandeh, R. Yazdian-Robati, A. Reisi-Vanani and M. Tafaghodi, Mater. Sci. Eng., C, 2018, 92, 631–643 CrossRef CAS.
- A. Pourjavadi, L. Dastanpour and Z. M. Tehrani, J. Nanopart. Res., 2018, 20, 282 CrossRef.
- T. K. Dash and V. B. Konkimalla, Mol. Pharmaceutics, 2012, 9, 2365–2379 CrossRef CAS.
- J. Peng, Y. Zhang, X. Yang and M. Qi, J. Chromatogr. A, 2016, 1466, 148–154 CrossRef CAS.
- J. Peng, Y. Shi, Z. Yang, M. Qi and F. Wang, J. Chromatogr. A, 2016, 1466, 129–135 CrossRef CAS.
- T. Rosen, I. Goldberg, W. Navarra, V. Venditto and M. Kol, Angew. Chem., Int. Ed., 2018, 130, 7309–7313 CrossRef.
- A. R. Pohlmann, F. N. Fonseca, K. Paese, C. B. Detoni, K. Coradini, R. C. R. Beck and S. S. Guterres, Expert Opin. Drug Delivery, 2013, 10, 623–638 CrossRef CAS.
- L. Shor, S. Güçeri, X. Wen, M. Gandhi and W. Sun, Biomaterials, 2007, 28, 5291–5297 CrossRef CAS.
- Q. Yao, J. G. L. Cosme, T. Xu, J. M. Miszuk, P. H. S. Picciani, H. Fong and H. Sun, Biomaterials, 2017, 115, 115–127 CrossRef CAS.
- J. H. Shim, J. h. Jeong, J. Y. Won, J. H. Bae, G. Ahn, H. Jeon, W. S. Yun, E. B. Bae, J. W. Choi, S. H. Lee, C. M. Jeong, H. Y. Chung and J. B. Huh, Biomed. Nanomater., 2017, 13, 015014 CrossRef.
- V. Guarino, F. Causa and L. Ambrosio, J. Appl. Biomater. Biomech., 2007, 5, 149–157 CAS.
- A. S. Hadj-Hamou, F. Metref and F. Yahiaoui, Polym. Bull., 2017, 74, 3833–3853 CrossRef CAS.
- Y. Zheng, S. Li, Z. Weng and C. Gao, Chem. Soc. Rev., 2015, 44, 4091–4130 RSC.
- Y. Zang, T. Aoki, M. Teraguchi, T. Kaneko, L. Ma and H. Jia, Polym. Rev., 2015, 55, 57–89 CrossRef CAS.
- S. D. Kimmins and N. R. Cameron, Adv. Funct. Mater., 2011, 21, 211–225 CrossRef CAS.
- X. Yang, L. Wang, W. Wang, H. Chen, G. Yang and S. Zhou, ACS Appl. Mater. Interfaces, 2014, 6, 6545–6554 CrossRef CAS.
- Y. Dai, H. Sun, S. Pal, Y. Zhang, S. Park, C. P. Kabb, W. D. Wei and B. S. Sumerlin, Chem. Sci., 2017, 8, 1815–1821 RSC.
- W. Wu, W. Wang and J. Li, Prog. Polym. Sci., 2015, 46, 55–85 CrossRef CAS.
- J. M. Ren, T. G. McKenzie, Q. Fu, E. H. H. Wong, J. Xu, Z. An, S. Shanmugam, T. P. Davis, C. Boyer and G. G. Qiao, Chem. Rev., 2016, 116, 6743–6836 CrossRef CAS.
- C. K. Balavigneswaran, S. K. Mahto, B. Subia, A. Prabhakar, K. Mitra, V. Rao, M. Ganguli, B. Ray, P. Maiti and N. Misra, Bioconjugate Chem., 2017, 28, 1236–1250 CrossRef CAS PubMed.
- P. Baheti, O. Gimello, C. Bouilhac, P. Lacroix-Desmazes and S. M. Howdle, Poly. Chem., 2018, 9, 5594–5607 RSC.
- A. M. Bhayo, R. Abdulkarim, S. G. Musharraf and M. I. Malik, RSC Adv., 2018, 8, 28569–28580 RSC.
- X. Xiong and M. Qi, J. Chromatogr. A, 2018, 1567, 191–197 CrossRef CAS.
- Y. Yang, Z. Chang, X. Yang, M. Qi and J. Wang, Anal. Chim. Acta, 2018, 1016, 69–77 CrossRef CAS.
- E. Pellattiero, A. Cecchinato, F. Tagliapietra, S. Schiavon and G. Bittante, J. Agric. Food Chem., 2015, 63, 963–974 CrossRef CAS.
- E. Kohyama, T. Chikumoto, H. Tada, K. Kitaichi and T. Ito, Forensic Toxicol., 2017, 35, 56–65 CrossRef CAS.
- H. Kataoka, J. Chromatogr. A, 1996, 733, 19–34 CrossRef CAS.
- S. Wang, Y. Cheng, M. Chen and K. Jiang, Eur. J. Mass Spectrom., 2018, 24, 337–343 CrossRef CAS.
- M. Chen, G. Zhu, S. Wang, K. Jiang, J. Xu, J. Liu and J. Jiang, J. Sep. Sci., 2018, 41, 440–448 CrossRef CAS.
- S. Wang, G. Zhu, M. Chen, J. Liu and K. Jiang, Eur. J. Mass Spectrom., 2016, 22, 127–132 CrossRef CAS.
- X. Yang, C. Li, M. Qi and L. Qu, J. Chromatogr. A, 2016, 1460, 173–180 CrossRef CAS.
Footnote |
† Electronic supplementary information (ESI) available. See DOI: 10.1039/c9ra05085j |
|
This journal is © The Royal Society of Chemistry 2019 |