DOI:
10.1039/C9RA04765D
(Paper)
RSC Adv., 2019,
9, 26291-26301
Extracellular expression of Aerococcus viridans pyruvate oxidase in recombinant Escherichia coli through SecB co-expression†
Received
25th June 2019
, Accepted 15th August 2019
First published on 21st August 2019
Abstract
Pyruvate oxidase (POD) is an important enzyme used for clinical applications and biochemical analyses, and recombinant Escherichia coli strains expressing Aerococcus viridans POD have been frequently employed for obtaining high POD yield. Although significant progress has been achieved in increasing recombinant POD production, intracellular POD expression and weak stability of POD make POD purification difficult. In this study, extracellular POD expression was achieved by co-expression of chaperone SecB under three promoters (T7, lac, bla). The weakest promoter, bla, when compared with T7 and lac promoters, provided the optimum extracellular POD activity among these three promoters. After optimization of cultivation conditions, such as IPTG concentration, pH, and temperature, the extracellular POD yield increased to 795.7 U L−1. Furthermore, by using glycine to disrupt recombinant E. coli cell wall and Cu2+ ions as POD stabilizer, the final extracellular POD yield reached 2926.3 U L−1. The expression intensity of chaperone had significant influence on heterologous protein secretion, and the high yield of extracellular POD implies potential widespread POD production and application.
Introduction
Pyruvate oxidase (POD) is an important enzyme with clinical and analytical industry applications.1 In recent years, POD from Aerococcus viridans has been successfully used as a reagent or biosensor in biochemical analyses owing to its good performance.2 However, the yield of POD from wild-type A. viridans is as low as 120 U L−1 (ref. 3), thus requiring novel technologies and processes to enhance the enzyme production. Escherichia coli is a well-established heterologous bacterial protein expression system with the advantages of easy genetic operation and cultivation.4 It has been reported that heterologous expression of A. viridans POD in recombinant E. coli strains achieved a high yield of 670 (ref. 5), 1078 (ref. 6), and 4106.9 U L−1 (ref. 7), promoting POD application in industries. However, intracellular expression of POD requires additional purification steps, when compared with secretory POD expression.8 Therefore, secretory expression of POD using various signal peptides is considered to be an ideal approach for achieving high POD production.9,10
Typically, extracellular fermentation broth is the target location of secretory protein from recombinant E. coli, which facilitates the heterologous proteins purification process.11 With the addition of glycine or TritonX-100, heterologous proteins can be easily transferred to the fermentation broth from periplasmic space.12 Furthermore, SecB dependent type II secretory system is the most common pathway for heterologous protein secretion, in which SecB, as a chaperone, combines with heterologous protein, assisting in its transportation.13,14 However, the amount of SecB in cells is relatively low;15 for example, the concentration of secB in E. coli K12 is about 1.6–2.5 μmol L−1 (ref. 16). Nevertheless, the SecB concentration can be altered, thus paving a way to enhance the secretion of heterologous protein. In a previous study, Kononova achieved a twofold increase in phoA secretion by increasing the concentration of SecB.17 Similarly, Zhou obtained 50% increase in human lymphotoxin expression in E. coli by co-expressing SecB.18 However, excessive chaperone expression could be a burden to the E. coli cells, leading to an increase in the formation of inclusion bodies.19 Therefore, regulation of SecB expression is an important step in secretory heterologous protein expression.
In the present study, a T7-promoted pod gene was co-expressed with three promoters (T7, lac, and bla) respectively that controlled SecB for POD secretory expression. The T7, lac, and bla promoters corresponded to strong, intermediate, and weak promoter activities, achieving 15.2–18.1, 0.28–0.84 (ref. 20), and 0.175–0.308 g L−1 h−1 heterologous proteins expression,21 respectively. The extracellular POD yield from recombinant E. coli was significantly increased through the regulation of SecB co-expression and cultivation conditions optimization, thus promoting industrial POD production.
Experimental
Medium
Luria–Bertani (LB) medium (1 L) was comprised 10.0 g of tryptone, 5.0 g of yeast extract, and 10.0 g of NaCl (pH 7.0). Terrific broth (TB) medium (1 L) consisted of 12 g of tryptone, 24 g of yeast extract, 4 mL of glycerol, 0.17 mol L−1 KH2PO4, and 0.72 mol L−1 K2HPO4 (pH 7.2).
Construction of recombinant E. coli strains for POD expression with SecB under different promoters
Four recombinant vectors (pRD-pod, pRD-pod-T7-secB, pRD-pod-lac-secB, and pRD-pod-bla-secB) were constructed for co-expression of SecB under T7, lac, and bla promoters with their terminators, respectively. Primers were listed in Table 1. pRD-pod was constructed based on pET25b-pod, which had been reported in our previous work.7 The pod gene (Gene ID: 32030008) with signal peptide pelB was obtained by PCR using primers pod-NdeI-F and pod-XhoI-R. Both pelB-pod fragment and pRSF-Duet1 fragment, after two enzymes (NdeI and XhoI) digestion and gel recovery, were ligated by T4 ligase at 16 °C overnight to obtain pRD-pod. To construct the recombinant vector pRD-pod-T7-secB, the secB gene was amplified using secB-BamHI-F and secB-NotI-R as primers from the E. coli BL21(DE3) genomic DNA. The amplified secB fragment and pRD-pod were digested with BamHI and NotI, and ligated using T4 ligase at 16 °C overnight to obtain pRD-pod-T7-secB. To construct the recombinant vector pRD-pod-lac-secB, the lac promoter was obtained using PCR with SphI-lac-F and lac-secB up-R as primers and pUC19 as template. The secB gene (ID: CP027060.1) was obtained using PCR with lac down-secB-F and TT-R as primers and E. coli BL21(DE3) genomic DNA as template. There was a 13-bp identity within the amplified 3′-lac and 5′-secB fragment ends which was combined by overlap extension PCR using SphI-lac-F and TT-SphI-R as out primers. The SphI-digested lac-secB was ligated with pRD-pod after SphI and calf-intestinal alkaline phosphatase (CIAP) digestion using T4 ligase at 16 °C overnight to obtain pRD-pod-lac-secB. To construct the recombinant vector pRD-pod-bla-secB, bla promoter was amplified using SphI-bla-F and bla-secB up-R as primers from pET25b vector. The secB gene was amplified using bla down-secB-F and TT-R as primers from the E. coli BL21(DE3) genomic DNA. The amplified bla and secB fragments were combined by overlap extension PCR using SphI-bla-F and TT-SphI-R as primers because their identical 14-bp nucleotide. The amplified bla-secB was digested using SphI and pRD-pod was digested with SphI and CIAP, and these two digested fragments were ligated using T4 ligase at 16 °C overnight to obtain pRD-pod-bla-secB.
Table 1 Primers sequences used in this study
Primers |
Sequences (5′-3′) |
pod-NdeI-F |
CCCATATGAAATACCTGCTGCCGACCGCTGC |
pod-XhoI-R |
GCGCTCGAGTTTGATGTATTTAGATTCTAAGCCTTC |
SphI-lac-F |
ACATGCATGCAAACGCCAGCAACGCGGCCTTTTTAC |
lac-secB up-R |
TTTGTTCTGACATAGCTGTTTCCTGTGTGAAATTG |
lac down-secB-F |
CACAGGAAACAGCTATGTCAGAACAAAACAACACTGAA |
TT-R |
ATTTCGATTATGCGGCCGTGTACAATACGATTACTTTCTGTTCGACTTAAGCATCAGGCATCCTGATGTTCTTCAGTACC |
TT-sphI-R |
GCGCATGCATTTCGATTATGCGGCCGTGTACAA |
secB-BamHI-F |
CGGGATCCAATGTCAGAACAAAACAACACTGAAATG |
secB-NotI-R |
TTGCGGCCGCTCAGGCATCCTGATGTTCTTCAGTA |
SphI-bla-F |
ATGCATGCCTGATAGACGGTTTTTCGCCCTT |
bla-secB up-R |
TTTGTTCTGACATACTCTTCCTTTTTCAATATTATTG |
bla down-secB-F |
GAAAAAGGAAGAGTATGTCAGAACAAAACAACACTGAA |
The four vectors were transformed into E. coli BL21(DE3) competent cells, and the recombinant E. coli BL21(DE3) strains were selected after incubation on LB plates containing 50 mg L−1 kanamycin (Kan) at 37 °C for 16 h. The four recombinant vectors were confirmed by NdeI and XhoI digestion for pRD-pod, BamHI, and NotI digestion for pRD-pod-T7-secB, SphI digestion for pRD-pod-lac-secB, and SphI digestion for pRD-pod-bla-secB, as well as through sequencing before and after transformation, respectively.
Effect of isopropyl β-D-1-thiogalactopyranoside concentration on POD expression in recombinant E. coli strains
The four recombinant E. coli strains (E. coli pRD-pod, E. coli pRD-pod-T7-secB, E. coli pRD-pod-bla-secB, and E. coli pRD-pod-lac-secB) were respectively inoculated into 250 mL flasks containing 100 mL of LB medium with 50 mg L−1 Kan and cultivated overnight at 37 °C and 200 rpm. Then, the fermentation broth was transferred (5% inoculum) into 250 mL flask containing 50 mL of TB medium (pH 7.2) and incubated at 37 °C and 200 rpm. After 2 h of cultivation, isopropyl β-D-1-thiogalactopyranoside (IPTG) was added to the culture at different concentrations (0.0015, 0.005, 0.015, 0.05, and 0.15 mmol L−1, respectively) and incubated for another 24 h at 25 °C and 200 rpm to induce POD expression. The cell densities and POD activities were determined at the end of cultivation.
Effect of pH on POD expression in recombinant E. coli pRD-pod-bla-secB
The recombinant E. coli pRD-pod-bla-secB was inoculated into 250 mL flask containing 100 mL of LB medium with 50 mg L−1 Kan and cultivated overnight at 37 °C and 200 rpm. Then, the culture broth was transferred (5% inoculum) into 250 mL flask containing 50 mL of TB medium with different pH values (5.0, 6.0, 7.0, 8.0, or 9.0) and incubated at 37 °C and 200 rpm. After 2 h of cultivation, IPTG was added at a final concentration of 0.05 mmol L−1 to the culture and incubated at 25 °C and 200 rpm for another 24 h to induce POD expression. The cell densities and POD activities were determined at the end of cultivation.
Effect of temperature on POD expression in recombinant E. coli pRD-pod-bla-secB
The recombinant E. coli pRD-pod-bla-secB was inoculated into 250 mL flask containing 100 mL of LB medium with 50 mg L−1 Kan and cultivated overnight at 37 °C and 200 rpm. Then, culture broth was transferred (5% inoculum) into 250 mL flask containing 50 mL of TB medium (pH 7.0) and incubated at 37 °C and 200 rpm. After 2 h of cultivation, IPTG was added at a final concentration of 0.05 mmol L−1 to the culture and incubated at 200 rpm and various temperatures (16 °C, 20 °C, 25 °C, and 30 °C, respectively) to induce POD expression. The cell densities and POD activities were determined every 12 h.
Effect of initial cell density on POD expression in recombinant E. coli pRD-pod-bla-secB
The recombinant E. coli pRD-pod-bla-secB was inoculated into 250 mL flask containing 100 mL of LB medium with 50 mg L−1 Kan, and cultivated overnight at 37 °C and 200 rpm. Then, the culture broth was transferred (5% inoculum) into 250 mL flask containing 50 mL of TB medium (pH 7.0) and incubated at 37 °C and 200 rpm. After the cell density reached 0.5, 2.5, and 4 OD600, IPTG was added at a final concentration of 0.05 mmol L−1 and incubated for another 24 h at 200 rpm and 25 °C to induce POD expression, respectively. As control (0 OD600), three 250 mL flasks containing 50 mL of TB medium (pH 7.0) were inoculated with recombinant E. coli cells from LB plate at the time of IPTG addition. The cell densities and POD activities were determined every 12 h.
Effect of glycine addition on POD expression in recombinant E. coli pRD-pod-bla-secB
The recombinant E. coli pRD-pod-bla-secB cells were transferred into 250 mL flask containing 100 mL of LB medium with 50 mg L−1 Kan, and cultivated overnight at 37 °C and 200 rpm. Then, the culture broth was transferred (5% inoculum) into 250 mL flask containing 50 mL of TB medium (pH 7.0) and cultivated at 37 °C and 200 rpm. After the cell density reached 0.5 OD600, IPTG (final concentration of 0.05 mmol L−1) and various concentrations of glycine (10, 30, and 60 g L−1, respectively) were added to the culture and incubated for another 24 h at 25 °C and 200 rpm to induce POD expression. The cell densities and POD activities were determined every 12 h.
Effect of metal ions on POD activity
The recombinant E. coli pRD-pod-bla-secB broth was centrifuged (7500 × g, 4 °C) for 5 min to obtain the supernatant POD solution. Then, metal ions (MnSO4, CuSO4, MgSO4, or CaSO4) at different concentrations (0.002 and 0.02 mmol L−1, respectively) were added to the POD solution and incubated at 25 °C in a water bath. The POD activities were determined at intervals.
Effect of Cu2+ ions addition on POD expression in recombinant E. coli pRD-pod-bla-secB
The recombinant E. coli pRD-pod-bla-secB was inoculated into 250 mL flask containing 100 mL of LB medium with 50 mg L−1 Kan, and cultivated overnight at 37 °C and 200 rpm. Then, the culture broth was transferred (5% inoculum) into 250 mL flask containing 50 mL of TB medium (pH 7.0) and incubated at 37 °C and 200 rpm. After the cell density of fermentation broth reached 0.5 OD600, a mixture containing IPTG (final concentration of 0.05 mmol L−1), glycine (final concentration of 30 g L−1), and different concentrations of Cu2+ (0.002, 0.02, and 0.2 mmol L−1, respectively) were added and incubated at 25 °C and 200 rpm to induce POD expression. The cell densities and POD activities were determined every 12 h.
Cell density determination
A total of 1 mL of recombinant E. coli fermentation broth was centrifuged at 7500 × g and 4 °C for 5 min. The cell pellet was washed twice and suspended in deionized water, and the absorbance was determined at 600 nm using a microplate reader (LMR-340M, Labexim International, Austria).
Protein concentration measurement and SDS-PAGE
A total of 10 mL of recombinant E. coli culture broth was centrifuged at 7500g and 4 °C for 5 min. The fermentation broth was collected for analysis. And the cell pellet was washed twice, and suspended in pH 6.8 0.1 mol L−1 phosphate-buffered saline (PBS) with a final volume of 10 mL. We obtained the soluble fraction after ultrasonication and centrifugation. We analyzed the protein content of soluble fraction and insoluble fraction after centrifugation by Bradford (1976). And SDS-PAGE was performed based on molecular cloning.22 The gel images were taken by a gel imaging system (Universal Hood II; Bio-Rad Laboratories, Inc., Hercules, CA, USA). The SecB band was analyzed using the BandScan 5.0 software (Glyko, Inc., Novato, CA, USA) according to its gray scale value.
POD activity assay
Total POD activity was calculated as the sum of intracellular and extracellular POD activities. To determine the intracellular and extracellular POD activities, a total of 1 mL of recombinant E. coli culture broth was centrifuged at 7500 × g and 4 °C for 5 min. The supernatant was used for extracellular POD activity determination, and the cell pellet was washed twice with 0.1 mol L−1 PBS (pH 6.8) and suspended in 0.1 mol L−1 PBS (pH 6.8). Then, the cells were disrupted by sonication for 4 s with a 6 s interval at 150 W for 3 min, and centrifuged at 12
000 × g and 4 °C for 5 min to obtain cell-free extract. The cell-free extract was used for intracellular POD activity determination.
For POD activity determination, a total of 100 μL of supernatant POD solution or cell-free extract were mixed with 1.0 mL of reaction solution (containing 0.1 mol L−1 sodium pyruvate, 0.015 mol L−1 magnesium chloride, 0.9% phenol, 1.5 mmol L−1 4-aminoantipyrine, 0.1 mol L−1 PBS (pH 6.8), 0.1 mmol L−1 flavin adenine dinucleotide, 1.0 mmol L−1 thiamine pyrophosphate, and 5 U mL−1 horseradish peroxidase) and incubated at 37 °C for 10 min. Subsequently, the absorbance of the solution was determined at 550 nm (OD550) by using a microplate reader (LMR-340M; Labexim International, Austria). One unit of POD activity was determined as the amount of enzyme required for the production of 1 μmol H2O2 per minute.
Results
Construction of recombinant E. coli strains for POD expression with SecB under different promoters
Four gene cassettes were constructed as shown in Fig. 1A. The pod gene of the four cassettes was expressed under a T7 promoter obtained from vector pET25b. The secB genes followed pod gene under T7, lac, and bla promoter in the three vectors pRD-pod-T7-secB, pRD-pod-bla-secB, and pRD-pod-lac-secB, respectively. These four vectors were transformed into E. coli BL21(DE3) cells to obtain four recombinant strains named E. coli pRD-pod, E. coli pRD-pod-T7-secB, E. coli pRD-pod-lac-secB, and E. coli pRD-pod-bla-secB, respectively. The genomic DNA of these four recombinant E. coli strains were used as templates to amplify the four gene cassettes (Fig. 1B). The sizes of the four gene fragments (pelB-pod, T7-secB, bla-secB, and lac-secB) were 1857, 548, 931, and 568 bp, respectively, which corresponded to the ideal sizes as designed. In addition, the recombinant vectors were also sequenced for verification.
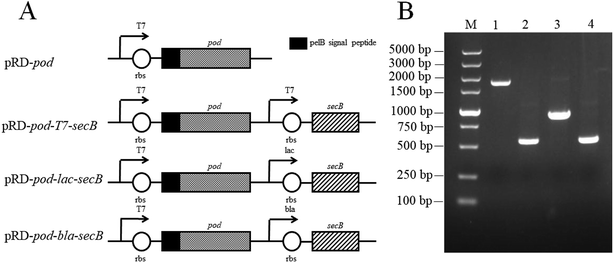 |
| Fig. 1 Construction of recombinant E. coli strains co-expressing pod and secB under different promoters ((A) sketch map of four gene cassettes; (B) DNA electrophoresis of four gene cassettes, M: DNA marker; 1: pelB-pod; 2: T7-secB; 3: bla-secB; 4: lac-secB). | |
Effects of IPTG concentration on POD expression in E. coli strains
The effects of IPTG concentration on the cell densities and POD activities of the four recombinant strains were presented in Fig. 2. The cell densities of E. coli pRD-pod, E. coli pRD-pod-T7-secB, E. coli pRD-pod-bla-secB, and E. coli pRD-pod-lac-secB decreased from 8.0 OD600 at 0 mmol L−1 IPTG to final cell densities of 1.6, 1.0, 1.2, and 1.1 OD600 at 0.15 mmol L−1 IPTG, respectively. The intracellular POD activities in the four recombinant E. coli strains showed bell-shaped profiles at IPTG concentration from 0 to 0.15 mmol L−1, with the highest values of 3093.0, 2631.4, 2629.2, and 2141.6 U L−1 obtained at 0.015 mmol L−1 IPTG for E. coli pRD-pod, E. coli pRD-pod-T7-secB, E. coli pRD-pod-bla-secB, and E. coli pRD-pod-lac-secB, respectively.
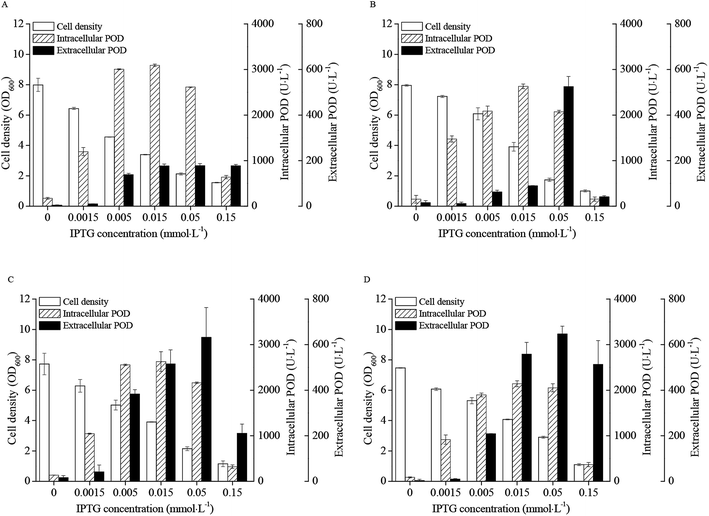 |
| Fig. 2 Effects of IPTG concentration on recombinant E. coli growth and POD expression (25 °C and 24 h of induction) ((A) pRD-pod; (B) pRD-pod-T7-secB; (C) pRD-pod-lac-secB; (D) pRD-pod-bla-secB). | |
The extracellular POD activity profiles differed among the four recombinant strains. The POD activities of E. coli pRD-pod increased with the increasing IPTG concentration, and reached 176.8 U L−1 at 0.015 mmol L−1 IPTG, and then remained stable till 0.15 mmol L−1 IPTG. In contrast, the POD activities of E. coli pRD-pod-T7-secB, E. coli pRD-pod-lac-secB, and E. coli pRD-pod-bla-secB increased to 525.0, 631.8, and 646.9 U L−1 with increasing IPTG concentration to 0.05 mmol L−1, and then significantly decreased to 41.1, 211.3, and 513.1 U L−1 at 0.15 mmol L−1 IPTG, respectively. These findings revealed that the extracellular POD activities increased in the presence of SecB, when compared with that noted in the control, indicating the positive effect of SecB on POD secretion from E. coli cells. Among the three recombinant E. coli strains co-expressing SecB under all concentrations of IPTG examined, the extracellular POD activities of E. coli pRD-pod-T7-secB presented the lowest value, whereas those of E. coli pRD-pod-bla-secB showed the highest value. These results indicated that the weak promoter (bla) produced the highest extracellular POD activity.
With the increasing concentration of IPTG, the total POD activity showed a bell-shaped profile. Co-expression of SecB produced a minor negative influence on total POD expression (Fig. S1A†), and T7 promoter controlled secB yielded the lowest total POD activities. In contrast, co-expression of secB under the lac and bla promoters led to higher extracellular POD expression, when compared with that noted in the control (Fig. S1B†). The extracellular POD activity in recombinant E. coli with bla-promoted secB was 24.0% and 58.2% of total POD activity at 0.05 and 0.15 mmol L−1 IPTG, respectively.
The SecB bands of different recombinant E. coli strains were analyzed with the results of Table 2 from their SDS-PAGE (Fig. S2†). The SecB expression level of E. coli pRD-pod-T7-secB increased from 193.4 mg L−1 to 469.6 mg L−1 when IPTG concentration increased from 0 mmol L−1 to 0.015 mmol L−1, and decreased to 357.0 mg L−1 and 0 mg L−1 at the condition of 0.05 mmol L−1 IPTG and 0.15 mmol L−1 IPTG. Under the lac promoter in E. coli pRD-pod-lac-secB, the SecB expression level increased from 67.1 mg L−1 to 173.4 mg L−1 when IPTG concentration increased from 0 mmol L−1 to 0.05 mmol L−1, and decreased to 79.8 mg L−1 at 0.15 mmol L−1 IPTG. Under the bla promoter in E. coli pRD-pod-bla-secB, the SecB expression level decreased from 14.1 mg L−1 to 1.9 mg L−1 continually when IPTG concentration increased from 0 mmol L−1 to 0.15 mmol L−1. At the both conditions of T7 promoter and lac promoter, there were SecB in fermentation broth, which showed the SecB leak from recombinant E. coli. There was not SecB at the condition of bla promoter which maybe attributed to low expression level of SecB. Therefore, the expression level of SecB was related inversely to the extracellular POD expression.
Table 2 The SecB expression levels in different recombinant E. coli strains
|
IPTG (mmol L−1) |
0 |
0.0015 |
0.005 |
0.015 |
0.05 |
0.15 |
E. coli pRD-pod |
Total protein in fermentation broth (mg L−1) |
126.0 |
137.6 |
282.3 |
438.8 |
334.3 |
123.5 |
Total protein in soluble fraction (mg L−1) |
714.7 |
774.6 |
380.7 |
576.3 |
286.0 |
164.6 |
Total protein in insoluble fraction (mg L−1) |
343.2 |
454.7 |
399.0 |
244.6 |
302.5 |
48.5 |
SecB in fermentation broth (mg L−1) |
— |
— |
— |
— |
— |
— |
SecB in soluble fraction (mg L−1) |
— |
— |
— |
— |
— |
— |
SecB in insoluble fraction (mg L−1) |
— |
— |
— |
— |
— |
— |
Total SecB (mg L−1) |
— |
— |
— |
— |
— |
— |
E. coli pRD-pod-T7-secB |
Total protein in fermentation broth (mg L−1) |
120.6 |
84.4 |
237.3 |
480.7 |
813.0 |
430.7 |
Total protein in soluble fraction (mg L−1) |
805.8 |
911.2 |
868.4 |
772.8 |
432.5 |
119.0 |
Total protein in insoluble fraction (mg L−1) |
407.5 |
166.4 |
407.5 |
482.6 |
247.8 |
125.7 |
SecB in fermentation broth (mg L−1) |
0.0 |
0.0 |
83.8 |
177.4 |
218.7 |
0.0 |
SecB in soluble fraction (mg L−1) |
155.5 |
261.5 |
289.2 |
258.9 |
132.3 |
0.0 |
SecB in insoluble fraction (mg L−1) |
37.9 |
25.6 |
35.5 |
33.3 |
5.9 |
0.0 |
Total SecB (mg L−1) |
193.4 |
287.1 |
408.4 |
469.6 |
357.0 |
0.0 |
E. coli pRD-pod-lac-secB |
Total protein in fermentation broth (mg L−1) |
138.7 |
179.6 |
297.6 |
403.9 |
653.1 |
628.1 |
Total protein in soluble fraction (mg L−1) |
788.0 |
816.6 |
684.4 |
592.4 |
278.9 |
141.3 |
Total protein in insoluble fraction (mg L−1) |
362.5 |
454.7 |
423.6 |
412.9 |
156.7 |
156.7 |
SecB in fermentation broth (mg L−1) |
0.0 |
31.2 |
72.9 |
80.8 |
162.6 |
79.8 |
SecB in soluble fraction (mg L−1) |
54.4 |
44.1 |
30.1 |
27.8 |
9.2 |
0.0 |
SecB in insoluble fraction (mg L−1) |
12.7 |
12.7 |
9.7 |
8.3 |
1.6 |
0.0 |
Total SecB (mg L−1) |
67.1 |
88.1 |
112.8 |
116.9 |
173.4 |
79.8 |
E. coli pRD-pod-bla-secB |
Total protein in fermentation broth (mg L−1) |
105.6 |
120.8 |
158.3 |
287.8 |
520.0 |
355.7 |
Total protein in soluble fraction (mg L−1) |
868.4 |
713.8 |
687.9 |
748.7 |
570.0 |
169.9 |
Total protein in insoluble fraction (mg L−1) |
380.7 |
442.9 |
465.4 |
386.1 |
290.7 |
127.8 |
SecB in fermentation broth (mg L−1) |
0.0 |
0.0 |
0.0 |
0.0 |
0.0 |
0.0 |
SecB in soluble fraction (mg L−1) |
0.0 |
0.0 |
0.0 |
0.0 |
0.0 |
0.0 |
SecB in insoluble fraction (mg L−1) |
14.1 |
10.6 |
10.7 |
6.6 |
4.7 |
1.9 |
Total SecB (mg L−1) |
14.1 |
10.6 |
10.7 |
6.6 |
4.7 |
1.9 |
Effect of pH on POD expression in E. coli pRD-pod-bla-secB
The pH value during IPTG induction phase had significant influence on cell densities and POD activities of E. coli pRD-pod-bla-secB (Fig. 3). Cell growth as well as intracellular and extracellular POD activities showed a bell-shaped curve at pH from 5.0 to 9.0, with highest values (3.7 OD600, 1940.3 U L−1, and 613.5 U L−1, respectively) noted at pH 7.0. Besides, the percentage of extracellular POD also showed a bell-shaped profile with the highest value of 24.0% achieved at pH 7.0 (Fig. S3†).
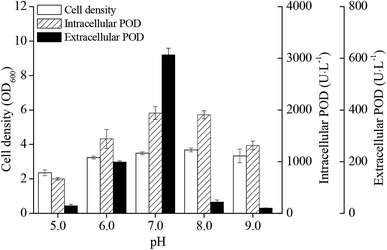 |
| Fig. 3 Effect of pH on POD expression in recombinant E. coli pRD-pod-bla-secB. | |
Effect of temperature on POD expression in E. coli pRD-pod-bla-secB
The effects of induction phase temperature on the cell densities and POD activities of recombinant E. coli pRD-pod-bla-secB were shown in Fig. 4. Overall, high cell density was obtained at 16–30 °C. The cell densities increased to 5.1–5.3 OD600 after 24 h of induction, and decreased to 4.1 and 3.4 OD600 with the cultivation time at 16 °C and 20 °C, respectively. Furthermore, at 25 °C and 30 °C, the cells showed diauxic growth as cell densities increased again after a decrease at 12 h of induction. The total POD activities of E. coli pRD-pod-bla-secB presented four bell-shaped curves within 48 h of induction with different peak values and corresponding relevant time points, respectively. Furthermore, the intracellular and extracellular POD activities at different temperatures exhibited bell-shaped profiles (Fig. 4C and D). For example, at 16 °C and 20 °C, the intracellular and extracellular POD activities reached their highest values after 36 h of induction, and significantly decreased. At 25 °C, the intracellular and extracellular POD activities reached their maximum values of 2137.1 and 629.8 U L−1, respectively, after 24 h of cultivation, and significantly decreased. At 30 °C, the intracellular and extracellular POD activities increased to their highest values of 278.8 and 33.6 U L−1, respectively, after 12 h of cultivation, and significantly decreased. Based on these findings, 25 °C was considered to be the ideal temperature for achieving maximum extracellular POD activity. Besides, at 25 °C, the extracellular POD activity contributed to the highest percentage of total POD activity at 36 h of cultivation.
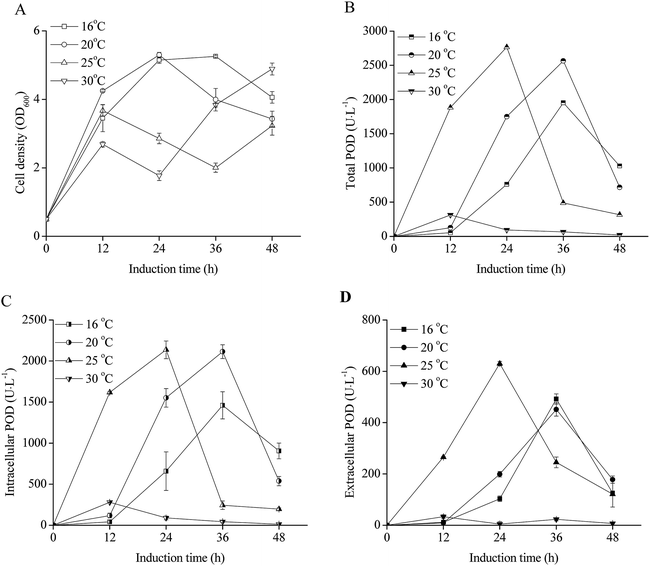 |
| Fig. 4 Effect of temperature on extracellular POD expression in recombinant E. coli pRD-pod-bla-secB ((A) cell density; (B) total POD activity; (C) intracellular POD activity; (D) extracellular POD activity). | |
Effect of initial cell density on POD expression in E. coli pRD-pod-bla-secB
The E. coli pRD-pod-bla-secB growth curves after IPTG induction at different initial cell densities were shown in Fig. 5A. The cell densities significantly increased following initial densities of 0 and 0.5 OD600, whereas remained relatively stable following initial densities of 2.5 and 4.0 OD600. The total POD activities presented bell-shaped curves with cultivation time (Fig. 5B), with the highest POD activity (3300.4 U L−1) noted at 2.5 OD600. Furthermore, while the intracellular POD activity showed bell-shaped curves (Fig. 5C), the extracellular POD activities exhibited various trends with relatively low POD activities obtained at initial cell densities of 0, 2.5, 4.0, and 9.0 OD600. The highest extracellular POD activity of 795.7 U L−1 was achieved after 24 h of induction with the cell density of 2.2 OD600 (Fig. 5A and D).
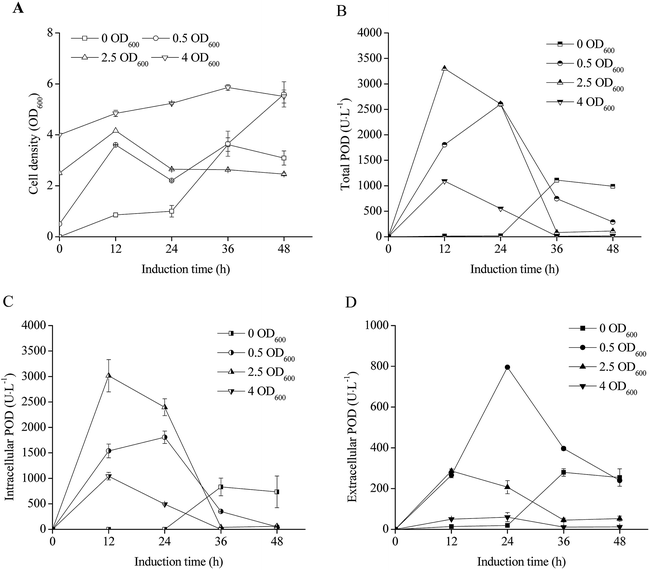 |
| Fig. 5 Effect of initial cell density on POD expression in recombinant E. coli pRD-pod-bla-secB ((A) cell density; (B) total POD activity; (C) intracellular POD activity; (D) extracellular POD activity). | |
Effect of glycine addition on POD expression in recombinant E. coli pRD-pod-bla-secB
The effects of glycine addition on E. coli pRD-pod-bla-secB cell density and POD expression are shown in Fig. 6. Although the negative influence of glycine on cell growth was significant as low cell density observed with increasing concentration of glycine (Fig. 6A), the total POD activities was enhanced at the condition of 10 g L−1 and 30 g L−1 glycine addition, which showed as bell-shaped curves as that of control (Fig. 6B). The highest total POD activity reached 3612.2 U L−1 at the condition of 30 g L−1 glycine after 24 h induction, which was 1.4 fold of control. For intracellular POD activity, glycine addition had a negative influence (Fig. 6C) because of higher extracellular POD activity was obtained at the condition of 10 g L−1 glycine and 30 g L−1 glycine addition (Fig. 6D). The highest extracellular POD activity (2759.6 U L−1) was obtained at 30 g L−1 glycine addition, which was 3.5 fold of control.
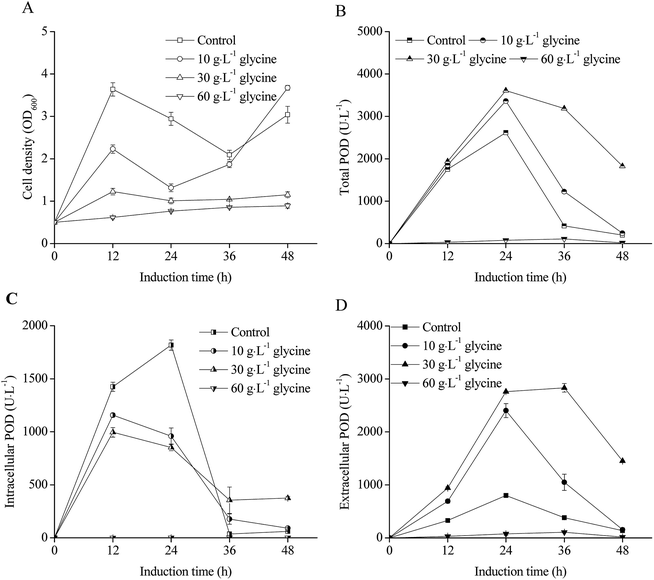 |
| Fig. 6 Effect of glycine on POD expression in recombinant E. coli pRD-pod-bla-secB ((A) cell density; (B) total POD activity; (C) intracellular POD activity; (D) extracellular POD activity). | |
Effect of metal ions addition on POD activity
The extracellular POD activities significantly decreased after 24 h of IPTG induction as shown in Fig. 4–6 owing to the weak stability of POD. Therefore, it was necessary to maintain POD as an active enzyme for achieving high POD production. Fig. 7 illustrates the effects of four metal ions on POD activities. Following addition of 0.02 mmol L−1 CuSO4, the residual POD activity remained 499.4 U L−1, when compared with that noted in the control (854.6 U L−1) after 18 h of cultivation (Fig. 7A). However, addition of MnSO4, MgSO4, and CaSO4 did not have any influence on POD stability (Fig. 7B–D).
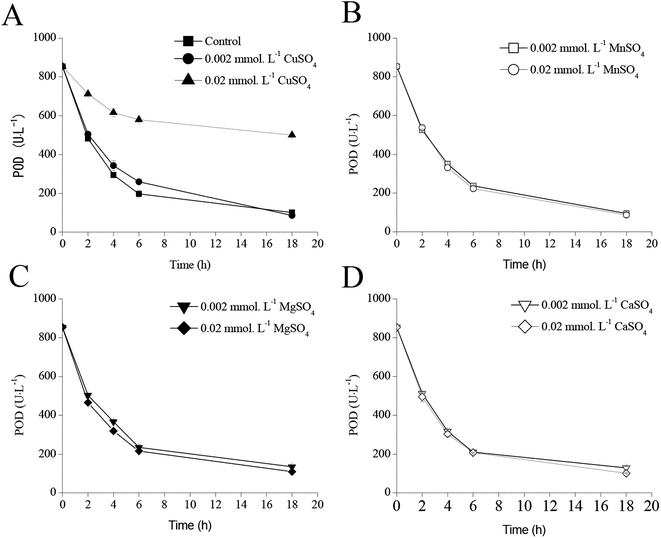 |
| Fig. 7 Effect of metal ions on POD stability ((A) CuSO4; (B) MnSO4; (C) MgSO4; (D) CaSO4). | |
Effects of CuSO4 addition on POD expression in recombinant E. coli pRD-pod-bla-secB
The effects of CuSO4 addition on cell growth and POD expression of recombinant E. coli pRD-pod-bla-secB were shown in Fig. 8. As indicated in Fig. 8A, CuSO4 addition had no significant influence on cell growth. The total POD activities presented similar curves before 24 h of IPTG induction, but exhibited varied trends after 24 h of induction (Fig. 8B). In the control of Fig. 8B, the total POD activities significantly decreased. Addition of 0.002 or 0.2 mmol L−1 CuSO4 resulted in lower decrease in total POD activities, whereas 0.002 mmol L−1 CuSO4 addition stabilized the total POD activities. Also, there was no significant different of intracellular POD activities at the condition of CuSO4 addition (Fig. 8C), with the POD activities increasing to 1224.9–1310.3 U L−1 after 12 h of IPTG induction, and subsequently decreasing to about 312.0–380.0 U L−1.
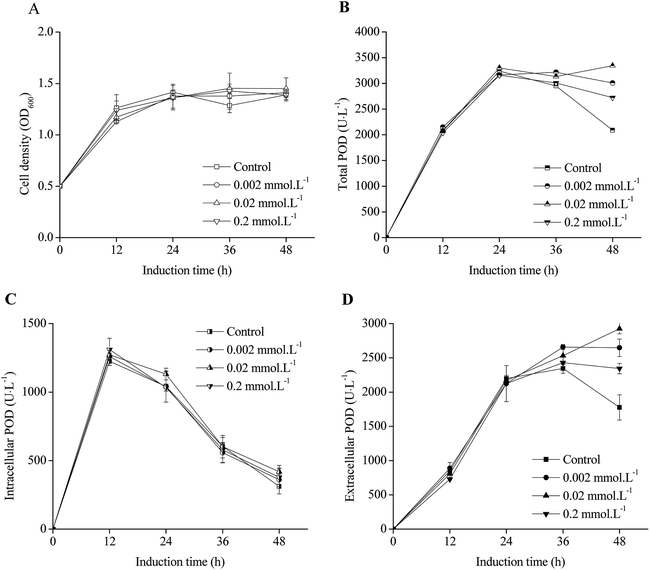 |
| Fig. 8 Effect of Cu2+ on POD expression in recombinant E. coli pRD-pod-bla-secB. Addition of 30 g L−1 glycine into E. coli pRD-pod-bla-secB cultivation was used as control ((A) cell density; (B) total POD activity; (C) intracellular POD activity; (D) extracellular POD activity). | |
The extracellular POD activities with CuSO4 addition in the first 24 h induction showed the same curve as that of control condition in which extracellular POD activities increased to 2124.7–2189.1 U L−1 (Fig. 8D). In the control condition, the extracellular POD activities decreased to 1776.8 U L−1 after 48 h of induction. In contrast, addition of 0.002, 0.02, and 0.2 mmol L−1 CuSO4 resulted in final extracellular POD activities to 26
483.0, 2926.3, and 2344.4 U L−1, respectively, indicating POD translocation from within the cell to the culture broth. The percentages of extracellular POD activities for all the experimental groups increased from 35–40% to 85–88%, and did not significantly differ with the addition of various concentrations of CuSO4 (Fig. S4†).
Discussion
In this study, secretory POD production was achieved through co-expression of SecB as a chaperone and optimization of cultivation conditions. The final POD yield was 2926.3 U L−1, which was the highest secretory POD yield, and 87.5% of total POD was secreted into the fermentation broth. These results demonstrated the potential of SecB, which has been reported as a multitasking chaperone.23 One of the SecB functions is to promote protein secretion from the cell. Among the three promoters examined in this study, the weakest promoter bla led to the highest secretory POD production. This result indicated worthy of tuning the ratio of heterologous protein and SecB for high secretory heterologous protein production from recombinant E. coli. As the diversity of heterologous proteins and chaperones investigated by researchers, it was necessary to test every single heterologous protein and chaperone considering the assistance of chaperon to heterologous protein secretory, and burden of chaperone to recombinant E. coli. Furthermore, secretory of POD activity decreased at the condition of further high IPTG loaded although the percentage of secretory POD increased with the increasing IPTG concentration (Fig. S1B†). The recombinant E. coli growth decreased at high IPTG concentration, indicating burden to the recombinant cell.24 To release this cell burden, several cultivation conditions were optimized for achieving high secretory POD production. At low temperature, the recombinant E. coli co-expressing SecB showed a growth difference (Fig. 4A), whereas high temperature exerted excess protein synthesis pressure on the recombinant cell.25 The high yield of extracellular POD obtained at 25 °C with a relatively low cell density suggested that SecB had a positive effect on POD peptide.17 To further increase the extracellular POD yield, glycine was added to release the POD within the cell, although glycine inhibited cell growth owing to cell leakage. The high yield of extracellular POD demonstrated the effect of glycine on protein leakage. Similar to previous studies that have optimized glycine concentration for achieving high protein leakage,26 the present study also attempted to optimize the concentration of glycine added to the culture broth to further increase extracellular POD production. On point should be emphasized that further POD was expressed at the condition of 30 g L−1 glycine because of stress of high POD in recombinant E. coli was released. This was supported by the higher total POD activities (Fig. 6B) and lower intracellular POD (Fig. 6C) obtained at the condition of 30 g L−1 glycine.
However, addition of Tween-20, Tween-80, and TritonX-100 was found to significantly interrupt POD activity. Besides, the weak stability of POD also significantly decreased POD activities. Therefore, the results of this study could only demonstrate the effect of cultivation conditions on residual POD activity. Moreover, Cu2+ ions stabilized POD by maintaining the tetramer structure of the enzyme as intermediate, and thus, high yield of extracellular POD was obtained with the addition of Cu2+ ions. In conclusion, the present study demonstrated an approach for secretory heterologous protein production by regulating the co-expression of SecB as chaperone.
Ethical statement
This article does not contain any studies with human participants or animals performed by any of the authors.
Conflicts of interest
Both authors declare they have no other competing interests.
Acknowledgements
Project supported by the National Natural Science Foundation of China (No. 31870045, 21306112).
References
- F. Lespinas, G. Dupuy, F. Revol and C. Aubry, Clin. Chem., 1989, 35, 654–658 CAS.
- M. Yasinzai, Int. J. Biol. Markers, 2009, 24, 107–111 CrossRef CAS PubMed.
- H. Misaki, K. Matsuura, S. Harada, S. Takenaka and Y. Horiuchi, US Pat., US4246342A, 1981.
- T. K. Singha, P. Gulati, A. Mohanty, Y. P. Khasa, R. K. Kapoor and S. Kumar, Process Biochem., 2017, 55, 17–31 CrossRef CAS.
- J. Zhao, Y. Wang, J. Chu, S. Zhang, Y. Zhuang and Z. Yuan, J. Ind. Microbiol. Biotechnol., 2008, 35, 257–262 CrossRef CAS PubMed.
- J. Zhao, Y. H. Wang, J. Chu, S. L. Zhang and Y. P. Zhuang, Journal of Changshu Institute of Technology, 2015, 29, 1–7 Search PubMed.
- J. Lu, Y. Zhao and J. Zhang, Lett. Appl. Microbiol., 2018, 67, 262–269 CrossRef CAS PubMed.
- J. Kaur, A. Kumar and J. Kaur, Int. J. Biol. Macromol., 2018, 106, 803–822 CrossRef CAS.
- M. A. Jonet, N. Muhammad Mahadi, A. M. Abdul Murad, A. Rabu, F. Abu Bakar, R. Abdul Rahim, K. O. Low and R. Illias, J. Mol. Microbiol. Biotechnol., 2012, 22, 48–58 CrossRef CAS PubMed.
- K. O. Low, N. M. Mahadi and R. M. Illias, Appl. Microbiol. Biotechnol., 2013, 97, 3811–3826 CrossRef CAS PubMed.
- F. Mergulhao, D. Summers and G. Monteiro, Biotechnol. Adv., 2005, 23, 177 CrossRef CAS PubMed.
- B. Li, L. Wang, L. Su, S. Chen, Z. Li, J. Chen and J. Wu, Biotechnol. Bioprocess Eng., 2012, 17, 1128–1134 CrossRef CAS.
- D. N. Collier, in Advances in Protein Chemistry, ed. C. B. Anfinsen, J. T. Edsall, F. M. Richards, D. S. Eisenberg and G. Lorimer, Academic Press, 1993, vol. 44, pp. 151–193 Search PubMed.
- M. N. Baeshen, A. Alhejin, R. S. Bora, M. M. Ahmed, H. A. I. Ramadan, K. S. Saini, N. A. Baeshen and E. M. Redwan, J. Microbiol. Biotechnol., 2015, 25, 953–962 CrossRef CAS PubMed.
- H. K. Seoh and P. C. Tai, J. Bacteriol., 1997, 179, 1077–1081 CrossRef CAS PubMed.
- B. T. Findik and L. L. Randall, PLoS One, 2017, 12, e0183231 CrossRef PubMed.
- S. V. Kononova, O. V. Khokhlova, S. N. Zolov and M. A. Nesmeyanova, Biochemistry, 2001, 66, 803–807 CAS.
- Y. Zhou, Q. Zhang, C. Yin, D. Song and Y. Chen, Chin. J. Biotechnol., 1997, 13, 433–436 CAS.
- Q. Jia, Y. e. Luo, D. Fan, P. Ma, X. Ma and W. Xue, J. Taiwan Inst. Chem. Eng., 2014, 45, 2843–2850 CrossRef CAS.
- C. Wang, J. Zhang, H. Wu, Z. Li and Q. Ye, J. Biotechnol., 2015, 214, 63–68 CrossRef CAS PubMed.
- J. Hou, Y. Liu, Q. Li and J. Yang, Protein Expression Purif., 2013, 88, 120–126 CrossRef CAS PubMed.
- Molecular Cloning: A Laboratory Manual, ed. M. R. Green and M. R. Green, Cold Spring Harbor Laboratory Press, Long Island, New York, 4th edn, 2012 Search PubMed.
- A. Sala, P. Bordes and P. Genevaux, Front. Microbiol., 2014, 5, 666 Search PubMed.
- P. Malakar and K. V. Venkatesh, Appl. Microbiol. Biotechnol., 2012, 93, 2543–2549 CrossRef CAS PubMed.
- A. Farewell and F. C. Neidhardt, J. Bacteriol., 1998, 180, 4704–4710 CAS.
- R. M. Bao, H. M. Yang, C. M. Yu, W. F. Zhang and J. B. Tang, Protein Expression Purif., 2016, 126, 9–15 CrossRef CAS PubMed.
Footnote |
† Electronic supplementary information (ESI) available. See DOI: 10.1039/c9ra04765d |
|
This journal is © The Royal Society of Chemistry 2019 |