DOI:
10.1039/C9RA03223A
(Paper)
RSC Adv., 2019,
9, 22161-22175
Processing milk causes the formation of protein oxidation products which impair spatial learning and memory in rats†
Received
30th April 2019
, Accepted 8th July 2019
First published on 17th July 2019
Abstract
This study explored the effects of protein oxidation during milk processing on spatial learning and memory in rats. Increasing the heating time, fat content, and inlet air temperature during processing by boiling, microwave heating, spray-drying, or freeze-drying increases milk protein oxidation. Oxidative damage done to milk proteins by microwave heating is greater than that caused by boiling. Dityrosine (DT), as a kind of tyrosine oxidation product, is the most important marker of this process, especially during spray-drying. Rats received diets containing either SWM (spray-dried milk powder diet), FWM (freeze-dried milk powder diet), FWM + LDT (freeze-dried milk powder + low dityrosine diet, DT: 1.4 mg kg−1), or FWM + HDT (freeze-dried milk powder + high dityrosine diet, DT: 2.8 mg kg−1) for 6 weeks. We found that the SWM group, the FWM + LDT group, and the FWM + HDT group appeared to have various degrees of redox state imbalance and oxidative damage in plasma, liver, and brain tissues. Further, hippocampal inflammatory and apoptosis genes were significantly up-regulated in such groups, while learning and memory genes were significantly down-regulated. Eventually, varying degrees of spatial learning and memory impairment were demonstrated in those groups in the Morris water maze. This means that humans should control milk protein oxidation and improve the processing methods applied to food.
1 Introduction
The Organization for Economic Co-operation and Development (OECD) and the Food and Agriculture Organization of the United Nations (FAO) pointed out in their agricultural outlook for 2018–2027 that world consumption of fresh dairy products and processed dairy products is poised to grow by 2.1% per annum and 1.7% per annum respectively, over this period.1 Dairy and dairy products have come to be an indispensable part of the lives of people all over the world. Milk is the most important raw material in dairy products. It is used in the processing of numerous foods because of its low price and rich nutritional value. Babies and the elderly are the largest consumers of milk powder. However, milk proteins are very prone to oxidative modification under conditions of high temperature, high pressure, shear, and radiation because milk contains a variety of pro-oxidant components, such as oxidizing enzymes, metal ions, unsaturated fatty acids, and flavors.2,3 These pro-oxidant components cause oxidative modification of the side-chain groups of the amino acid residues in milk proteins, which can cause the peptide chain to break4 and can lead to protein aggregation,5 cross-linking,6 and conformational changes,7 as well as changes in proteins' physicochemical properties, such as their solubility, hydrophobicity, water-holding capacity, and antioxidant ability, or even lead to the complete loss of protein function.8 Protein oxidation can also reduce the nutritional value of food due to the resultant increased content of carbonyl compounds and decreased essential amino acid content and protein digestibility.9 Oxidized protein products such as protein carbonyl (PC),10 sulfhydryl (SH),11 and dityrosine (DT)12 can be used as indicators of the extent of protein oxidation. The casein content of milk is about 80% of the total protein content,13,14 so the oxidation of tyrosine products is of primary concern. Our previous study found such tyrosine oxidation products, including 3-nitrotyrosine (3-NT), advanced oxidative protein products (AOPPs), and DT. DT is formed by the cross-linking of two tyrosine molecules, and is a major component of tyrosine's oxidation products.15 In addition, the oxidation of proteins promotes the oxidation of fats and produces fat oxidation products such as malondialdehyde (MDA), which in turn accelerate the oxidation, denaturation, and cross-linking of proteins.16,17 This leads to further a decrease in product quality, safety, and nutritional value.
After foods rich in these protein oxidation products are ingested, they first enter the digestive organs. In the cells of these digestive organs, protein oxidation products can cause protein degradation, misfolding, and deposition, which may lead to oxidative stress, making the body's redox state imbalanced and ultimately inducing a variety of diseases, including atherosclerosis,18 neurodegenerative diseases,19–21 and diabetes.22 Previous studies in our laboratory also explored some of the problems that can arise from this. Yang et al.23 and Li et al.21 found that dietary oxidized tyrosine could change many systemic metabolic processes, after mice were fed a diet containing oxidized tyrosine, oxidative injury in the liver, kidneys, and digestive organs occurred in the short-term, and oxidative stress, inflammation, and renal fibrosis through the JNK/p38/TGF-β1 signaling pathway were induced in them in the long-term. Results of a study by Ding et al.24–26 showed that exposure to dietary oxidized tyrosine products led to apoptosis in mice islet cells and MIN-6 cells, and also changes in thyroid hormone levels. Ran et al.27 showed that oxidative stress occurred in the hippocampus of mice following the gastrointestinal administration of DT to them, and damage also occurred to novel object recognition in these mice. The mRNA expression level of N-methyl-D-aspartate receptors (NMDAR) in the hippocampus and cerebral cortex of mice was significantly decreased by this treatment. Based on these studies, we proposed the following hypothesis: milk processing can cause protein oxidation that contributes to the formation of protein oxidation products, which can cause oxidative stress in the brain and hippocampus, leading to learning and memory impairment.
For this study, we have selected several milk processing methods, among which boiling is the most commonly used method. Microwave heating is the most common in eastern countries, especially China. Spray-drying and freeze-drying are the most commonly used milk powder processing methods. We compared the effects of different processing times, milk fat content and temperatures on milk oxidation in these four processing methods and confirmed the occurrence of oxidation by quantifying levels of PC, SH, DT, and MDA. In addition, we also carried out animal experiments by feeding rats diets containing milk powder that had undergone different degrees of oxidation. To determine the effects of oxidized milk proteins and their products, the redox state, cognitive and memory ability, and tissue gene and protein expression in the test animals were observed and verified by behavioral experiments.
2 Materials and methods
2.1 Materials
The BCA protein assay kit was purchased from Thermo Fisher Scientific (USA). The 2,4-dinitrophenylhydrazine (DNPH) used was acquired from Sinopharm Chemical Reagent Co., Ltd. (Suzhou, China). The 5,5-thio-2-nitrobenzoic acid (DNTB) used was obtained from Ming Han Biotechnology Co., Ltd. (Guizhou, China). Trizol was purchased from Vazyme Biotech Co., Ltd. (Nanjing, China). DT was synthesized by Ruidong Biotechnology Co., Ltd. (Hong Kong, China). Total cholesterol (TC), triglyceride (TG), high-density lipoprotein cholesterol (HDL-C), and low-density lipoprotein cholesterol (LDL-C) assay kits were purchased from Shanghai Fenghui medical science and technology Co., Ltd. Reduced glutathione (GSH), oxidized glutathione (GSSG), total antioxidant capacity (T-AOC), superoxide dismutase (SOD), and MDA assay kits were purchased from the Nanjing Jiancheng Bioengineering Institute (Nanjing, China). DT, AOPPs, and 3-NT Elisa kits were purchased from Xiamen Huijia Biotechnology Co., Ltd. (Xiamen, China). RIPA lysis buffer and 5× loading buffer were purchased from Beyotime Institute of Biotechnology (Shanghai, China). Primary antibodies Bcl-2, Bax, and β-actin were purchased from Abcam Co., Ltd (Shanghai, China); the secondary antibody was purchased from LI-COR Inc. (Lincoln, Nebraska, USA).
2.2 Milk samples treatment and determinations
Fresh milk was provided from cows by a local farmer directly after milking and was immediately cooled on ice.
2.2.1 Semi-skimmed milk preparation. Fresh milk was preheated to 40 °C and centrifuged at 5000 rpm for 30 min to separate the fat layer. The substrata were semi-skimmed milk (SM) and stored at 4 °C until use. The contents of fat and protein in whole milk (WM) and SM were measured by the Babcock method28 and Kjeldahl method,29 respectively. The results of these analyses are provided in Table 1.
Table 1 Fat and protein content in each groupa
Group |
Fat content (g/100 g) |
Protein content (g/100 g) |
WM, whole milk; SM, semi-skimmed milk. |
WM |
3.2 |
3 |
SM |
2.1 |
3.6 |
2.2.2 Preparation of boiling milk. Two-hundred mL of WM, SM were placed in 250 mL Erlenmeyer flasks. A small hole was drilled with a needle in the cap of the Erlenmeyer flasks to maintain equal air pressure within and outside the flasks. The milk samples were then boiled by placing the flasks in a 93 °C water bath, at atmospheric pressure, for 3, 6, or 12 min. Untreated milk was maintained as a control sample. After boiling, the milk samples were immediately cooled on ice.
2.2.3 Preparation of microwave heating milk. Two-hundred mL of WM and SM were placed in 250 mL Erlenmeyer flasks, and these are placed in the center of a microwave oven tray and heated for 40, 60, or 90 s in the microwave high power (1000 W).30 Untreated milk was also maintained as a control sample. After microwave heating, the milk samples were immediately cooled on ice.
2.2.4 Preparation of spray-dried milk powder. Spray drying milk powder was produced using the method as reported by Veronica et al.31 The spray drier was purchased by Wuxi Linzhou drying equipment Co., Ltd (Wuxi, China), which has a water evaporation capacity of about 1 L h−1. Drying air flow rate (80 m3 h−1), feed rate (7 × 10−4 m3 h−1), compressed air flow (2.4 m3 h−1), compressed air pressure (0.25 MPa). The air inlet temperature for WM spray-drying was 130, 140, 150, 160, 170, or 180 °C, and the air outlet temperature was 80 °C. The air inlet temperature for SM spray-drying was 170 °C and the air outlet temperature was 80 °C. The powders were collected and stored at −20 °C for further experiments.
2.2.5 Preparation of freeze-dried milk powder. Freeze drying refers to the method of Ashleigh et al.32 WM was frozen at −20 °C for 24 h to freeze the water in the milk fully, and then the freeze-dryer was started. Freeze-drying was carried out using a SCIENTZ-10N freeze-dryer (72 h, pressure < 10 kPa; SCIENTZ BIOTECHNOLOGY CO., LTD, Ningbo, China). The prepared milk powder was stored at −20 °C.
2.2.6 Determination. The level of PC in each sample was determined by the DNPH method.33 SH content was determined by the DNTB method.34 Methods to determine the DT and MDA content were carried out using kits, following the manufacturer's instructions. The data for each set of determinations was corrected with reference to the current protein concentration determined using the protein BCA kit.
2.3 Animal experiments
Animal use and protocol were reviewed and approved by the Jiangnan University Experimental Animal Management and Animal Welfare Ethics Committee.
2.3.1 Animal and diets. A total of 40 male 3 week-old Sprague-Dawley (SD) rats (weighing 53 ± 2 g) were obtained from Shanghai Slack Laboratory Animals Co., Ltd. (Shanghai, China). The rats were housed in cages containing 5 rats each, placed in an animal room at 22 ± 2 °C with a 12/12 h light/dark cycle, and provided with water ad libitum. The diets formula was slightly modified from the AIN-93M35 Spray-dried and freeze-dried whole milk powder fat and protein content are shown in ESI Table S1.† The ingredients of the four experimental diets are shown in ESI Table S2.† After 7 days of acclimatization, the rats were randomly divided into four groups:(1) The 180 °C spray-dried whole milk group (SWM): fed with 180 °C spray-dried whole milk powders diet (n = 10).
(2) The freeze-dried whole milk group (FWM): fed with freeze-dried whole milk powder diet (n = 10).
(3) The freeze-dried whole milk + low dityrosine group (FWM + LDT): fed with freeze-dried whole milk powder + low DT diet (DT: 1.4 mg kg−1) (n = 10).
(4) The freeze-dried whole milk + high dityrosine group (FWM + HDT): fed with 40% freeze-dried whole milk powder + high DT diet (DT: 2.8 mg kg−1) (n = 10).
180 °C inlet air temperature spray-dried WM powder content of 20 g/100 g protein, 26.5 g/100 g fat, and 1400 pg mg−1 prot DT. Frozen-dried WM powder content of 22 g/100 g protein and 26 g/100 g fat. FWM + LDT group added 5 times DT higher than the SWM group is 1.4 mg kg−1, FWM + HDT group add 10 times higher DT than the SWM group is 2.8 mg kg−1. Because the composition of the milk powder is complex, it is impossible to completely replicate the components to design a normal control group. Therefore, in this study, the FWM group was used as the control group. The body weight of the rats was measured every week. Food intake and water intake by the rats were detected at the end of week 6. Rats weight, food intake, and water intake are shown in Fig. 4.
2.3.2 Behavioral tests. The Morris water maze (MWM) test was applied as the behavioral tests used in this study, which is comprised of three parts: visible platform (day 1), hidden platform (days 2–5), and probe trial (day 6). The apparatus consisted of a round, black pool (210 cm in diameter, 50 cm in height), a platform (12 cm in diameter, 25 cm in height), high-contrast spatial cues on the interior of the pool,36 and a computerized tracking system with a recording video camera and software (Any-maze Behavior Tracking Software, Stoelting Co. Ltd., USA). The pool was filled with water (22 ± 2 °C) to a depth of 25 cm. The water was rendered opaque by adding black ink. The MWM test arena was divided into eight directions (N, S, W, E, NE, SE, WS, and NW) (Fig. 5A). On the day of the test (the 43rd day of the experiment), all rats in each group were trained to searching for the visible platform for 60 s. To begin testing, a small flag was first placed on the platform to ensure that the platform was visible. The rats were then gently placed into the water, facing the edge of the pool, and then the experimenters quickly left the testing area. If the rats found the platform before the 60 s cutoff, they were allowed to stay on the platform for 5 s and were then returned to their cages. If the rats did not find the platform within 60 s, they were placed on the platform and allowed it to stay there for 20 s before being returning to their cages.37 On days 2–5 (the 44th–47th days of the experiment), the flag was removed, and additional water was added to the pool to submerge the platform to 1 cm below the surface. The rats were then subjected to training and allowed to search for the hidden platform, as before. Each rat performed four training trails daily for four consecutive days with platform located in the same position throughout all trials. On day 6 (the 48th day of the experiment), the platform was removed from the pool and the rats were allowed to swim freely for 60 s from same start direction. The time spent in each quadrant and the number of rats crossing the location of the platform and on paths tracking toward it were used as the evidence of the acquisition of memory during training. MWM platform location and starting direction in day 1–6 are shown in ESI Table S3.†
2.3.3 Tissue collection and blood sampling. After the behavioral tests (end of the 7th week), rats were deeply anesthetized with an intraperitoneal injection of a solution including 2% pentobarbital sodium (60 mg kg−1). Blood samples were taken by cardiac puncture with heparinized tubes, and then these samples were centrifuged at 4 °C 3000 rpm for 20 min to separate out the plasma. The cerebral cortex, hippocampus, hypothalamus, amygdala, and liver were then removed, and tissue samples were immediately prepared as appropriate for each experimental technique.
2.3.4 Determination of lipid levels and oxidative stress status. Plasma TC, TG, HDL-C, and LDL-C were assayed by kits, which were measured as described by the manufacturer's instructions. The level of oxidative stress in the rats was determined by quantifying the levels of reactive oxygen species (ROS), T-AOC, SOD, and GSH/GSSG using their respective assays. The T-AOC and SOD activity in the plasma and liver were determined using kits, while following the manufacturer's instructions. The concentration of protein was measured using the BCA protein assay kit. The activities of GSH/GSSG in the plasma, liver, and hippocampus were determined using specific kits, following the manufacturer's instructions. ROS level was measured in the whole blood and liver by luminol-dependent chemiluminescence assay as described by Kobayashi et al.38 ROS production was expressed in terms of relative light units (RLUs).
2.3.5 Determination of oxidative damage. Liver and the left of the hippocampus were homogenized in ice-cold physiological saline to form tissue homogenate (tissue
:
saline ratio = 1
:
9) and centrifuged at 4 °C and 3500 rpm for 15 min to obtain the supernatant, which was then used in the subsequent tests. PC content was determined by the DNPH method;33 and the content of DT, 3-NT, and AOPPs was determined using their respective Elisa kits, while following the manufacturer's instructions. The concentration of protein was measured using the BCA protein assay kit.
2.3.6 Quantitative real-time polymerase chain reaction (qRT-PCR) analysis. The half right of hippocampus RNA was extracted by the Trizol method, and the RNA was reverse transcribed into cDNA using a Vazyme reverse transcription kit (Suzhou, China) and stored in a refrigerator at −20 °C. The cDNA was used as the template for qRT-PCR using SYBR green master mix (Vazyme, Suzhou, China). Gene expression was calculated relative to β-actin. The primer sequences used are shown in Table 2. The qRT-PCR used were as follows: stage 1, 1 cycle at 95 °C for 5 min; stage 2, 40 cycles at 95 °C for 10 s and 60 °C for 30 s; stage 3, one cycle at 95 °C for 15 s, 60 °C for 1 min, and 95 °C for 15 s.
Table 2 Sequences of primers used in qRT-PCR
Gene |
Forward (5′–3′) |
Reverse (5′–3′) |
Nrf2 |
GACCTAAAGCACAGCCAACACAT |
CTCTAATCGGCTTGAATGTTTGTC |
Prdx-1 |
TCATCTGGCATGGATTAACAC |
CGCTTGGGATCTGATACCA |
P38 |
CGAGCGATACCAGAACCT |
GGATTATGTCAGCCGAGTGT |
Nr1 |
GGTCAAGAAGGAGATTCCCA |
AGTCCTACTAGCAACCACAG |
Nr2a |
GTTGGTGATGGTGAGATGG |
ACTCATCACCTCATTCTTCTC |
Nr2b |
ATTTCTGCTCAGACTCTCACC |
GGAGGACTCATCCTTATCCG |
CaMK2A |
CGTGGACTGCCTGAAGAA |
GTGGTGTTGGTGCTCTCA |
CaMK2B |
TGGGATACCCACCTTTCTG |
GGGATGGGAAGTCATAGGC |
CREB |
GCAGTATATTGCCATTACCCA |
ATGGTTAATGTCTGCAGGC |
BDNF |
AATGGTGTCATAAAGTTCCACC |
GCAACCGAAGTATGAAATAACC |
Trkb |
CCAAGTTTGGCATGAAAGGTTTTG |
GCAACAGTAGTCCCAGGAGTT |
PSD-95 |
GCTCCCTGGAGAATGTGCTA |
TGGAATGTGTGTGGGAGAAA |
CytC |
GATTGACCAGGAAGCTGCAG |
CCACCAAAATCTCCTGCGTT |
Caspase-3 |
AACTCTTCATCATTCAGGCCT |
CCATATCATCGTCAGTTCCAC |
Caspase-9 |
ATGGACGAAGCGGATCGGCGGCTCC |
GCACCACTGGGGGTAAGGTTTTCTAG |
2.3.7 Western blot analysis. The remaining half of the right hippocampus Western blot analysis was performed as previously described.39 Hippocampus was homogenized in ice-cold RIPA buffer including 1 mmol L−1 phenylmethylsulfonyl fluoride. The mixture was incubated on ice for 30 minutes and then centrifuged (12
000 rpm, 5 min, 4 °C). After taking the supernatant, the protein was quantified by the BCA method. After the protein was leveled to the same concentration, 5× loading buffer was added. The mixture was then heated at 95 °C for 15 min and stored for later use. The mixture was separated by SDS-PAGE and transferred onto nitrocellulose membrane according to the standard scheme. Antibodies included Bax, Bcl-2, β-actin (Abcam, Shanghai, China) were used as primary antibodies. Then, the membrane was probed with secondary antibodies (goat anti-rabbit IgG, Lincoln, USA). Image Studio software was used to analyze the bands, and the expression ratios were normalized to that of β-actin.
2.4 Statistical analyses
All statistical analyses were performed using IBM SPSS Statistics 24. Graphs were drawn with GraphPad Prism software version 7.01 for Windows (GraphPad Software). The results were expressed as mean ± standard error of the mean (SEM) values. Differences among groups in the training trial sessions of the Morris water maze task (mean escape latency) were analyzed using a two-way repeated measures analysis of variance (ANOVA), followed by a Bonferroni test.40 Other datasets were compared among groups using the one-way ANOVAs followed by post hoc Tukey's test. P < 0.05 was defined as the threshold for statistical significance.
3 Results
3.1 Effects of heating methods on milk protein oxidation
The effects of milk fat content and heating time while boiling on milk protein oxidation are shown in Fig. 1. The potential effects of evaporation during boiling were neglected in this study due to minimal moisture volatilization being observed. Untreated milk was used as the control in this experiment. At the same fat content, levels of PC, DT, and MDA increased with increased boiling time, with the change in PC being the most significant among these (P < 0.05). Conversely, levels of SH were significantly reduced as boiling time increased (P < 0.05). When boiling time was increased from 3 to 12 min, the PC content in WM increased by 48%, and the SH content was reduced by 13%. When milk was boiled for the same amount of time, the levels of PC, DT and MDA in WM group were significantly highly than SM group (P < 0.05). Compared with the control, the WM after boiling significantly increased PC, DT, and MDA (P < 0.05), and significantly decreased SH (P < 0.05).
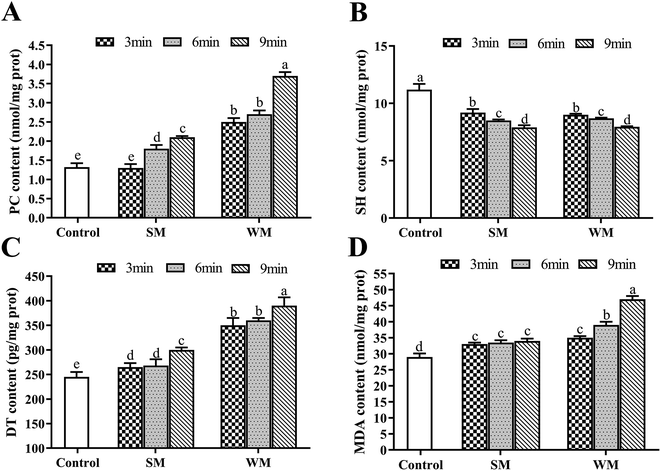 |
| Fig. 1 Effects of fat content and boiling time on PC, SH, DT and MDA in milk protein. (A) PC content in control, SM and WM; (B) SH content in control, SM and WM; (C) DT content in control, SM and WM; (D) MDA content in control, SM and WM. Control, untreated milk; SM, semi-skimmed milk; WM, whole milk; PC, protein carbonyl; SH, sulfhydryl; DT, dityrosine; MDA, malondialdehyde. Data are expressed as the mean ± SEM (n = 5 per group). Means among bars without a common letter differ, P < 0.05. | |
The effects of microwave heating on milk protein oxidation are shown in Fig. 2. Untreated milk was used as the control in this experiment. Compared with the control, the levels of PC, DT, and MDA in WM and SM significantly increased with microwave heating (P < 0.05) and increased more as the heating time was increased. As can be seen in Fig. 2C and D, the DT and MDA content of the milk samples increased as the fat content of the milk increased for the same microwave heating time. SH levels in the experimental groups were significantly lower than those in the control (P < 0.05) and tended to decrease as the heating time increased.
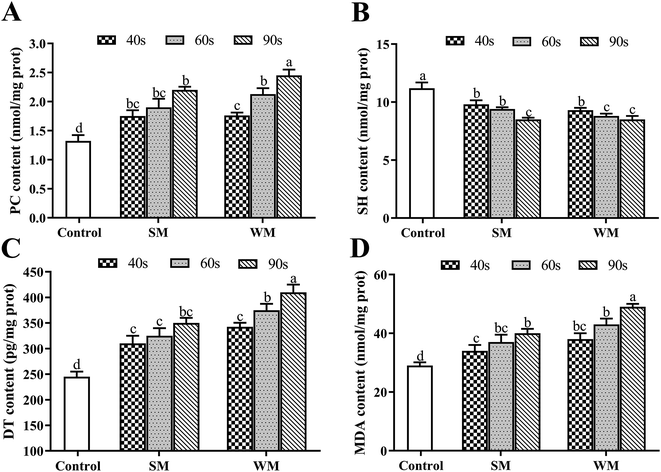 |
| Fig. 2 Effects of microwave heating on PC, SH, DT and MDA in milk protein. (A) PC content in control, SM and WM; (B) SH content in control, SM and WM; (C) DT content in control, SM and WM; (D) MDA content in control, SM and WM. Control, untreated milk; SM, semi-skimmed milk; WM, whole milk; PC, protein carbonyl; SH, sulfhydryl; DT, dityrosine; MDA, malondialdehyde. Data are expressed as the mean ± SEM (n = 5 per group). Means among bars without a common letter differ, P < 0.05. | |
3.2 Effects of drying treatments on milk protein oxidation
The results for different drying treatments are shown in Fig. 3. We observed an increase in the levels of PC, DT, and MDA and a decrease in those of SH in WM as the air inlet temperature during spray-drying increased, and these differences were significantly greater in the levels of PC and DT (P < 0.05). The increases in PC and DT levels in WM at the higher air inlet temperatures (160–180 °C) were much greater than those observed at the lower air inlet temperature (130–150 °C). We found that there were no significant differences in the content of PC, SH, DT, and MDA in WM and SM after spray-drying at 170 °C (P > 0.05). In addition, the content of PC and DT in milk after freeze-drying was significantly lower than that in milk after spray-drying at 180 °C (P < 0.05).
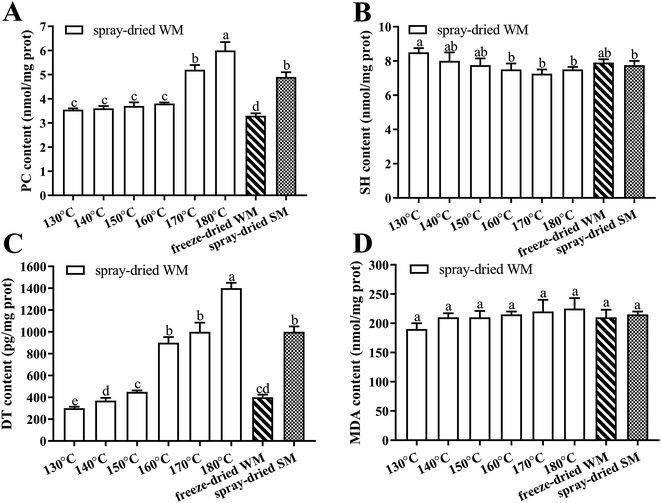 |
| Fig. 3 Effects of spray dried and freeze dried on the contents of PC, SH, DT and MDA. (A) PC content in spray-dried WM, frozen-dried WM and frozen-dried SM; (B) SH content in spray-dried WM, frozen-dried WM and frozen-dried SM; (C) DT content in spray-dried WM, frozen-dried WM and frozen-dried SM; (D) MDA content in spray-dried WM, frozen-dried WM and frozen-dried SM. 130–180 °C, air inlet temperature of WM spray drying; freeze-dried WM, freeze-dried whole milk; spray-dried SM, 170 °C air inlet temperature spray-dried semi-skimmed milk; PC, protein carbonyl; SH, sulfhydryl; DT, dityrosine; MDA, malondialdehyde. Data are expressed as the mean ± SEM (n = 5 per group). Means among bars without a common letter differ, P < 0.05. | |
3.3 Effects of diets containing milk powder at different degrees of oxidation on body weight, food intake, water intake, and plasma lipid levels in rats
We compared the initial body weight at 0th week and the final body weight at 6th week of the experiment between the rats in four experimental groups (Fig. 4A). There were no significant differences in body weight among the SWM, SFM, SFM + LDT, and SFM + HDT groups at the beginning of the experiment. All four groups showed a progressive increase in weight over the course of the experiment. However, from the 5th week to the end of the experimental protocol, the rats in the FWM + HDT group had significantly (P < 0.05) higher body weights compared to those in the FWM group. The body weight gain of the rats, measured from the 0th to the 6th week of the experiment, was found to have been significantly higher in the SWM, FWM + LDT, and FWM + HDT treatments than that in the FWM one (P < 0.05) (Fig. 4B). There were no statistically significant differences among the four groups in terms of their food intake and water intake (Fig. 4C and D), but the food intake did tend to decrease as the degree of oxidation of the milk included in the diet increased. We found that there was no significant difference in TC, TG, HDL-C and LDL-C between the four groups (Fig. 4E–H), but with the increase of dietary oxidation level, TC, TG and LDL-C showed an upward trend, and HDL-C showed a downward trend.
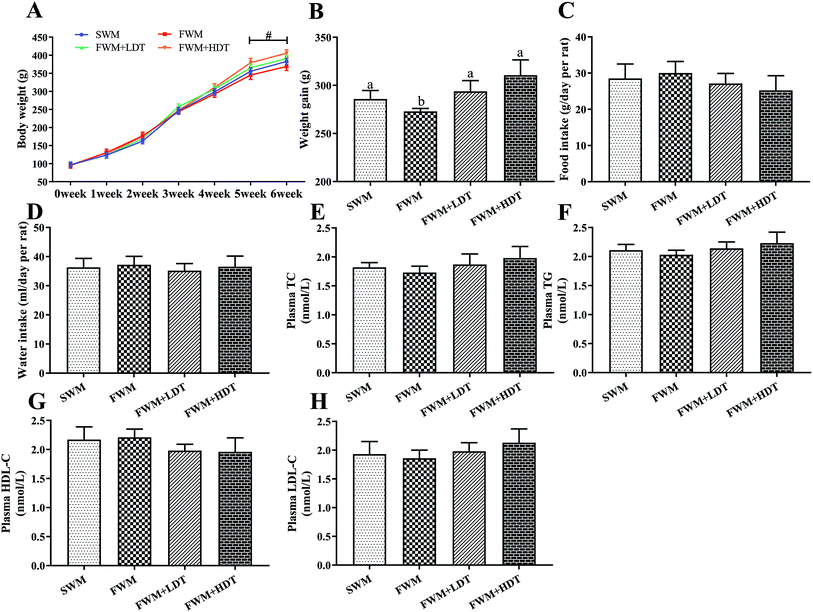 |
| Fig. 4 Effects of milk oxidation diet on body weight, food intake and water intake in rats. (A) Body weight, (B) body weight gain, (C) food intake at end of week 6, (D) water intake at end of week 6, (E) plasma TC content, (F) plasma TG content, (G) plasma HDL-C content, (H) plasma LDL-C content. SWM, 180 °C spray-dried whole milk group; FWM, freeze-dried whole milk group; FWM + LDT freeze-dried whole milk + low dityrosine group; FWM + HDT, freeze-dried whole milk + high dityrosine group; TC, total cholesterol; TG, triglyceride; HDL-C, high-density lipoprotein cholesterol; LDL-C, low-density lipoprotein cholesterol. Body weight gain of rats measured from 0th week until the 6th week in experiments. Data are expressed as the mean ± SEM (n = 10 rats per group). Significant levels at P < 0.05 were considered to indicate statistical significance. # indicates significantly different between the FWM + HDT and the FWM groups (P < 0.05). | |
3.4 Effects of diets containing milk powder at different degrees of oxidation on the long-term spatial memory of rats
The MWM test was used to assess whether there were any effects of milk protein oxidation on the long-term spatial memory of rats after they were fed with different diets containing oxidized milk powder. We found that there was no significant escape latency and speed on day 1 (P > 0.05) (Fig. 5B and C), which indicated that there were no problems with the exercise state and vision of the rats used. On days 2–5, the rats were trained to find platforms hidden underwater. We found that the escape latency continuously decreased over the 4 days of the training phase. However, in the last day of the hidden platform period, the latency of the FWM group was significantly lower than other groups (P < 0.05), and the latency of the SWM and FWM + LDT group was significantly lower than FWM + HDT group (P < 0.05) (Fig. 5D). As can be seen from the results for day 6, the rats in the FWM group stayed in the platform quadrant time, and the number of times they were crossing the platform area was significantly higher than that observed in the other groups (P < 0.05) (Fig. 5E and F). The same trend was also shown in the rats' trajectories on day 6 (Fig. 5G–J). In general, these results showed that diets containing more oxidized milk proteins impaired spatial learning in these rats, as well as their recall functions in the MWM test.
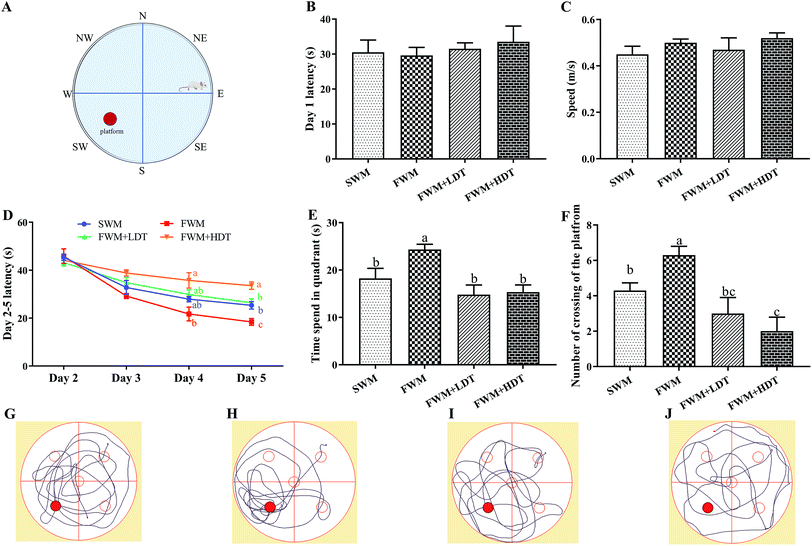 |
| Fig. 5 Effects of milk powder oxidation diet on the hippocampus-dependent spatial memory of SD rats in the Morris water maze (MWM) test. (A) Diagram of the MWM test, (B) latency in the visible platform task (day 1), (C) speed in the visible platform task (day 1), (D) latency in the hidden platform task (days 2–5), (E) time spend in quadrant, (F) number of crossing of the platform, (G) SWM tracking paths, (H) FWM tracking paths, (I) FWM + LDT tracking paths, (J) FWM + HDT tracking paths,. SWM, 180 °C spray-dried whole milk group; FWM, freeze-dried whole milk group; FWM + LDT freeze-dried whole milk + low dityrosine group; FWM + HDT, freeze-dried whole milk + high dityrosine group. Data are expressed as the mean ± SEM (n = 10 rats per group). Means among bars without a common letter differ, P < 0.05. | |
3.5 Effects of diets containing milk powder at different degrees of oxidation on oxidative stress status in rats
The levels of ROS (Fig. 6A and B) in the whole blood and liver, T-AOC (Fig. 6C and D) and SOD (Fig. 6E and F) activities in the plasma and liver, and GSH/GSSG levels (Fig. 6G) in the plasma, hippocampus, and liver were all assayed to evaluate the oxidative stress status in the tested rats. We observed a significant increase (P < 0.05) in the levels of ROS in the whole blood and liver of rats in the FWM + HDT group as compared to those in the FWM group. The ROS level in the liver of rats in the SWM group was significantly higher than that of rats in the FWM group (P < 0.05). There were significantly lower (P < 0.05) levels of T-AOC and SOD activity in the plasma and liver of rats in the FWM + HDT group as compared to those in rats of the FWM group. The GSH/GSSG levels in the plasma, hippocampus, and liver were significantly lower in rats in the FWM + HDT group than in those in the FWM group (P < 0.05). In general, as the degree of oxidation of the milk powder in the diet increased, the ROS levels in and oxidative stress status of the rats increased, while the antioxidant activity in them decreased.
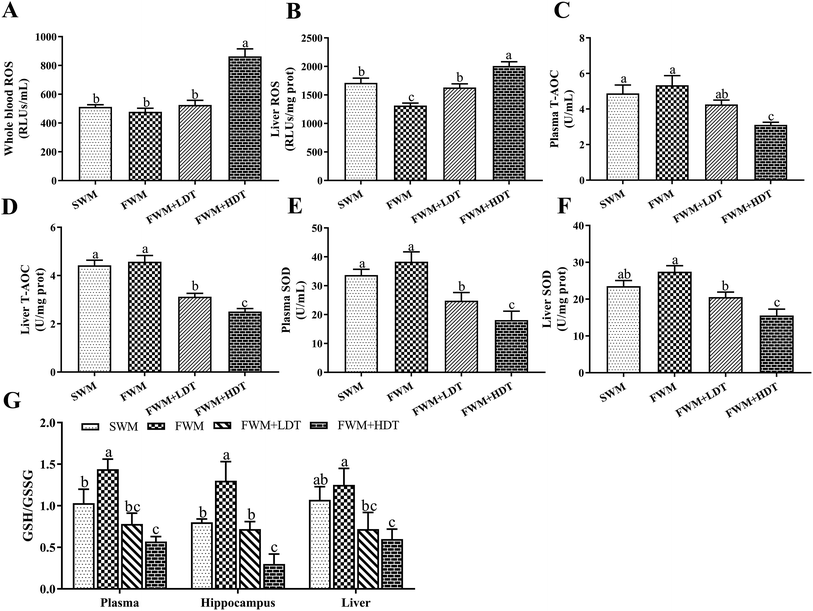 |
| Fig. 6 Effects of milk powder oxidation diet on the levels of oxidative stress status. (A) Whole blood level of ROS, (B) liver level of ROS, (C) plasma levels of T-AOC, (D) liver levels of T-AOC, (E) plasma levels of SOD, (F) liver levels of SOD, (G) plasma, hippocampus and liver level of GSH/GSSG. ROS, reactive oxygen species; T-AOC, total antioxidant capacity; SOD, superoxide dismutase; GSH, glutathione; GSSG, oxidized glutathione; SWM, 180 °C spray-dried whole milk group, FWM, freeze-dried whole milk group, FWM + LDT freeze-dried whole milk + low dityrosine group, FWM + HDT, freeze-dried whole milk + high dityrosine group. Data are expressed as the mean ± SEM (n = 10 rats per group). Means among bars without a common letter differ, P < 0.05. | |
3.6 Effects of diets containing milk powder at different degrees of oxidation on oxidative damage in rats
Table 3 illustrates how the ingestion of diets containing milk powder at different degrees of oxidation led to oxidative damage to the plasma, brain tissue, and liver of the tested rats, where protein oxidation product deposits were formed. In the hippocampus, hypothalamus, and liver, the PC content of rats in the SWM group was significantly higher than that in those of the FWM group (P < 0.05). In the plasma and hypothalamus, the DT deposition was significantly greater in the SWM group than that in the FWM group (P < 0.05). In the hippocampus and amygdala, the 3-NT content in the SWM group was significantly higher than that in the FWM group (P < 0.05). In the plasma, hippocampus, cerebral cortex, and liver, the content of AOPPs in the SWM group was significantly higher than that in the FWM group (P < 0.05). The DT and 3-NT content in the plasma and all other tissues of the FWM + HDT group was significantly higher than that in the FWM (P < 0.05) and SWM groups (P < 0.05). The PC and AOPP content in the plasma and all other tissues of the rats in the FWM + HDT group was significantly higher than that in the FWM + LDT (P < 0.05), FWM (P < 0.05), and SWM groups (P < 0.05). The DT content in the plasma, hippocampus, hypothalamus, cerebral cortex, and liver of rats in the FWM + HDT group was significantly higher than that in rats of the FWM + LDT group (P < 0.05). In general, the amount of oxidative damage done to the body and the content of PC, DT, AOPPs, and 3-NT in the plasma, brain tissue, and liver tended to increase as the degree of oxidation of the milk powder included in the diet increased.
Table 3 Oxidative damage in plasma, liver and brain tissuesa
|
SWM |
FWM |
FWM + LDT |
FWM + HDT |
PC, protein carbonyl; DT, dityrosine; 3-NT, 3-nitrotyrosine; AOPPs, advanced oxidative protein products; SWM, 180 °C spray-dried whole milk group, FWM, freeze-dried whole milk group, FWM + LDT freeze-dried whole milk + low dityrosine group, FWM + HDT, freeze-dried whole milk + high dityrosine group. Data are expressed as the mean ± SEM (n = 10 rats per group). Means among bars without a common letter differ, P < 0.05. |
Plasma |
PC (nmol l−1) |
2.16 ± 0.11bc |
1.95 ± 0.15c |
2.79 ± 0.45b |
3.82 ± 0.23a |
DT (pg mL−1) |
106.91 ± 5.12b |
68.56 ± 9.35c |
129.14 ± 9.86b |
171.92 ± 3.32a |
3-NT (nmol l−1) |
15.34 ± 2.78b |
12.86 ± 1.77b |
17.15 ± 3.65b |
27.19 ± 2.12a |
AOPPs (pmol l−1) |
276.61 ± 25.18b |
208.32 ± 12.66c |
317.86 ± 18.47b |
448.82 ± 11.35a |
![[thin space (1/6-em)]](https://www.rsc.org/images/entities/char_2009.gif) |
Hippocampus |
PC (nmol g−1 prot) |
3.83 ± 0.23b |
3.02 ± 0.25c |
4.35 ± 0.35b |
5.77 ± 0.21a |
DT (pg mg−1 prot) |
109.45 ± 8.45c |
78.50 ± 3.36d |
149.45 ± 7.11b |
178.66 ± 8.90a |
3-NT (nmol g−1 prot) |
61.42 ± 1.17b |
50.31 ± 5.69c |
77.38 ± 7.12a |
84.77 ± 6.32a |
AOPPs (pmol g−1 prot) |
373.43 ± 17.53c |
243.65 ± 12.36d |
454.32 ± 15.69b |
507.77 ± 11.31a |
![[thin space (1/6-em)]](https://www.rsc.org/images/entities/char_2009.gif) |
Hypothalamus |
PC (nmol g−1 prot) |
3.01 ± 0.12b |
2.26 ± 0.17c |
3.52 ± 0.45b |
4.97 ± 0.31a |
DT (pg mg−1 prot) |
38.17 ± 4.11b |
25.86 ± 3.21c |
40.76 ± 11.75b |
67.23 ± 7.32a |
3-NT (nmol g−1 prot) |
48.46 ± 2.68b |
45.06 ± 4.12b |
53.07 ± 2.32b |
68.21 ± 3.11a |
AOPPs (pmol g−1 prot) |
230.75 ± 19.14bc |
215.57 ± 12.36c |
269.10 ± 7.13b |
343.34 ± 21.36a |
![[thin space (1/6-em)]](https://www.rsc.org/images/entities/char_2009.gif) |
Amygdala |
PC (nmol g−1 prot) |
2.86 ± 0.23b |
2.66 ± 0.15b |
2.99 ± 0.29b |
3.55 ± 0.14a |
DT (pg mg−1 prot) |
94.21 ± 8.19ab |
76.20 ± 10.16b |
104.32 ± 4.34a |
118.77 ± 15.75a |
3-NT (nmol g−1 prot) |
41.03 ± 1.98c |
36.34 ± 2.11d |
50.35 ± 3.05b |
61.31 ± 1.25a |
AOPPs (pmol g−1 prot) |
262.64 ± 17.69b |
241.94 ± 26.68b |
263.60 ± 12.36b |
436.44 ± 18.32a |
![[thin space (1/6-em)]](https://www.rsc.org/images/entities/char_2009.gif) |
Cerebral cortex |
PC (nmol g−1 prot) |
2.45 ± 0.16c |
2.64 ± 0.17c |
3.26 ± 0.33b |
4.15 ± 0.24a |
DT (pg mg−1 prot) |
98.18 ± 10.31bc |
79.45 ± 5.08c |
113.86 ± 2.47b |
140.68 ± 10.06a |
3-NT (nmol g−1 prot) |
37.62 ± 2.12c |
32.15 ± 1.51c |
48.23 ± 3.08b |
56.12 ± 2.54a |
AOPPs (pmol g−1 prot) |
346.14 ± 7.98c |
289.22 ± 9.36d |
442.51 ± 7.98b |
520.74 ± 10.14a |
![[thin space (1/6-em)]](https://www.rsc.org/images/entities/char_2009.gif) |
Liver |
PC (nmol g−1 prot) |
2.78 ± 0.12c |
2.03 ± 0.25d |
3.54 ± 0.13b |
4.79 ± 0.25a |
DT (pg mg−1 prot) |
112.14 ± 11.12b |
92.32 ± 15.23b |
114.33 ± 6.65b |
134.31 ± 4.28a |
3-NT (nmol g−1 prot) |
43.68 ± 4.07b |
40.67 ± 1.76b |
55.12 ± 2.25a |
59.03 ± 3.67a |
AOPPs (pmol g−1 prot) |
301.25 ± 12.12b |
226.94 ± 8.69c |
311.86 ± 11.12b |
370.77 ± 13.36a |
3.7 Effects of diets containing milk powder at different degrees of oxidation on the relative expression of genes and proteins in the rat hippocampus
The expression levels of hippocampus-dependent learning-and memory-related genes and proteins examined in this study are shown in Fig. 7. In this study, we examined the differences among groups of rats in their expression of the mRNA of the antioxidant and inflammatory factors Nrf2, Prdx-1, and P38-α (Fig. 7A); the apoptosis factors CytC, caspase-3, and caspase-9 (Fig. 7B); learning and memory factors, including the NMDAR subunits Nr1, Nr2a, and Nr2b and the CaMKII subunits CaMK2A and CaMK2B (Fig. 7C); and the downstream genes CREB, BDNF and their receptors Trkb as well as PSD-95 (Fig. 7D). In addition, we also detected relative expression of Bcl-2 and Bax protein in rat's hippocampus (Fig. 7E–G).
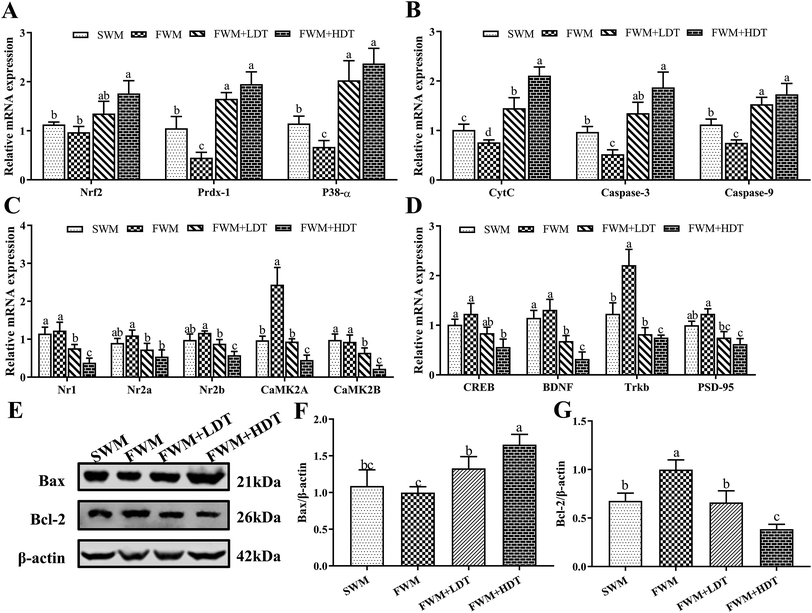 |
| Fig. 7 Effects of milk oxidation diet on the relative expression of hippocampus genes and proteins in SD rats. (A) The relative mRNA expression of Nrf2, Prdx-1, P38-α; (B) the relative mRNA expression of CytC, caspase-3, caspase-9; (C) the relative mRNA expression of Nr1, Nr2a, Nr2b, CaMK2A, CaMK2B; (D) the relative mRNA expression of CREB, BDNF, Trkb, PSD-95; (E) Western blot bands of Bax, Bcl-2, and β-actin; (F) relative expression of Bax protein; (G) relative expression of Bcl-2 protein. Nrf2, nuclear factor (erythroid-derived 2)-like 2; Prdx-1, peroxiredoxin-1; P38-α, mitogen-activated protein kinase 14; CytC, cytochrome complex; caspase-3, cysteine-requiring aspartate protease-3; caspase-9, cysteine-requiring aspartate protease-9; Nr1, N-methyl-D-aspartate receptor 1; Nr2a, N-methyl-D-aspartate receptor 2a; Nr2b, N-methyl-D-aspartate receptor 2b; CAMK2A, Ca2+/calmodulin-dependent protein kinase II alpha chain; CAMK2B, Ca2+/calmodulin-dependent protein kinase II beta chain; CREB, cAMP response element-binding protein; BDNF, brain-derived neurotrophic factor; PSD-95, postsynaptic density protein 95; Bcl-2, B-cell lymphoma 2; Bax, Bcl-2-associated X. SWM, 180 °C spray-dried whole milk group; FWM, freeze-dried whole milk group; FWM + LDT freeze-dried whole milk + low dityrosine group; FWM + HDT, freeze-dried whole milk + high dityrosine group. Data are expressed as the mean ± SEM (n = 10 rats per group). Means among bars without a common letter differ, P < 0.05. | |
Among the antioxidant and inflammatory factors investigated, the expression of Prdx-1 and P38-α in the SWM group was significantly higher than that in the FWM group (P < 0.05). The expression of Nrf2, Prdx-1, and P38-α in the FWM + HDT group was significantly higher than that in the FWM (P < 0.05) and SWM groups (P < 0.05). Among the apoptosis factors considered, the relative expression of CytC, caspase-3, and caspase-9 all significantly differed between the FWM and FWM + HDT groups and the FWM and SWM groups (P < 0.05). Among the learning and memory factors, we found that the relative expression of the NMDAR subunits Nr1, Nr2a, and Nr2b decreased with the degree of diets containing milk powder oxidation increased, and their expression in the FWM + HDT group was significantly lower than that in the FWM group (P < 0.05). The relative expression of CaMK2A in the SWM group was significantly lower than that in the FWM group (P < 0.05). The relative expression levels of CaMK2A and CaMK2B in the FWM + LDT, and FWM + HDT groups were all lower than those in the FWM group, and the differences in expression between these groups were also all significant (P < 0.05). The relative expression levels of CREB, BDNF, Trkb, and PSD-95 were decreased in the SWM group compared with those in the FWM group, wherein Trkb expression was significantly downregulated (P < 0.05). The relative expression levels of CREB, BDNF, Trkb, and PSD-95 in the SWM, FWM + LDT, and FWM + HDT groups were all lower than those in the FWM group, and their expression in the FWM + HDT group was significantly lower than that in the FWM group (P < 0.05). In terms of protein expression, the relative expression of Bax protein in the FWM + LDT group and the FWM + HDT group was significantly higher than that in the FWM group (P < 0.05). The relative expression levels of Bcl-2 protein were significantly down-regulated in the SWM group, the FWM + LDT group, and the FWM + HDT group compared with the FWM group (P < 0.05).
4 Discussion
In this study, the effects of different heating times, fat content, and inlet air temperatures on the degree of protein oxidation in processed milk were evaluated by measuring levels of PC, SH, DT, and MDA. We found that different processing conditions can cause different degrees of protein oxidation in milk. Among these, the difference in the DT content in milk powder between spray-drying and freeze-drying was the most remarkable. Based on this, we carried out an animal experiment in which rats were fed diets containing milk powder with different degrees of oxidation. A combined approach using traditional clinical medical techniques, an animal behavioral analysis system, Elisa biochemical analyses, qRT-PCR analysis of gene expression, Western blot analysis of protein expression and other methods was used to determine the oxidative stress damage and the expression of learning- and memory-related genes in rats fed different diets, and these changes were confirmed using the MWM test. The experimental results verified our previous assumptions that milk processing can lead to protein oxidation, and the intake of oxidized milk proteins can cause oxidative stress and damage to the body, eventually leading to spatial learning and memory impairment.
Different processing conditions can cause different degrees of protein oxidation in milk. We found that PC, DT, and MDA levels in milk increased, and SH content decreased, with longer boiling and microwave heating times, which indicated that the level of milk protein oxidation was increased by these processing methods. Processing of milk with a higher fat content also promoted the oxidation of more proteins in the milk. Our results also indicated that the increased temperature of the air inlet during spray-drying exacerbated not only the extent but also the rate of protein oxidation. Research by Lamberti et al.41 indicated that modifications to the protein profile occurred between raw and boiled milk following thermal treatments. Xiao et al.42 studied the changes in bovine whey protein after ordinary heating and microwave heating, and found that the levels of PC and DT in bovine whey protein significantly increased, and that of SH significantly decreased, after microwaves heating. Tang et al.43 observed a significant increase in the PC and DT content in oxidize casein and a decrease in protein digestibility in vitro, which was related to oxidize casein with MDA. These findings were consistent with our previous experimental results. During protein oxidation, amino acids with NH or NH2 in the side-chain are very sensitive to hydroxyl radicals,44 as for example tyrosine, can produce PC by hydroxyl radicals.45,46 High temperatures may increase the chances of inter-protein cross-linking occurring after the oxidative modification of proteins,47 and the groups exposed after the thermal denaturation of proteins can also become aggregated through forming disulfide bonds and various secondary bonds, which ultimately leads to a decrease in SH content.48 High temperatures will also result in the continued formation of more tyrosyl radicals and tyrosine residues, and increasing amounts of DT are produced as the interactions between these radicals or residues gradually increase.49 In food oxidize system, protein oxidation and lipid peroxidation are usually interdependent.50 MDA is an active carbonyl species that is produced during lipid peroxidation and has high reactivity,51 and can thus interact with the free amino groups of proteins and cause the carbonyl-ammonia cross-linking of proteins within or between polypeptide molecules, leading to the oxidative modification of proteins.52 In comparison, we found that the content of DT and MDA in WM and SM after microwave heating for 60 s exceeded the levels of these in milk boiling for 6 min, which shows that the oxidative damage done to milk proteins by microwave heating is greater than that caused by boiling. This may be related to the fact that during microwave heating electromagnetic waves can cause the vibration or rotation of molecules, which contributes to the breaking of chemical bonds and is responsible for the thermal effects of microwaves on polar molecules.42 Ultimately leads to cross-linking and denaturation of proteins. In the drying processes, the spray-drying of milk powder uses a mechanical force, such as pressure or centrifugal force, to disperse concentrated milk as mist-like droplets, which are then ejected through a sprayer into hot air to remove most of the water instantaneously and produce a solid powder. Proteins in milk may undergo cross-linking and denaturation after being subjected to the high temperatures and shear forces applied during spray-drying.53 However, the products obtained by freeze-drying are mostly cakes and lumps. This process could thus avoid the effects of high temperature on the oxidation of proteins and protect the original activity and quality of milk products.41 Therefore, the levels of PC and DT in milk powder produced by freeze-drying were significantly lower than those in milk processed through spray-drying.
Casein accounts for 80% of milk protein. Our previous studies found that casein oxidation leads to an increase in DT production.21 We found in milk processing tests in this study that different processing methods lead to differences in DT production, especially spray-drying and freeze-drying has the greatest impact on it. Previous studies also have shown that dietary DT can induce defects in novel object recognition in mice.27 Therefore, in animal experiments, we compared the effects of freeze-dried whole milk powder and spray-dried whole milk powder on spatial learning and memory in rats. Moreover, further added different doses of DT to the FWM diet to verify our hypothesis: the difference of DT content may play a significant role in the effects of freeze-dried whole milk powder and spray-dried whole milk powder on spatial learning and memory in mice. Our results support this hypothesis.
In this study, dietary milk protein oxidation was shown to impair spatial learning and memory in rats. The commonly used MWM test for hippocampus-dependent learning, and long-term spatial memory.54 The results of the MWM test showed that in the SWM, FWM + LDT, and FWM + HDT groups, the learning ability and spatial memory of rats were impaired relative to those of rats in the FWM group. The injury to learning and memory in the FWM + HDT group was the most serious. Notably, hippocampal NMDAR play essential roles in the induction of long-term potentiation (LTP), which is a synaptic process underlying learning and memory.55 When the expression of the Nr2b subunit is downregulated, this results in a decrease in Ca2+ influx, thereby reducing intracellular Ca2+ concentrations.56 Ca2+ can regulate Ca2+/calmodulin-dependent protein kinase II (CaMKII) family mRNA expression. Among these, CAMK2A and CAaMK2B are closely related to learning and memory.57 Research by Martina et al.58 indicates that the alpha chain encoded by CaMK2A is required for hippocampal LTP and spatial learning. Moreover, other research has shown that the expression of CREB gene, which is a downstream gene in the LTP pathway59 is regulated by CaMKII and NMDAR. This gene can also affect learning and memory through the cAMP-PKA-CREB-BDNF pathway by mediating the expression of BDNF and its receptor, Trkb.60 Moreover, BDNF is widely distributed in the central nervous system and plays a major role in the survival, differentiation, and growth of neurons during the development of the central nervous system.61 Collectively, the results showed that the induction of high oxidation levels due to the inclusion of oxidized milk powder in the diet can lead to spatial learning and memory/cognitive impairment, as well as decreased expression of the mRNA of genes associated with hippocampal learning and memory.
The possible mechanism by which milk protein oxidation causes the above harmful effects is through the induction of oxidative stress in the body. Excessive ROS can do harm to the body by attacking vital macromolecules and various cells, reducing T-AOC, and inducing various diseases.62 When the T-AOC is lowered, the cells of the body undergo a change in the direction of signal transduction in them, which causes the body to undergo early body reactions to stress and defense.63 The reduction in T-AOC also indicates that the body's antioxidant defense capacity is impaired.64 SOD is an active enzyme derived from living organisms, which is the first line of defense against ROS.60 GSH is an antioxidant that has a variety of biochemical protective effects on cells, which can remove ROS and other free radicals in the body and have cell detoxification effects.65 The reduction of SOD and GSH/GSSG activity in the body also indicates that oxidative stress has been initiated in the body, which has consumed the body's reducing power and caused a redox imbalance. Oxidized products are transferred to various target organs as they circulate within the body, where they can cause further harm. DT, 3-NT, and AOPPs have been regarded as novel biomarkers of oxidative stress in the body.66 With increasing levels of protein oxidation in the milk powder diets provided to the rats in this study, we saw more deposition of PC, DT, 3-NT, and AOPPs in the plasma, brain tissues, and liver. This indicated that the intake of diets containing milk powder at a high degree of oxidation led to oxidative stress and a state of redox imbalance in the body. However, in our study, PC, DT, and 3-NT were found to be deposited the most in the hippocampus. The hippocampus is mainly responsible for storing information and is a key component of human spatial learning and memory, which is also more prone to oxidative damage than other organs. The upregulation of the expression of Nrf2 and Prdx-1 mRNA in the hippocampus, suggesting that oxidative stress stimulates the hippocampus to initiate antioxidant defense mechanisms, while the upregulation of P38-α indicates that oxidative stress induced the release of inflammatory cytokines.67 Further, oxidative stress caused by ingestion of protein oxidation products may lead to apoptosis of nerve cells through the mitochondrial apoptotic pathway. Apoptosis is the main mechanism of neuronal loss, as occurs for example in Parkinson's disease and Alzheimer's disease.68 In this study, we also found significant changes in mRNA expression and protein expression of apoptotic factors in the hippocampus. This may have been a cause of the spatial learning and memory impairment observed in the rats after they were fed diets containing oxidized milk powder. The bcl-2 and the bax gene are currently known as a pair of most important regulatory genes that are functionally antagonistic in the process of apoptosis regulation. CytC is located outside the inner mitochondrial membrane and is released and secreted into the cytoplasm after damage to the mitochondria, which then initiates caspase-9 activation. Activated caspase-9 further activates the apoptosis effector protein caspase-3, which increases the expression levels of genes and proteins specifically involved in accelerating the apoptosis process.69,70 Collectively, these results showed that oxidative stress can cause an imbalanced redox state and neuronal apoptosis in the body after the ingestion of oxidized milk proteins, particularly DT, which ultimately leads to spatial learning and memory impairment.
5 Conclusions
In conclusion, prolonged heating time, increased fat content, and increased inlet air temperature all increased the oxidation of milk proteins during processing. Oxidative damage of milk proteins was greater by microwave heating than that caused by boiling. Feeding diets containing oxidized milk powder to SD rats caused increased oxidative stress in the brain, deposition of protein oxidation products, downregulation of learning and memory-associated genes, and upregulation of inflammation and apoptosis genes, which eventually led to problems with behavioral spatial learning and memory. Among the protein oxidation products considered, DT may play a particularly important role in these impacts. Therefore, it is recommended that the appropriate processing conditions are selected when preparing dairy products to control the oxidation level of the proteins in milk, improve people's eating habits, and avoid the damage to human health that could be caused by milk protein oxidation products.
Author contributions
Bowen Li was performing the experiments, collecting data, and preparing the manuscript draft; Yueting Ge and Yuhui Yang were involved in directing the research goals and editing of the manuscript; Ling Mo, Shuai Zhang and Jingbing Xu performed the processing experiments. Yuncong Xu performed the behavioral tests. Guowei Le and Yonghui Shi were responsible for experimental design, analyzing data, writing and editing the manuscript. All authors approved the submission of the final manuscript.
Ethical approval
All procedures performed in studies involving animals were in accordance with the ethical standards laid down in the National Guidelines for Experimental Animal Welfare (China) and in the 1964 Declaration of Helsinki and its later amendments. Animal use and protocol were reviewed and approved by the Jiangnan University Experimental Animal Management and Animal Welfare Ethics Committee.
Funding
This work received financial support from the 12th 5 Year Plan for Science and Technology Development (No. 2012BAD33B05), State Key Laboratory of Food Science and Technology of Jiangnan University in China (No. SKLF-ZZB-201609), the National Natural Science Foundation of China (No. 31571841, No. 31700301), Collaborative Innovation Center of Food Safety and Quality Control in Jiangsu Province.
Conflicts of interest
The authors declare that there are no conflicts of interest.
Abbreviations used
OECD | Organization for economic co-operation and development |
FAO | Food and agriculture organization of the united nation |
PC | Protein carbonyl |
SH | Sulfhydryl |
DT | Dityrosine |
MDA | Malondialdehyde |
3-NT | 3-Nitrotyrosine |
AOPPs | Advanced oxidative protein products |
ROS | Reactive oxygen species |
T-AOC | Total antioxidant capacity |
SOD | Superoxide dismutase |
GSH | Glutathione |
GSSG | Oxidized glutathione |
DNPH | 2,4-Dinitrophenylhydrazine |
DNTB | 5,5-Thio-2-nitrobenzoic acid |
WM | Whole milk |
SM | Semi-skimmed milk |
Sprague-Dawley rat | SD rat |
SWM | 180 °C spray-dried whole milk group |
FWM | Freeze-dried whole milk group |
FWM + LDT | Freeze-dried whole milk + low dityrosine group |
FWM + HDT | Freeze-dried whole milk + high dityrosine group |
MWM | Morris water maze |
TC | Total cholesterol |
TG | Triglyceride |
HDL-C | High-density lipoprotein cholesterol |
LDL-C | Low-density lipoprotein cholesterol |
qRT-PCR | Quantitative real-time PCR |
SEM | Standard error of the mean |
ANOVA | Analysis of variance |
Nrf2 | Nuclear factor (erythroid-derived 2)-like 2 |
Prdx-1 | Peroxiredoxin-1 |
P38-α | Mitogen-activated protein kinase 14 |
NMDAR | N-Methyl-D-aspartate receptor |
LTP | Long-term potentiation |
Nr1 | N-Methyl-D-aspartate receptor 1 |
Nr2a | N-Methyl-D-aspartate receptor 2a |
Nr2b | N-Methyl-D-aspartate receptor 2b |
CAMK II | Ca2+/calmodulin-dependent protein kinase II |
CAMK2A | Ca2+/calmodulin-dependent protein kinase II alpha chain |
CAMK2B | Ca2+/calmodulin-dependent protein kinase II beta chain |
CREB | cAMP response element-binding protein |
PKA | Protein kinase A |
BDNF | Brain-derived neurotrophic factor |
PSD-95 | Postsynaptic density protein 95 |
CytC | Cytochrome complex |
Caspase-9 | Cysteine-requiring aspartate protease-9 |
Caspase-3 | Cysteine-requiring aspartate protease-3 |
Bcl-2 | B-cell lymphoma 2 |
Bax | Bcl-2-associated X |
References
- O. T. U. Nations and A. Organization, OECD-FAO Agricultural Outlook 2018–2027, OECD Publishing, Paris, 2018 Search PubMed.
- E. R. Stadtman and R. L. Levine, Ann. N. Y. Acad. Sci., 2010, 899, 191–208 CrossRef.
- L. Xing, Y. Li and T. Li, PDA J. Pharm. Sci. Technol., 2019, 73, 260–275 CrossRef.
- C. B. Afonso and C. M. Spickett, Redox Biol., 2018, 6, 101066 CrossRef.
- J.-H. Kim, H.-J. Lee, D.-M. Shin, T.-K. Kim, Y.-B. Kim and Y.-S. Choi, Korean J. Food Sci. Anim. Resour., 2018, 38, 1101–1108 CrossRef.
- R. R. Panthi, A. L. Kelly, J. J. Sheehan, K. Bulbul, A. H. Vollmer and D. J. McMahon, J. Dairy Sci., 2019, 102, 177–189 CrossRef CAS.
- K. Kurpiewska, A. Biela, J. I. Loch, S. Świątek, B. Jachimska and K. Lewiński, Colloids Surf., B, 2018, 161, 387 CrossRef CAS.
- A. Aicardo, M. Mastrogiovanni, A. Cassina and R. Radi, Free Radical Res., 2018, 52, 159–170 CrossRef CAS.
- M. Zhao, M. Jiao, F. Zhou, L. Lin and W. Sun, Food Chem., 2018, 268, 315–323 CrossRef CAS.
- A. K. Verma, S. Singh and S. I. Rizvi, Gen. Comp. Endocrinol., 2019, S0016-6480(19)30081-4 Search PubMed.
- Z. Han, J. Zhang, J. Zheng, X. Li and J.-H. Shao, Food Chem., 2019, 280, 263–269 CrossRef CAS PubMed.
- F. Wang, W. Yang and X. Hu, Int. J. Mol. Sci., 2018, 20, 115 CrossRef.
- X. Wang, X. Zhao, D. Huang, X. Pan, Y. Qi, Y. Yang, H. Zhao and G. Cheng, Sci Rep, 2017, 7, 43020 CrossRef.
- S. H. M. Gorissen, J. J. R. Crombag, J. M. G. Senden, W. A. H. Waterval, J. Bierau, L. B. Verdijk and L. J. C. van Loon, Amino Acids, 2018, 50, 1685–1695 CrossRef CAS PubMed.
- E. Fuenteslemus, E. Silva, P. Barrias, A. Aspee, E. Escobar, L. G. Lorentzen, L. Carroll, F. Leinisch, M. J. Davies and C. Lópezalarcón, Free Radical Biol. Med., 2018, 124, 176–188 CrossRef CAS.
- Q. Liu, J. Wang and D. Bu, Food Sci., 2009, 30, 443–446 CAS.
- P. Zhang, X. Wu, M. Wang, Y. Wang, C. Gu, M. Wang, M. Guo, M. Wu, Q. Ge and H. Yu, Food Ferment. Ind., 2013, 39, 143–148 CAS.
- A. B. Gurung and A. Bhattacharjee, Int. J. Biol. Macromol., 2018, 107, 1956–1964 CrossRef CAS.
- I. Majkutewicz, E. Kurowska, M. Podlacha, D. Myślińska, B. Grembecka, J. Ruciński, K. Pierzynowska and D. Wrona, Brain Res., 2018, 1686, 19–33 CrossRef CAS.
- A.-L. Bulteau, N. P. Mena, F. Auchère, I. Lee, A. Prigent, C. S. Lobsiger, J.-M. Camadro and E. C. Hirsch, Free Radical Biol. Med., 2017, 108, 236–246 CrossRef CAS.
- Z. L. Li, Y. Shi, Y. Ding, Y. Ran and G. Le, Amino Acids, 2016, 49, 1–20 Search PubMed.
- A. H. Bryk, M. Konieczynska, P. Rostoff, E. Broniatowska, J. Hohendorff, M. T. Malecki and A. Undas, Thromb. Haemostasis, 2019, 119, 213–222 CrossRef PubMed.
- Y. Yang, H. Zhang, B. Yan, T. Zhang, Y. Gao, Y. Shi and G. Le, J. Agric. Food Chem., 2017, 65, 6957–6971 CrossRef CAS.
- Y. Y. Ding, X. R. Cheng, Z. Q. Li, S. J. Wu, Y. Yang, Y. H. Shi and G. W. Le, RSC Adv., 2017, 7, 26809–26826 RSC.
- Y. Y. Ding, Z. Q. Li, X. R. Cheng, Y. M. Ran, S. J. Wu, Y. Shi and G. Le, Amino Acids, 2017, 49, 1–14 CrossRef PubMed.
- Y. Y. Ding, X. Tang, X. R. Cheng, F. F. Wang, Z. Q. Li, S. J. Wu, X. R. Kou, Y. Shi and G. Le, RSC Adv., 2017, 7, 54610–54625 RSC.
- Y. Ran, B. Yan, Z. Li, Y. Ding, Y. Shi and G. Le, Physiol. Behav., 2016, 164, 292–299 CrossRef CAS PubMed.
- R. E. Ginn and V. S. Packard, J. Milk Food Technol., 1969, 32, 203–205 CrossRef CAS.
- R. B. Bradstreet, Anal. Chem., 1965, 26, 185–187 CrossRef.
- R. Sieber, P. Eberhard and P. U. Gallmann, Int. Dairy J., 1996, 6, 231–246 CrossRef.
- V. O. Alvarenga, G. T. P. Brancini, E. K. Silva, A. K. R. da Pia, F. B. Campagnollo, G. Ú. L. Braga, M. D. Hubinger and A. S. Sant'Ana, Int. J. Food Microbiol., 2018, 286, 80–89 CrossRef CAS.
- A. Stewart, A. Grandison, C. Fagan, A. Ryan, D. Festring and J. K. Parker, J. Dairy Sci., 2018, 101, 8822–8836 CrossRef CAS PubMed.
- P. Whongsiri, S. Phoyen and C. Boonla, Methods Mol. Biol., 2018, 1655, 109–117 CrossRef CAS.
- C. N. Oliver, B. W. Ahn, E. J. Moerman, S. Goldstein and E. R. Stadtman, J. Biol. Chem., 1987, 262, 5488 CAS.
- P. G. Reeves, F. H. Nielsen and G. C. Fahey, J. Nutr., 1993, 123, 1939–1951 CrossRef CAS PubMed.
- D. G. Tu, Y. L. Chang, C. H. Chou, Y. L. Lin, C. C. Chiang, Y. Y. Chang and Y. C. Chen, Food Funct., 2017, 9, 124–133 RSC.
- K. Bromley-Brits, Y. Deng and W. Song, J. Visualized Exp., 2011, 53, e2920 Search PubMed.
- H. Kobayashi, E. Gil-Guzman, A. M. Mahran, K. Rakesh, D. R. Nelson, A. J. Thomas and A. Agarwa, J. Androl., 2001, 22, 568–574 CAS.
- T. Luo, Y. Yang, Y. Xu, Q. Gao, G. Wu, Y. Jiang, J. Sun, Y. Shi and G. Le, Food Funct., 2019, 10, 2676–2690 RSC.
- Z. Sui, C. Qi, Y. Huang, S. Ma, X. Wang, G. Le and J. Sun, Food Funct., 2017, 8, 1460–1467 RSC.
- C. Lamberti, C. Baro, M. Giribaldi, L. Napolitano, L. Cavallarin and M. G. Giuffrida, J. Sci. Food Agric., 2018, 98, 2370–2377 CrossRef CAS.
- Y. Xiao, Y. T. Fang, H. U. Ye-Qing, F. Xie, X. L. Zhou, Y. M. Zhou and X. U. Di, Sci. Technol. Food Ind., 2014, 8, 105–108 Search PubMed.
- T. Xue, W. Qiuping, L. Guowei, W. Jiao, Y. Kaijian and S. Yonghui, J. Food Sci., 2012, 77, H16–H22 CrossRef.
- M. Estévez, Meat Sci., 2011, 89, 259–27957 CrossRef.
- V. Santé-Lhoutellier, T. Astruc, P. Marinova, E. Greve and P. Gatellier, J. Agric. Food Chem., 2008, 56, 1488–1494 CrossRef.
- V. Sante-Lhoutellier, L. Aubry and P. Gatellier, J. Agric. Food Chem., 2007, 55, 5343–5348 CrossRef CAS.
- S. Egp, G. Koutina, K. Almdal, T. Hassenkam, A. Mackie, R. Ipsen and B. Svensson, Food Funct., 2018, 9, 797–805 RSC.
- Q. Ruan, Y. Chen, X. Kong and Y. Hua, J. Agric. Food Chem., 2015, 63, 3524–3533 CrossRef CAS PubMed.
- H. Ostdal, M. J. Bjerrum, J. A. Pedersen and H. J. Andersen, J. Agric. Food Chem., 2000, 48, 3939–3944 CrossRef CAS.
- D. Xiang, L. Mei, S. Jing, H. Chen, X. Xu, Z. Jin and X. Liu, Food Hydrocolloids, 2017, S0268005X17306963 Search PubMed.
- W. U. Qiu-Ping, J. Wang, L. E. Guo-Wei, Y. J. Shui and Y. H. Shi, Acta Nutr. Sin., 2011, 33, 14–18 Search PubMed.
- W. Wei, H. Yufei and L. Qinlu, J. Food Sci. Technol., 2014, 51, 485–493 CrossRef.
- W. Shi, R. Bai, L. Lishuang, L. I. Jianlin, L. Shen, L. Zhang and T. Zheng, Food Sci., 2018, 9, 95–101 Search PubMed.
- E. N. Abd Al Haleem and W. M. El-Bakly, Chem.-Biol. Interact., 2019, 302, 108–116 CrossRef CAS.
- C. S. H. Law and L. S. Leung, eNeuro, 2018, 5, 0236-18 CrossRef.
- Q. Wu, Y. Zhao, X. Chen, M. Zhu and C. Miao, Can. J. Physiol. Pharmacol., 2018, 96, 241–248 CrossRef CAS.
- J. S. Brzozowski and K. A. Skelding, Pharmaceuticals, 2019, 2, E8 CrossRef.
- M. Proietti Onori, B. Koopal, D. B. Everman, J. D. Worthington, J. R. Jones, M. A. Ploeg, E. Mientjes, B. W. van Bon, T. Kleefstra, H. Schulman, S. A. Kushner, S. Küry, Y. Elgersma and G. M. van Woerden, Hum. Mutat., 2018, 39, 2008–2024 CrossRef CAS.
- B. Xue, J. Huang, B. Ma, B. Yang, D. Chang and J. Liu, Neuroscience, 2019, 404, 326–337 CrossRef CAS.
- H. Bai, L. Zhao, H. Liu, H. Guo, W. Guo, L. Zheng, X. Liu, X. Wu, J. Luo, X. Li, L. Gao, D. Feng and Y. Qu, Brain Res. Bull., 2018, 143, 145–154 CrossRef CAS.
- Y. Xu, Y. Yang, J. Sun, Y. Zhang, T. Luo, B. Li, Y. Jiang, Y. Shi and G. Le, Food Funct., 2019, 10, 1411–1425 RSC.
- J. Yang, Microvasc. Res., 2018, 123, 62–67 CrossRef.
- L. Wang, Y. Xie, W. Yang, Z. Yang, S. Jiang, C. Zhang and G. Zhang, Sci. Rep., 2019, 9, 1782 CrossRef.
- J. Zhang, J. Yu, Y. Chen, L. Liu, M. Xu, L. Sun, H. Luo, Y. Wang and G. Meng, Oxid. Med. Cell. Longevity, 2018, 9396089 Search PubMed.
- M.-Z. Huang, Y.-J. Yang, X.-W. Liu, Z. Qin and J.-Y. Li, Br. J. Pharmacol., 2019, 14592 Search PubMed.
- G. Gabai, E. De Luca, G. Miotto, G. Zin, A. Stefani, L. Da Dalt, A. Barberio and P. Celi, Antioxidants, 2019, 8, E21 CrossRef PubMed.
- J.-R. Wang, Y.-H. Luo, X.-J. Piao, Y. Zhang, Y.-C. Feng, J.-Q. Li, W.-T. Xu, Y. Zhang, T. Zhang, S.-N. Wang, H. Xue, W.-Z. Wang, L.-K. Cao and C.-H. Jin, Drug Dev. Res., 2019, 1–10 Search PubMed.
- S. Y. Cheon and K. J. Cho, J. Mol. Med., 2019, 97, 153–161 CrossRef CAS.
- Y. Zhao, J. Wang, J. Du, B. Li, X. Gou, J. Liu, L. Hou, H. Sang and B. Deng, Front. Cell. Neurosci., 2018, 12, 2–16 Search PubMed.
- J. H. Jeong, D. K. Kim, N.-S. Lee, Y.-G. Jeong, H. W. Kim, J.-S. Kim and S.-Y. Han, ASN Neuro, 2018, 10, 1–20 CrossRef.
Footnote |
† Electronic supplementary information (ESI) available. See DOI: 10.1039/c9ra03223a |
|
This journal is © The Royal Society of Chemistry 2019 |