DOI:
10.1039/C9RA02470K
(Paper)
RSC Adv., 2019,
9, 16718-16728
A nonlinear optical switch induced by an external electric field: inorganic alkaline–earth alkalide†
Received
2nd April 2019
, Accepted 14th May 2019
First published on 29th May 2019
Abstract
Exploring a new type of nonlinear optical switch molecule with excess electron character is extremely important for promoting the application of excess electron compounds in the nonlinear optical (NLO) field. Here, we report external electric field (EEF) induced second-order NLO switch molecules of inorganic alkaline–earth alkalides, M(NH3)6Na2 (M = Mg or Ca). The centrosymmetric structure of M(NH3)6Na2 is destroyed in the presence of an EEF, and then a long-range charge transfer process occurs. It has been found that excess electrons are gradually transferred from one Na atom to the other Na atom through the inorganic metal cluster M(NH3)6. Finally, the excess electrons are completely located on one of the two Na atoms. In particular, the electronic contribution of the static first hyperpolarizability (βe0) for M(NH3)6Na2 exhibits a large significant difference when the EEF is switched on. The βe0 value of M(NH3)6Na2 is 0 when EEF = 0, while the peak βe0 values are 5.95 × 106 (a.u.) for Mg(NH3)6Na2 (EEF = 58 × 10−4 (a.u.)) and 1.83 × 107 (a.u.) for Ca(NH3)6Na2 (EEF = 53 × 10−4 (a.u.)). This work demonstrates that the compounds M(NH3)6Na2 can serve as potential candidates for NLO switches.
1. Introduction
After the second harmonic generation (SHG)1 phenomenon was discovered in the 1960s and some inorganic crystals (i.e. LiNbO3 and KTiPO4)2 were widely used in commercial nonlinear optical (NLO) devices, NLO materials gained enormous attention and became an active research field, owing to their broad applications.3–9 Up to now, various types of strategies have been proposed to enhance (hyper)polarizability coefficients, i.e., designing organic molecules with a donor–acceptor architecture,10,11 spin-polarization molecules with singlet diradical character,12–14 importing diffuse excess electrons into a molecular system to form excess electron compounds, etc. Excess electron compounds have been proven to serve as potential candidates for NLO materials with excellent NLO responses.15–20
In recent years, studies on excess electron compounds have gained plentiful attention.21–29 Alkalides and electrides are typical representatives of excess electron compounds, where anion sites are occupied by anionic alkalis and trapped electrons, respectively.21–23,30–33 At room temperature, stable organic alkalides have been synthesized by Dye et al.33 Normally, an alkalide can be obtained by doping two alkali atoms into a suitable ligand, one of them acting as an anion and the other providing an excess of electrons. In addition, the first alkaline–earth based alkalide Ba2+(H5Azacryptand[2.2.2]−)Na−·2MeNH2 was synthesized by Dye and his co-workers.34 Some alkaline–earth alkalides with large NLO responses have been reported by Li et al., suggesting that an alkaline–earth with alkali atoms can also form an alkalide, where an alkaline–earth atom instead of an alkali atom provides the excess electrons.35
Stable magnesium and calcium ammines are well known to exhibit new and interesting properties. The inorganic metal cluster Ca(NH3)6 can be obtained by dissolving calcium in ammonia and its structure has been determined by experimental methods.36–40 However, inorganic magnesium ammine Mg(NH3)6 has not been obtained so far.41 Nevertheless, with the existence of Cl− counterions, Mg(NH3)6Cl2 can be obtained.42–46 Li's group35 used M(NH3)6 (M = Mg, Ca) to theoretically design a series of novel alkalide molecules with double alkali anions M(NH3)6Na2 and they found that those compounds had large static first hyperpolarizability (electronic contribution βe0) values ranging from 0 to 1.23 × 105 (a.u.). It was noticed that one of the isomers with centrosymmetry (with D3d point group) of M(NH3)6Na2 was the most stable, but its βe0 value was zero. The isomers with centrosymmetry M(NH3)6Na2 may be considered as potential NLO switches by breaking their symmetry with external stimulation, i.e. light irradiation,47 redox reaction,48 pH variation, ion recognition,49 external electric field (EEF), and so on.19,20,50
On the one hand, among the above-mentioned external stimulators, EEF plays an important role in chemistry. For instance, Nakano et al. have shown that singlet diradical molecules induced by EEF can produce giant static second hyperpolarizability.51,52 Bai et al. suggested that benzene has a large βe0 value after the centrosymmetric structure has been broken by switching on a large EEF.53 Sun et al. demonstrated that the βe0 value of superatom compounds can be greatly improved by 4790 times by imposing an EEF along the direction of charge transfer.54 Furthermore, EEF is also used to probe other properties, such as the Π stacking interaction,55 proton transfer,56 metal–ligand bonding, chemical reactions etc.57
On the other hand, NLO switches with a large difference in NLO properties in the presence of external stimulator triggering have attracted more and more attention in recent years,19,20,50 because of their excellent potential applications in signal processing, data storage and optical frequency converters etc.3–9 In the presence of EEF triggering, NLO switches of the organic electride molecule K(1)⋯calix[4]pyrrole⋯K(2) with βe0 values ranging from 0 (EEF = 0 (a.u.)) to 3.147 × 106 (a.u.) (EEF = 8 × 10−4 (a.u.)) and the all-metal electride molecules e− + M2+(Ni@Pb12)2−M2+ + e− (M = Be, Mg or Ca) with βe0 values ranging from 0 (EEF = 0 (a.u.)) to 2.2 × 106 (a.u.) (EEF = 30 × 10−4 (a.u.)), as well as Be6Li8 and Be6Li14, were reported by Li and Hou et al.19,20,50
It is worth noticing that the above-mentioned NLO molecular switches are all electride molecules.19,20,50 To the best of our knowledge, NLO switch molecules of an inorganic alkaline–earth alkalide have not been reported yet. Obviously, exploring new NLO switches with excess electrons is extremely necessary for promoting excess electron applications in the NLO field, which is the target of this work. With this motivation, we theoretically investigate the effects of EEF on the geometries, molecular orbitals and NLO properties of previously reported35 novel alkaline–earth alkalide molecules M(NH3)6Na2 (M = Mg, Ca) (see Fig. 1) in this work.
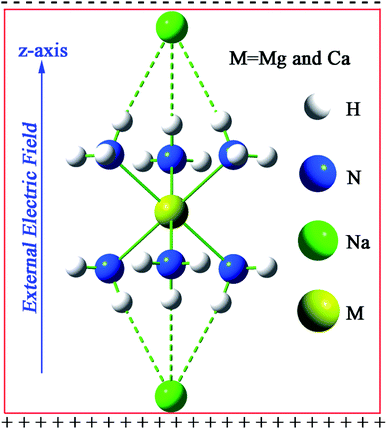 |
| Fig. 1 The optimized geometrical structure of M(NH3)6Na2 (M = Mg or Ca) at the CAM-B3LYP/6-311++G(2d,2p) level. | |
2. Computational details
All quantum chemistry calculations were executed by the Gaussian16 program package (revision B.01).58 The Coulomb-attenuated hybrid exchange–correlation functional CAM-B3LYP has been proposed59,60 and successfully applied to calculate (hyper)polarizabilities for charge transfer systems.20,35,61–65 What is more, this method has been confirmed to give a better performance for calculating hyperpolarizability in the presence of EEF.19 Furthermore, this functional can also provide molecular geometries close to experimental geometrical parameters.66 Therefore, geometrical optimization, frequency calculation, natural population analysis charge, interaction energy, electronic contributions of the polarizability and the static first hyperpolarizability were performed at the CAM-B3LYP/6-311++G(2d,2p) level.67 The oscillator strength of the crucial excited state (f0), the excited energy of the crucial excited state (ΔE), and the difference in transition dipole moment between the ground state and the crucial excited state (Δμ) were calculated at the TD-CAM-B3LYP/6-311++G(2d,2p) level.68 It should be mentioned that f0 and Δμ were obtained with Gaussian16, while Δμ was obtained with the free and open source Multiwfn program package (revision 3.6).69
The interaction energies (Eint) are defined by eqn (1):
|
Eint = EM(NH3)6Na2 − E(NH3)6Na2 − ENa2
| (1) |
where
EM(NH3)6Na2,
E(NH3)6Na2 and
ENa2 correspond to the energies of M(NH
3)
6Na
2, (NH
3)
6Na
2 and Na
2.
The electronic contributions of the polarizability αe and the static first hyperpolarizability βe0 are defined by eqn (2)–(4):
|
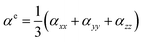 | (2) |
|
βe0 = (βx2 + βy2 + βz2)1/2
| (3) |
where
|
βi = (βiii + βijj + βikk), i, j, k = x, y, z
| (4) |
3. Results and discussion
3.1 EEF effects on geometries
The equilibrium geometries of M(NH3)6Na2 (M = Mg or Ca) were obtained at the CAM-B3LYP/6-311++G(2d,2p) level and are depicted in Fig. 1. It should be noted that the direction of the imposed EEF is along the z-axis with a magnitude of 0 to 68 × 10−4 (a.u.). If the strength of the imposed EEF is larger than 63 × 10−4 (a.u.), the geometry of Mg(NH3)6Na2 will be destroyed, whereas, if EEF is larger than 68 × 10−4 (a.u.), the geometry of Ca(NH3)6Na2 will be destroyed. Given that Ca(NH3)6Na2 (−37.43 kcal mol−1) shows a larger interaction compared with Mg(NH3)6Na2 (−35.02 kcal) in the absence of EEF, the largest EEF thresholds are different. The point group of M(NH3)6Na2 will be changed from D3d (EEF = 0) to C3V (EEF ≠ 0). For a good visualization of the variable relationships between geometrical parameters and EEF, they are plotted in Fig. 2, and the related geometrical parameters have been collected in Tables S1 and S2.†
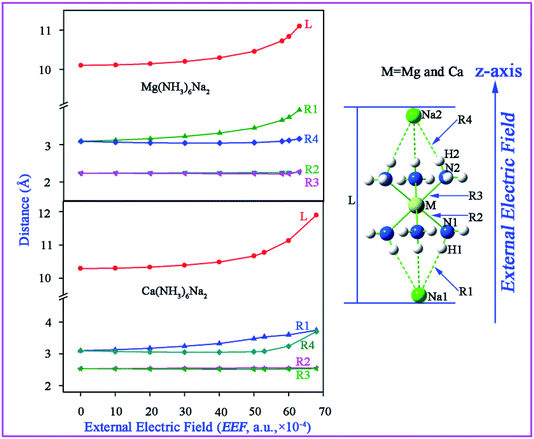 |
| Fig. 2 The geometrical parameters (Å) with different magnitudes of the external electric field (EEF, a.u., 10−4) for M(NH3)6Na2 (M = Mg or Ca) at the CAM-B3LYP/6-311++G(2d,2p) level. | |
From Fig. 2, for both Mg(NH3)6Na2 and Ca(NH3)6Na2, one can see that the distance R1(Na1–H1) is equal to R4(Na2–H2), and R2(N1–Mg) is equal to R3(N2–Mg) when EEF = 0. While, if EEF is not equal to 0, R1 ≠ R4, R2 ≈ R3 and L(Na1–Na2) is elongated. It should be noticed that the variation in L, R1, and R4 is small when EEF is less than 40 × 10−4 (a.u.). But, L, R1 and R4 are all drastically elongated when EEF is greater than 50 × 10−4 (a.u.). In terms of an electro-optical device, it is expected that the geometry should retain its integrity. Therefore, EEF switches on the range from 0 to 40 × 10−4 (a.u.) for Mg(NH3)6Na2 and Ca(NH3)6Na2, which is conducive to the development of a reversible switch.
3.2 EEF effects on the highest occupied molecular orbitals
The highest occupied molecular orbitals (HOMOs) (see Fig. 3) of M(NH3)6Na2 (M = Mg or Ca) clearly display that the electron cloud is mainly distributed on the two Na atoms or one of two Na atoms, which suggests that M(NH3)6Na2 compounds have some excess electrons. In addition, the charges by the natural population analysis on Na atoms are negative (−0.695 to −0.776|e| for M = Mg, −0.787 to −1.055|e| for M = Ca, see Table S3†), which further suggests that M(NH3)6Na2 compounds exhibit an alkalide character. From Fig. 3, one can see that when EEF = 0, the two excess electrons are distributed on the two Na atoms and exhibit centrosymmetry. The excess electrons are gradually transferred from one Na to the other Na atom with an increase in the EEF strength, resulting in the symmetry of the HOMOs for M(NH3)6Na2 being broken. When EEF > 20 × 10−4 (a.u.), the excess electrons located on one of the two Na atoms (the electric cloud of the large part is excess electrons of Na, the electronic cloud of the small part is the lone pair of N atoms of the NH3 clusters), which demonstrates that a long-range charge transfer process is occurring through the inorganic metal cluster M(NH3)6 and this process may bring about a large NLO response.
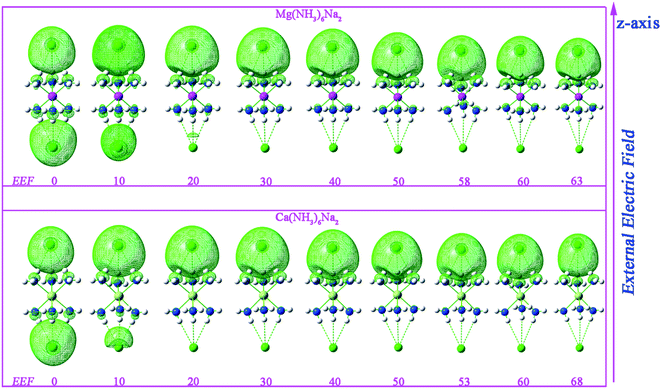 |
| Fig. 3 The highest occupied molecular orbital (HOMO) with different magnitudes of external electric field (EEF, a.u., 10−4) for M(NH3)6Na2 (M = Mg or Ca) at the CAM-B3LYP/6-311++G(2d,2p) level. | |
3.3 EEF effects on nonlinear optical properties
The βe0 values of M(NH3)6Na2 (M = Mg or Ca) with and without EEF were calculated. To better visualize the results, the relationships between the βe0 values of M(NH3)6Na2 and EEF have been plotted in Fig. 4 and 5.
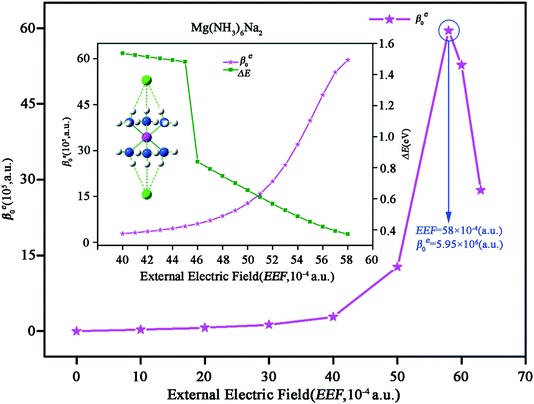 |
| Fig. 4 The electronic contribution of the static first hyperpolarizability (βe0, a.u.) and the excited energy with different magnitudes of external electric field (EEF, a.u., 10−4) for Mg(NH3)6Na2 at the CAM-B3LYP/6-311++G(2d,2p) level. | |
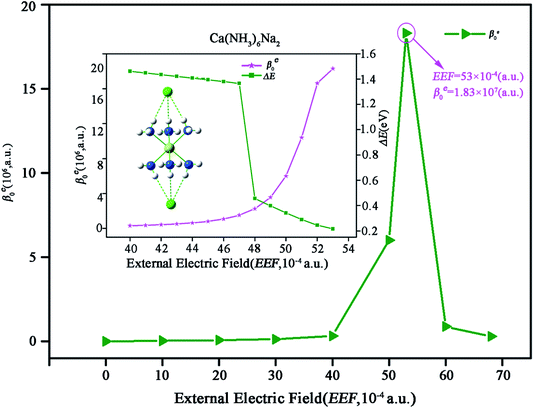 |
| Fig. 5 The electronic contribution of the static first hyperpolarizability (βe0, a.u.) and the excited energy with different magnitudes of external electric field (EEF, a.u., 10−4) for Ca(NH3)6Na2 at the CAM-B3LYP/6-311++G(2d,2p) level. | |
As shown in Fig. 4, the βe0 of Mg(NH3)6Na2 first increases to the peak value of 5.95 × 106 (a.u.) for EEF = 58 × 10−4 (a.u.), and then rapidly reduces to 2.79 × 106 (a.u.) for EEF = 63 × 10−4 (a.u.), indicating that the βe0 value exhibits a large difference without and with EEF. It should be noticed that the βe0 value of Mg(NH3)6Na2 slowly increases when EEF is less than 40 × 10−4 (a.u.), but βe0 greatly increases when the EEF is between 40 × 10−4 and 58 × 10−4 (a.u.). Fig. 4 clearly displays that the βe0 value sharply increases from 2.84 × 105 (a.u.) to 5.95 × 106 (a.u.) when EEF is in the range of 40 × 10−4 to 58 × 10−4 (a.u.). Therefore, we focus our attention on variation in βe0 over this range of EEF. The βe0 value of Mg(NH3)6Na2 for this range of EEF was recalculated (EEF with a step size 1 × 10−4 (a.u.)) and the results are plotted in Fig. 4 (inset). The βe0 of Mg(NH3)6Na2 slowly increases when EEF is in the range between 40 × 10−4 and 45 × 10−4 (a.u.), and βe0 increases significantly when EEF is within the range from 46 × 10−4 to 58 × 10−4 (a.u.).
To better understand the change in βe0 with the EEF switched on, a simplified two-level calculation is performed,70
|
 | (5) |
where Δ
μ,
f0, and Δ
E denote the difference in transition dipole moment between ground state and crucial excited state, the oscillator strength of the crucial excited state and the excited energy of the crucial excited state. From
eqn (5), one can see that
βe0 depends on three quantities: Δ
μ,
f0 and Δ
E. Obviously, Δ
E is the decisive factor for
βe0, since
βe0 is proportional to the inverse of its cube. While, sometimes the other two factors also cannot be neglected. Thus, we provide criteria for choosing the crucial excited state using values of these three quantities. The related Δ
μ,
f0, and Δ
E values of the crucial excited state are collected in Table S4,
† where one can clearly see a better inverse relationship between
βe0 and Δ
E, which is plotted in
Fig. 4 (inset). To be specific, Δ
E exhibits a decreasing trend with increasing EEF, which demonstrates why
βe0 increases with increasing EEF. In addition,
Fig. 4 also displays that Mg(NH
3)
6Na
2 holds high excited energies (1.4816 eV to 1.5356 eV) when EEF is between 40 × 10
−4 and 45 × 10
−4 (a.u.), but low excited energies (0.3722 eV to 0.8384 eV) when EEF is between 46 × 10
−4 and 58 × 10
−4 (a.u.). The reason for this is that the crucial excited states are located at high excited state S6 for EEF ranging from 40 × 10
−4 to 45 × 10
−4 (a.u.) and low excited state S1 for EEF ranging from 40 × 10
−4 to 45 × 10
−4 (a.u.). This explains why
βe0 slowly increases at first, and then quickly increases when EEF is within the range of 40 × 10
−4 to 58 × 10
−4 (a.u.). In addition, one can see from
Fig. 4 that the
βe0 values rapidly decrease after presenting a maximum, which is due to Δ
E increasing with increasing EEF; the excited energy increased to 1.6794 eV.
For the case of Ca(NH3)6Na2, from Fig. 5 one can clearly see that βe0 value of Ca(NH3)6Na2 gradually increases to 3.11 × 105 (a.u.) when EEF is less than 40 × 10−4 (a.u.), and then dramatically increases to the largest βe0 value of 1.83 × 107 (a.u.) for EEF = 53 × 10−4 (a.u.), rapidly decreasing to 2.99 × 105 (a.u.) for EEF = 68 × 10−4 (a.u.). Here, we mainly focus on discussing the variation in βe0 with EEF ranging from 40 × 10−4 to 53 × 10−4 (a.u.). The corresponding βe0 (EEF with a step size of 1 × 10−4 (a.u.)) was calculated and is given in Fig. 5 (inset).
How can we understand the variation in βe0 with changing EEF? It is likely that we will find some clues from eqn (4). Fig. 5 clearly shows the relationship between βe0 and ΔE. When EEF continuously increases, the βe0 value of Ca(NH3)6Na2 increases with decreasing ΔE (1.4617 to 0.2155 eV). Furthermore, Fig. 5 also illustrates that ΔE dramatically decreases. The reason for this is that the crucial excited state goes from high excited state S6 for EEF ranging from 40 × 10−4 to 47 × 10−4 (a.u.) to low excited state S1 for EEF within in the range of 48 × 10−4 to 53 × 10−4 (a.u.), which explains why βe0 increases slowly at first and then rapidly increases in this EEF range (40 × 10−4 to 53 × 10−4 (a.u.)). Therefore, ΔE is a decisive factor for the variation of βe0 with changing EEF. Similarly, the βe0 values dramatically decrease after exhibiting a peak value, owing to ΔE increasing with increasing EEF, which increased to 1.7838 eV.
In the above discussions, we have obtained a qualitative explanation for the relationship between βezzz and the external electric field according to the simplified two-level equation. In order to obtain a quantitative explanation for this, hyperpolarizability density analysis is a good choice.71,72 Hyperpolarizability density ρ is defined by the electronic density of a spatial point r in the presence of an external field F, namely, by the Taylor expansion of energy with respect to an external field:
|
 | (6) |
From eqn (6), βezzz can be obtained by the following formula:
|
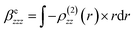 | (7) |
where
|
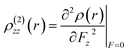 | (8) |
The first hyperpolarizability density maps (see Fig. 6) are plotted with the free and open source Multiwfn program.69 One can see from Fig. 6 that the electronic contribution on the first hyperpolarizability mainly originates from the upper Na atom under high EEF. Furthermore, the values of βezzz increase with increasing EEF, which explains why the total βe0 values increase with increasing EEF.
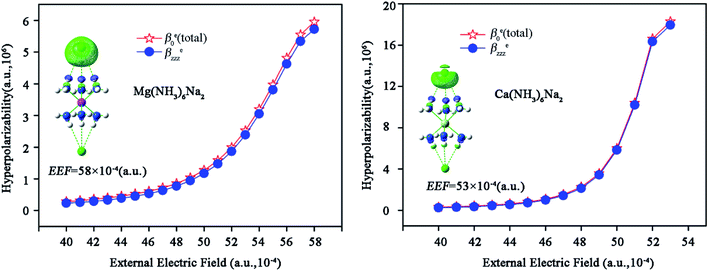 |
| Fig. 6 Diagrams of hyperpolarizability density −ρ(2)zz (EEF = 58 × 10−4 for Mg(NH3)6Na2 and EEF = 53 × 10−4 for Ca(NH3)6Na2) and the relationships between βezzz and the external electric field. | |
For a good illustration of the performance of M(NH3)6Na2 (M = Mg or Ca) in a nonlinear optical switch, we further compared the electronic contribution of the first hyperpolarizability value between the current work and previous reports for a nonlinear optical switch. It was found that the inorganic alkaline–earth alkalides M(NH3)6Na2 (M = Mg or Ca), with βe0 values of 0 to 5.95 × 106 (a.u.) and 0 to 1.83 × 107 (a.u.) for Mg(NH3)6Na2 and Ca(NH3)6Na2, respectively, show a better performance than organic electride K(1)⋯calix[4]pyrrole⋯K(2) with a βe0 of 0 to 3.15 × 106(a.u.),19 all-metal electride e− + M2+(Ni@Pb12)2−M2+ + e− (M = Be, Mg or Ca) with βe0 ranging from 0 to 2.20 × 106 (a.u.)20 or all-metal electride Be6Li8 with the largest βe0 value of 5.54 × 104 (a.u.) and Be6Li14 with the largest βe0 value 5.0 × 106 (a.u.).50 Furthermore, M(NH3)6Na2 also showed a better NLO performance than the organic compound benzene with the largest βe0 value of 3.9 × 105 (a.u.) in the presence of EEF.53
Furthermore, some NLO switch molecules have been synthesized in the presence of external stimulator triggering: e.g. an acido-triggered second-order NLO switch photochromic cyclometallated platinum(II) complex,73 a temperature-induced symmetry-breaking phase transition NLO switch74 and so forth. Experimental investigations further demonstrate the possibility of the synthesis of a stimulator introduced NLO switch.
In terms of applications of nonlinear optical switches, stability is also an important issue. Thus, to characterize the stability of compounds with a high external electric field, we further calculated the interaction energy between M(NH3)6 (M = Mg or Ca) and Na atoms in the range of a high external electric field. The calculated results showed that the ranges of interaction energy for Mg(NH3)6Na2 and Ca(NH3)6Na2 are −27.70 to −35.19 and −29.17 to −36.93 kcal mol−1 (see Fig. 7), respectively, which demonstrates that M(NH3)6Na2 (M = Mg or Ca) compounds are stable over the range of a high external electric field. Mg(NH3)6Na2 still has a large interaction energy of −7.71 kcal mol−1 under a working external electric field, it should be revised that Mg(NH3)6Na2 still has a large interaction energy −27.71 kcal mol−1 under a working external electric field. Thus, this compound also is relatively stable under a working external electric field with the largest value of βe0.
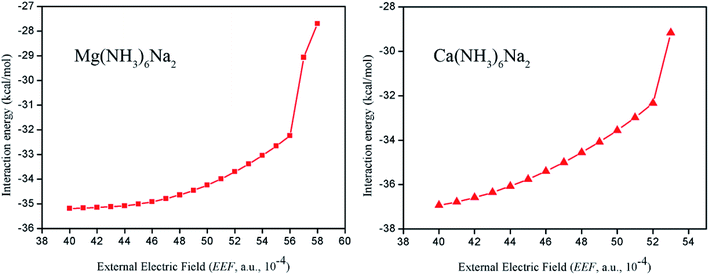 |
| Fig. 7 The interaction energy between M(NH3)6 (M = Mg or Ca) and Na atoms under different external electric fields (EEF, a.u., 10−4) for Ca(NH3)6Na2 at the CAM-B3LYP/6-311++G(2d,2p) level. | |
To better understand the frequency dependence behavior of M(NH3)6Na2 (M = Mg or Ca), we further calculated the electronic contribution of the polarizability (αe0) dispersion curve of M(NH3)6Na2 (EEF = 58 × 10−4 (a.u.) for M = Mg, EEF = 53 × 10−4 (a.u.) for M = Ca) with an optical frequency (ω) ranging from 0 to 0.5 eV, and the relationships between αe0 and ω are plotted in Fig. 8. This clearly shows that there are pole points with their positions close to 0.3722 eV and 0.2155 eV for Mg(NH3)6Na2 and Ca(NH3)6Na2, respectively. When ω is calculated to be close to the pole points, it will generate a very large electronic contribution of dynamic (hyper)polarizabilities.
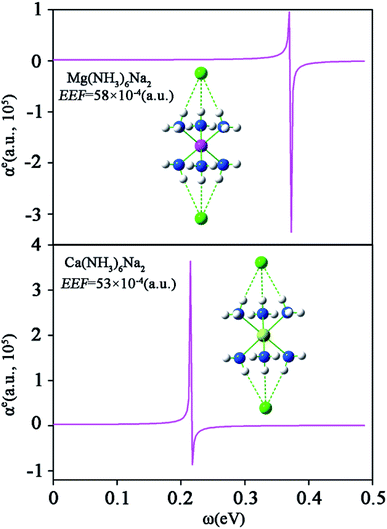 |
| Fig. 8 The electronic contribution of the polarizability dispersion curve for M(NH3)6Na2 (M = Mg or Ca) at the CAM-B3LYP/6-311++G(2d,2p) level. | |
In addition, the nuclear relaxation contribution of the static first hyperpolarizability βnr0 also plays a key role in NLO properties.75,76 Thus, we further calculated the βnr0 of M(NH3)6Na2 by using the field induced coordinates (FICs) method which was proposed by Luis's group, and the results are given in Table 1. For more detailed information on FICs, readers are recommended to read ref. 77–79. One can clearly see that βnr0 exhibits a large contribution with 3.84 × 106 (a.u.) and 4.01 × 106 (a.u.) for Mg(NH3)6Na2 and Ca(NH3)6Na2, respectively, which indicates that the nuclear relaxation contribution to the NLO properties of M(NH3)6Na2 is also a significant component.
Table 1 The electron (βe0, a.u.) and nuclear relaxation (βnr0, a.u.) contributions of the static first hyperpolarizability of M(NH3)6Na2 at the CAM-B3LYP/6-311++G(2d,2p) level
EEF (a.u., ×10−4) |
Mg(NH3)6Na2 |
EEF (a.u., ×10−4) |
Ca(NH3)6Na2 |
βe0 |
βnr0 |
βe0 |
βnr0 |
58 |
5.95 × 106 |
3.84 × 106 |
53 |
1.83 × 107 |
4.01 × 106 |
4. Conclusions
In this work, we have designed NLO switch molecules, Mg(NH3)6Na2 and Ca(NH3)6Na2, by a theoretical study. Our computational results demonstrate that the inorganic alkaline–earth alkalide M(NH3)6Na2 (M = Mg or Ca) can serve as a potential candidate for a NLO switch. The results highlight the following points:
(1) The centrosymmetric structure of M(NH3)6Na2 is destroyed in the presence of an EEF, and then a long-range transfer process occurs.
(2) The electronic contribution of the static first hyperpolarizability (βe0) is very sensitive to the EEF. The βe0 exhibits a significant difference when the EEF is switched on. The peak βe0 value is 5.95 × 106 (a.u.) for Mg(NH3)6Na2 and 83 × 107 (a.u.) for Ca(NH3)6Na2.
(3) The electronic contribution of the polarizability curve for M(NH3)6Na2 (EEF = 58 × 10−4 (a.u.) for M = Mg, EEF = 53 × 10−4 (a.u.) for M = Ca) with optical frequency ranging from 0 to 0.5 eV was obtained. The pole points are 0.3722 eV and 0.2155 eV for M = Mg and M = Ca, respectively.
(4) The nuclear relaxation contribution of the static first hyperpolarizability plays a key role in the NLO properties of M(NH3)6Na2.
In general, we hope that this work can provide a theoretical reference for designing NLO switches and motivate experimental chemists to synthesize them in the near future.
Conflicts of interest
There are no conflicts to declare.
Acknowledgements
The authors are grateful for financial support from the National Key R&D Program of China (Grant No. 2017YFB0203403). This work was also supported by the National Natural Science Foundation of China (Grant No. 21673085 and 21773075) and the Guangdong-Hong Kong Technology Cooperation Funding Scheme (Grant No. 2017A050506048). All authors thank Prof. Josep M. Luis of University of Girona for providing Field Induced Coordinates (FICs) code and thank Dr Hui-Min He of Jilin University for technology support with FICs.
References
- P. A. Franken, A. E. Hill, C. W. Peters and G. Weinreich, Phys. Rev. Lett., 1961, 7, 118–119 CrossRef.
- D. R. Kanis, M. A. Ratner and T. J. Marks, Design and construction of molecular assemblies with large second-order optical nonlinearities. Quantum chemical aspects, Chem. Rev., 1994, 94, 195–242 CrossRef CAS.
- F. Meyers, S. R. Marder, B. M. Pierce and J. L. Brédas, Electric Field Modulated Nonlinear Optical Properties of Donor-Acceptor Polyenes: Sum-Over-States Investigation of the Relationship between Molecular Polarizabilities (.alpha., .beta., and .gamma.) and Bond Length Alternation, J. Am. Chem. Soc., 1994, 116, 10703–10714 CrossRef CAS.
- M. Ja Lee, M. Piao, M. Y. Jeong, S. Hae Lee, K. Min Kang, S. J. Jeon, T. Gun Lim and B. Rae Cho, Novel azo octupoles with large first hyperpolarizabilities, J. Mater. Chem., 2003, 13, 1030–1037 RSC.
- O. Ostroverkhova and W. E. Moerner, Organic photorefractives: mechanisms, materials, and applications, Chem. Rev., 2004, 104, 3267–3314 CrossRef CAS PubMed.
- S. Muhammad, H. L. Xu, R. L. Zhong, Z. M. Su, A. G. Al-Sehemic and A. Irfanc, Quantum chemical design of nonlinear optical materials by sp 2-hybridized carbon nanomaterials: issues and opportunities, J. Mater. Chem. C, 2013, 1, 5439–5449 RSC.
- L. W. Chen, G. T. Yu, W. Chen, C. Y. Tu, X. G. Zhao and X. R. Huang, Constructing a mixed π-conjugated bridge to effectively enhance the nonlinear optical response in the Möbius cyclacene-based systems, Phys. Chem. Chem. Phys., 2014, 16, 10933–10942 RSC.
- L. Wang, J. T. Ye, H. Chen, Z. Z. Chen, Y. Q. Qiu and H. M. Xie, A structure–property interplay between the width and height of cages and the static third order nonlinear optical responses for fullerenes: applying gamma density analysis, Phys. Chem. Chem. Phys., 2017, 19, 2322–2331 RSC.
- M. Rajeshirke, M. C. Sreenath, S. Chitrambalam, I. H. Joe and N. Sekar, Enhancement of NLO Properties in OBO Fluorophores Derived from Carbazole–Coumarin Chalcones Containing Carboxylic Acid at the N-Alykl Terminal End, J. Phys. Chem. C, 2018, 122, 14313–14325 CrossRef CAS.
- J. O. Morley, Calculated hyperpolarisabilities of polythiophenes, polyfurans and polypyrroles, J. Chem. Soc., Faraday Trans., 1991, 87, 3009–3013 RSC.
- N. N. Ma, S. L. Sun, C. G. Liu, X. X. Sun and Y. Q. Qiu, Quantum Chemical Study of Redox-Switchable Second-Order Nonlinear Optical Responses of D− π–A System BNbpy and Metal Pt (II) Chelate Complex, J. Phys. Chem. A, 2011, 115, 13564–13572 CrossRef CAS PubMed.
- M. Nakano, R. Kishi, T. Nitta, T. Kubo, K. Nakasuji, K. K. K. Ohta, B. Champagne, E. Botek and K. Yamaguchi, Second hyperpolarizability (γ) of singlet diradical system: dependence of γ on the diradical character, J. Phys. Chem. A, 2005, 109, 885–891 CrossRef CAS PubMed.
- M. Nakano, R. Kishi, S. Ohta, H. Takahashi, T. Kubo, K. Kamada, K. Ohta, E. Botek and B. Champagne, Relationship between third-order nonlinear optical properties and magnetic interactions in open-shell systems: a new paradigm for nonlinear optics, Phys. Rev. Lett., 2007, 99, 033001 CrossRef PubMed.
- M. Nakano and B. Champagne, Theoretical design of open-shell singlet molecular systems for nonlinear optics, J. Phys. Chem. Lett., 2015, 6, 3236–3256 CrossRef CAS.
- W. Chen, Z. R. Li, D. Wu, Y. Li, C. C. Sun, F. L. Gu and Y. Aoki, Nonlinear Optical Properties of Alkalides Li+(calix[4]pyrrole)M- (M = Li, Na, and K): Alkali Anion Atomic Number Dependence, J. Am. Chem. Soc., 2006, 128, 1072–1073 CrossRef CAS PubMed.
- R. L. Zhong, H. L. Xu, Z. R. Li and Z. M. Su, Role of excess electrons in nonlinear optical response, J. Phys. Chem. Lett., 2015, 6, 612–619 CrossRef CAS PubMed.
- W. M. Sun, L. T. Fan, Y. Li, J. Y. Liu, D. Wu and Z. R. Li, On the potential application of superalkali clusters in designing novel alkalides with large nonlinear optical properties, Inorg. Chem., 2014, 53, 6170–6178 CrossRef CAS PubMed.
- B. Li, C. Xu, X. Xu, C. Y. Zhu and F. L. Gu, Remarkable nonlinear optical response of excess electron compounds: theoretically designed alkali-doped aziridine M–(C2NH5)n, Phys. Chem. Chem. Phys., 2017, 19, 23951–23959 RSC.
- J. J. Wang, Z. J. Zhou, H. M. He, D. Wu, Y. Li, Z. R. Li and H. X. Zhang, An External Electric Field Manipulated Second-Order Nonlinear Optical Switch of an Electride Molecule: A Long-Range Electron Transfer Forms a Lone Excess Electron Pair and Quenches Singlet Diradical, J. Phys. Chem. C, 2016, 120, 13656–13666 CrossRef CAS.
- H. M. He, Y. Li, H. Yang, D. Yu, S. Y. Li, D. Wu, J. H. Hou, R. L. Zhong, Z. J. Zhou, F. L. Gu, J. M. Luis and Z. R. Li, Efficient External Electric Field Manipulated Nonlinear Optical Switches of All-Metal Electride Molecules with Infrared Transparency: Nonbonding Electron Transfer Forms an Excess Electron Lone Pair, J. Phys. Chem. C, 2017, 121, 958–968 CrossRef CAS.
- J. L. Dye, Electrides: from 1D Heisenberg chains to 2D pseudo-metals, Inorg. Chem., 1997, 36, 3816–3826 CrossRef CAS.
- A. S. Ichimura, J. L. Dye, M. A. Camblor and L. A. Villaescusa, Toward inorganic electrides, J. Am. Chem. Soc., 2002, 124, 1170–1171 CrossRef CAS PubMed.
- S. Matsuishi, Y. Toda, M. Miyakawa, K. Hayashi, T. Kamiya, M. Hirano, I. Tanaka and H. Hosono, High-density electron anions in a nanoporous single crystal:[Ca24Al28O64]4+(4e-), Science, 2003, 301, 626–629 CrossRef CAS PubMed.
- M. J. Wagner and J. L. Dye in Molecular Recognition: Receptors for Cationic Guests, ed. G. W. Gokel, Pergamon, Oxford, UK, 1996, vol. 1, pp. 477–510 Search PubMed.
- J. L. Dye, Anionic electrons in electrides, Nature, 1993, 365, 10–11 CrossRef.
- P. P. Edwards, P. A. Anderson and J. M. Thomas, Dissolved alkali metals in zeolites, Acc. Chem. Res., 1996, 29, 23–29 CrossRef CAS PubMed.
- J. L. Dye, M. J. Wagner, G. Overney, R. H. Huang, T. F. Nagy and D. Tománek, Cavities and channels in electrides, J. Am. Chem. Soc., 1996, 118, 7329–7336 CrossRef CAS.
- V. I. Srdanov, G. D. Stucky, E. Lippmaa and G. Engelhardt, Evidence for an Antiferromagnetic Transition in a Zeolite-Supported Cubic Lattice of F Centers, Phys. Rev. Lett., 1998, 80, 2449–2452 CrossRef CAS.
- J. L. Dye, Electrons as anions, Science, 2003, 301, 607–608 CrossRef CAS PubMed.
- S. B. Dawes, D. L. Ward, R. H. Huang and J. L. Dye, First electride crystal structure, J. Am. Chem. Soc., 1986, 108, 3534–3535 CrossRef CAS.
- F. J. Tehan, B. L. Barnett and J. L. Dye, Alkali anions. Preparation and crystal structure of a compound which contains the cryptated sodium cation and the sodium anion, J. Am. Chem. Soc., 1974, 96, 7203–7208 CrossRef CAS.
- J. L. Dye, J. M. Ceraso, M. L. Tak, B. L. Barnett and F. J. Tehan, Crystalline salt of the sodium anion (Na-), J. Am. Chem. Soc., 1974, 96, 608–609 CrossRef CAS.
- J. Kim, A. S. Ichimura, R. H. Huang, M. Redko, R. C. Phillips, J. E. Jackson and J. L. Dye, Crystalline Salts of Na- and K- (Alkalides) that Are Stable at Room Temperature, J. Am. Chem. Soc., 1999, 121, 10666–10667 CrossRef CAS.
- M. Y. Redko, R. H. Huang, J. E. Jackson, J. F. Harrison and J. L. Dye, Barium Azacryptand Sodide, the First Alkalide with an Alkaline Earth Cation, Also Contains a Novel Dimer, (Na2)2-, J. Am. Chem. Soc., 2003, 125, 2259–2263 CrossRef CAS PubMed.
- W. M. Sun, D. Wu, Y. Li, J. Y. Liu, H. M. He and Z. R. Li, A theoretical study on novel alkaline earth-based excess electron compounds: unique alkalides with considerable nonlinear optical responses, Phys. Chem. Chem. Phys., 2015, 17, 4524–4532 RSC.
- W. Press, P. Damay, F. Leclercq and P. Chieux, On the structure of solid Ca(ND3)x; with x≈6, J. Chem. Phys., 1989, 91, 1167–1172 CrossRef CAS.
- P. Damay, F. Leclercq and P. Chieux, Geometry of the ND3group in a metallic Ca(ND3)6compound and in solid and liquid deuteroammonia as measured by neutron scattering, Phys. Rev. B: Condens. Matter Mater. Phys., 1990, 41, 9676–9682 CrossRef CAS.
- J. C. Wasse, C. A. Howard, H. Thompson, N. T. Skipper, R. G. Delaplane and A. Wannberg, The structure of calcium–ammonia solutions by neutron diffraction, J. Chem. Phys., 2004, 121, 996 CrossRef CAS PubMed.
- T. R. White, D. A. Gordon, R. F. Marzke, R. B. V. Dreele, J. L. Yarnell, A. L. Bowman and W. S. Glaunsinger, Structures and molecular motions in alkaline earth hexammines, Nature, 1978, 271, 414–417 CrossRef.
- W. S. Glaunsinger, R. B. Von Dreele, R. F. Marzke, R. C. Hanson, P. Chieux, P. Damay and R. Catterall, Structures and properties of metal-ammonia compounds on the trail of a new ammonia geometry, J. Phys. Chem., 1984, 88, 3860–3877 CrossRef CAS.
- I. C. Hwang, T. Drews and K. Seppelt, Mg(NH3)6Hg22, a Mercury Intercalation Compound, J. Am. Chem. Soc., 2000, 122, 8486–8489 CrossRef CAS.
- C. H. Christensen, R. Z. Sørensen, T. Johannessen, U. J. Quaade, K. Honkala, T. D. Elmøe, R. Køhler and J. K. Nørskov, Metal ammine complexes for hydrogen storage, J. Mater. Chem., 2005, 15, 4106 RSC.
- J. S. Hummelshøj, R. Z. Sørensen, M. Y. Kustova, T. Johannessen, J. K. Nørskov and C. H. Christensen, Generation of Nanopores during Desorption of NH3 from Mg(NH3)6Cl2, J. Am. Chem. Soc., 2006, 128, 16–17 CrossRef PubMed.
- C. H. Christensen, T. Johannessen, R. Z. Sørensen and J. K. Nørskov, Towards an ammonia-mediated hydrogen economy?, Catal. Today, 2006, 111, 140–144 CrossRef CAS.
- Y. Liu, R. Ma, R. Luo, K. Luo, M. Gao and H. Pan, Hydrogen Storage Properties of the Mg (NH3) 6Cl2-LiH Combined System, Mater. Trans., 2011, 52, 627–634 CrossRef CAS.
- M. H. Sørby, O. M. Løvvik, M. Tsubota, T. Ichikawa, Y. Kojima and B. C. Hauback, Crystal structure and dynamics of Mg(ND3)6Cl2, Phys. Chem. Chem. Phys., 2011, 13, 7644 RSC.
- F. Castet, V. Rodriguez, J. L. Pozzo, L. Ducasse, A. Plaquet and B. Champagne, Design and characterization of molecular nonlinear optical switches, Acc. Chem. Res., 2013, 46, 2656–2665 CrossRef CAS PubMed.
- N. N. Ma, S. J. Li, L. K. Yan, Y. Q. Qiu and Z. M. Su, Switchable NLO response induced by rotation of metallacarboranes [NiIII/IV(C2B9H11)2]−/0 and C-, B-functionalized derivatives, Dalton Trans., 2014, 43, 5069–5075 RSC.
- A. Plaquet, B. Champagne, J. Kulhanek, F. Bures, E. Bogdan, F. Castet, L. Ducasse and V. Rodriguez, Effects of the Nature and Length of the π-Conjugated Bridge on the Second-Order Nonlinear Optical Responses of Push–Pull Molecules Including 4,5-Dicyanoimidazole and Their Protonated Forms, ChemPhysChem, 2011, 12, 3245–3252 CrossRef CAS PubMed.
- J. H. Hou, Y. J. Liu, X. Zhang, Q. Duan, D. Y. Jiang, J. M. Qin and R. Q. Zhao, Electric-field-induced nonlinear optical switches of all-metal spherical aromatic molecules with infrared transparency: a theoretical study, New J. Chem., 2018, 42, 1031–1036 RSC.
- M. Nakano, T. Minami, K. Yoneda, S. Muhammad, R. Kishi, Y. Shigeta, T. Kubo, L. Rougier, B. Champagne, K. Kamada and K. Ohta, Giant Enhancement of the Second Hyperpolarizabilities of Open-Shell Singlet Polyaromatic Diphenalenyl Diradicaloids by an External Electric Field and Donor–Acceptor Substitution, J. Phys. Chem. Lett., 2011, 2, 1094–1098 CrossRef CAS.
- M. Nakano, B. Champagne, E. Botek, K. Ohta, K. Kamada and T. Kubo, Giant electric field effect on the second hyperpolarizability of symmetric singlet diradical molecules, J. Chem. Phys., 2010, 133, 154302 CrossRef PubMed.
- Y. Bai, Z. J. Zhou, J. J. Wang, Y. Li, D. Wu, W. Chen, Z. R. Li and C. C. Sun, The effects of external electric field: creating non-zero first hyperpolarizability for centrosymmetric benzene and strongly enhancing first hyperpolarizability for non-centrosymmetric edge-modified graphene ribbon H2N-(3,3)ZGNR-NO2, J. Mol. Model., 2013, 19, 3983–3991 CrossRef CAS.
- W. M. Sun, C. Y. Li, J. Kang, D. Wu, Y. Li, B. L. Ni, X. H. Li and Z. R. Li, Superatom Compounds under Oriented External Electric Fields: Simultaneously Enhanced Bond Energies and Nonlinear Optical Responses, J. Phys. Chem. C, 2018, 122, 7867–7876 CrossRef CAS.
- A. K. Jissy and A. Datta, Effect of External Electric Field on H-Bonding and π-Stacking Interactions in Guanine Aggregates, ChemPhysChem, 2012, 13, 4163–4172 CrossRef CAS.
- A. Susarrey-Arce, R. M. Tiggelaar, M. Morassutto, J. Geerlings, R. G. P. Sanders, B. Geerdink, S. Schlautmann, L. Lefferts, A. vanHouselt and J. G. E. Gardeniers, A new ATR-IR microreactor to study electric field-driven processes, Sens. Actuators, B, 2015, 220, 13–21 CrossRef CAS.
- P. M. De Biase, D. A. Paggi, F. Doctorovich, P. Hildebrandt, D. A. Estrin, D. H. Murgida and M. A. Marti, Molecular Basis for the Electric Field Modulation of Cytochrome c Structure and Function, J. Am. Chem. Soc., 2009, 131, 16248–16256 CrossRef CAS PubMed.
- M. J. Frisch, G. W. Trucks, H. B. Schlegel, G. E. Scuseria, M. A. Robb, J. R. Cheeseman, G. Scalmani, V. Barone, G. A. Petersson, H. Nakatsuji, X. Li, M. Caricato, A. V. Marenich, J. Bloino, B. G. Janesko, R. Gomperts, B. Mennucci, H. P. Hratchian, J. V. Ortiz, A. F. Izmaylov, J. L. Sonnenberg, D. Williams-Young, F. Ding, F. Lipparini, F. Egidi, J. Goings, B. Peng, A. Petrone, T. Henderson, D. Ranasinghe, V. G. Zakrzewski, J. Gao, N. Rega, G. Zheng, W. Liang, M. Hada, M. Ehara, K. Toyota, R. Fukuda, J. Hasegawa, M. Ishida, T. Nakajima, Y. Honda, O. Kitao, H. Nakai, T. Vreven, K. Throssell, J. A. Montgomery Jr, J. E. Peralta, F. Ogliaro, M. J. Bearpark, J. J. Heyd, E. N. Brothers, K. N. Kudin, V. N. Staroverov, T. A. Keith, R. Kobayashi, J. Normand, K. Raghavachari, A. P. Rendell, J. C. Burant, S. S. Iyengar, J. Tomasi, M. Cossi, J. M. Millam, M. Klene, C. Adamo, R. Cammi, J. W. Ochterski, R. L. Martin, K. Morokuma, O. Farkas, J. B. Foresman and D. J. Fox, Gaussian16, version B.01, Gaussian Inc., Wallingford CT, 2016 Search PubMed.
- T. Yanai, D. P. Tew and N. C. Handy, A new hybrid exchange–correlation functional using the Coulomb-attenuating method (CAM-B3LYP), Chem. Phys. Lett., 2004, 393, 51–57 CrossRef CAS.
- Y. Tawada, T. Tsuneda, S. Yanagisawa, T. Yanai and K. Hirao, A long-range-corrected time-dependent density functional theory, J. Chem. Phys., 2004, 120, 8425–8433 CrossRef CAS.
- W. M. Sun, D. Wu, Y. Li and Z. R. Li, Substituent Effects on the Structural Features and Nonlinear Optical Properties of the Organic Alkalide Li+(calix[4]pyrrole)Li−, ChemPhysChem, 2013, 14, 408–416 CrossRef CAS PubMed.
- M. Niu, G. T. Yu, G. H. Yang, W. Chen, X. G. Zhao and X. R. Huang, Doping the Alkali Atom: An Effective Strategy to Improve the Electronic and Nonlinear Optical Properties of the Inorganic Al12N12 Nanocage, Inorg. Chem., 2014, 53, 349–358 CrossRef CAS PubMed.
- W. M. Sun, D. Wu, Y. Li and Z. R. Li, Theoretical study on superalkali (Li3) in ammonia: Novel alkalides with considerably large first hyperpolarizabilities, Dalton Trans., 2014, 43, 486–494 RSC.
- S. J. Wang, Y. Li, Y. F. Wang, D. Wu and Z. R. Li, Structures and nonlinear optical properties of the endohedral metallofullerene-superhalogen compounds Li@ C 60–BX 4 (X= F, Cl, Br), Phys. Chem. Chem. Phys., 2013, 15, 12903–12910 RSC.
- W. M. Sun, X. H. Li, J. Wu, J. M. Lan, C. Y. Li, D. Wu, Y. Li and Z. R. Li, Can Coinage Metal Atoms Be Capable of Serving as an Excess Electron Source of Alkalides with Considerable Nonlinear Optical Responses?, Inorg. Chem., 2017, 56, 4594–4600 CrossRef CAS PubMed.
- P. A. Limacher, K. V. Mikkelsen and H. P. Lüthi, On the accurate calculation of polarizabilities and second hyperpolarizabilities of polyacetylene oligomer chains using the CAM-B3LYP density functional, J. Chem. Phys., 2009, 130, 194114 CrossRef PubMed.
- A. D. McLean and G. S. Chandler, Contracted Gaussian basis sets for molecular calculations. I. Second row atoms, Z=11–18, J. Chem. Phys., 1980, 72, 5639–5648 CrossRef CAS.
- E. Gross, J. Dobson and M. Petersilka, Density Functional Theory of Time-Dependent Phenomena, in Density Functional Theory II, Springer, 1996, pp. 81–172 Search PubMed.
- T. Lu and F. W. Chen, Multiwfn: a multifunctional wavefunction analyzer, J. Comput. Chem., 2012, 33, 580–592 CrossRef CAS PubMed.
- K. Sasagane, F. Aiga and R. Itoh, Higher-order response theory based on the quasienergy derivatives: The derivation of the frequency-dependent polarizabilities and hyperpolarizabilities, J. Chem. Phys., 1993, 99, 3738–3778 CrossRef CAS.
- S. Yamada, M. Nakano, I. Shigemoto and K. Yamaguchi, Static second hyperpolarizabilities γ of nitroxide radical and formaldehyde: evaluation of spatial contributions to γ by a hyperpolarizability density analysis, Chem. Phys. Lett., 1996, 254, 158–164 CrossRef CAS.
- H. Y. Wu, A. Chaudhari and S. Lee, Theoretical studies on nonlinear optical properties of formaldehyde oligomers by ab initio and density functional theory methods, J. Comput. Chem., 2005, 26, 1543–1564 CrossRef CAS PubMed.
- J. Boixel, V. Guerchais, H. L. Bozec, A. Chantzis, D. Jacquemin, A. Colombo, C. Dragonetti, D. Marinottoe and D. Robertode, Sequential double second-order nonlinear optical switch by an acido-triggered photochromic cyclometallated platinum(II) complex, Chem. Commun., 2015, 51, 7805–7808 RSC.
- G. Q. Mei, H. Y. Zhang and W. Q. Liao, A symmetry breaking phase transition-triggered high-temperature solid-state quadratic nonlinear optical switch coupled with a switchable dielectric constant in an organic–inorganic hybrid compound, Chem. Commun., 2016, 52, 11135–11138 RSC.
- M. Torrent-Sucarrat, J. M. Anglada and J. M. Luis, Evaluation of the nonlinear optical properties for annulenes with Huckel and Mobius topologies, J. Chem. Theory Comput., 2011, 7, 3935–3943 CrossRef CAS PubMed.
- M. Garcia-Borràs, M. Solà, J. M. Luis and B. Kirtman, Electronic and vibrational nonlinear optical properties of five representative electrides, J. Chem. Theory Comput., 2012, 8, 2688–2697 CrossRef PubMed.
- J. M. Luis, J. Martí, M. Duran, J. L. Andrés and B. Kirtman, Nuclear relaxation contribution to static and dynamic (infinite frequency approximation) nonlinear optical properties by means of electrical property expansions: Application to HF, CH4, CF4, and SF6, J. Chem. Phys., 1998, 108, 4123–4130 CrossRef CAS.
- J. M. Luis, M. Duran, B. Champagne and B. Kirtman, Determination of vibrational polarizabilities and hyperpolarizabilities using field-induced coordinates, J. Chem. Phys., 2000, 113, 5203–5213 CrossRef CAS.
- J. M. Luis, M. Duran, B. Champagne and B. Kirtman, Calculation of static zero-point vibrational averaging corrections and other vibrational curvature contributions to polarizabilities and hyperpolarizabilities using field-induced coordinates, Int. J. Quantum Chem., 2000, 80, 471–479 CrossRef CAS.
Footnote |
† Electronic supplementary information (ESI) available. See DOI: 10.1039/c9ra02470k |
|
This journal is © The Royal Society of Chemistry 2019 |
Click here to see how this site uses Cookies. View our privacy policy here.