DOI:
10.1039/C9RA01826C
(Review Article)
RSC Adv., 2019,
9, 19278-19284
Research progress of photocatalytic sterilization over semiconductors
Received
11th March 2019
, Accepted 12th May 2019
First published on 19th June 2019
Abstract
Considering the situation that environmental issues become more serious day by day, research on practical applications of semiconductor photocatalysis for environmental purification has attracted broad attention, including the remediation of water pollution, air contaminant treatment, photocatalytic sterilization etc., among which the application of semiconductor photocatalytic properties for the disinfection of soil surfaces, air and water, such as TiO2, is of great interest. In this paper, we give an overview of the photocatalytic antibacterial ability of TiO2 and other novel carbon material semiconductors. We have introduced the background information of photocatalytic disinfection and the disinfection mechanism of pure TiO2. Furthermore, other modified TiO2 sterilization materials are listed, such as those with doping modification. In addition, some novel carbon based nanomaterials are discussed as well in this review, for instance, g-C3N4, carbon nanotubes and graphene nanosheets. Finally, we present an outlook over two dimensional (2D) materials and coupling techniques based on the combination of photocatalysis and other sterilization technologies.
1 Introduction
With the increasing need of human material life, people pay more attention to the hygiene situation of work and living environments than ever, thus a variety of antibacterial materials have come into being. Commonly, antibacterial agents can be classified into two types: organic antibacterial agents and inorganic antibacterial agents.1,2 Moreover, inorganic antimicrobial agents mainly depend on silver ions, which merely kill bacteria, but cannot break down endotoxins. TiO2 photocatalytic sterilization can be used to overcome the shortcomings of the traditional silver inorganic antibacterial agents. The active hydroxyl radicals generated by TiO2 photocatalytic reactions possess a reaction energy of 120 kcal mol−1, which is higher than that of various chemical bonds in organic compounds, such as C–C (83 kcal mol−1), C–H (99 kcal mol−1), C–O (84 kcal mol−1), and H–O (111 kcal mol−1) bonds. Therefore, hydroxyl radicals can quickly and efficiently decompose organic compounds, such as the main component of the bacterium.3 Additionally, the synergy of hydroxyl radicals and other reactive radicals generated from the photocatalysis, for instance ˙OOH and O2˙− etc., makes the attacks on bacteria more frequent and causes reactions with biological macromolecules such as lipids, enzymes and nucleic acid macromolecules, which causes extensive damage to biological cell structures either directly or through a series of oxidative chain destructions.4 Moreover, some other nanomaterials, such as ZnO, CdS, CeO2 and BiVO4 etc., show excellent performance in photocatalytic sterilization.2,5–9 TiO2 has always been considered as the most promising catalyst in the photocatalytic disinfection of bacteria; however, due to the wide band gap of anatase TiO2 (3.2 vs. 3.0 eV of rutile), it is limited to UV light at wavelengths less than 385 nm, which accounts for less than 5% of solar energy.10,11 Thus, it is indeed of great necessity to expand its adsorption region from UV light to the visible light region. Some modification has been well done on TiO2, for instance non-metal and metal doping or construction of heterojunction semiconductors. Another effective strategy is to develop novel candidate nanomaterials for photocatalytic disinfection. Carbon materials, represented by g-C3N4, carbon nanotubes (CNTs) and graphene oxide (GO) have been deeply investigated in photocatalysis. Hence, in this review, we have not only reviewed the representative TiO2 nanomaterials, but also emphasized the brand new carbon nanomaterials of g-C3N4, carbon nanotubes (CNTs) and graphene oxide (GO) for disinfection applications. To this end, we propose the future research direction in photocatalytic sterilization over semiconductors.
2 Photocatalytic disinfection of TiO2
2.1 Photocatalytic disinfection of pure TiO2
Heterogeneous photocatalytic oxidation technology has always been a hot spot at home and abroad.12–14 Since 1972, Fujishima and Honda15 reported that the redox reaction of light-emitting TiO2 could sustainably take place in photovoltaic cells. After that, in 1976, Carey et al.16 successfully applied the TiO2 photocatalytic oxidation method to dechlorination and detoxification of PCB compounds in water. In 1985, Tadashi Matsunaga et al.17 firstly found that TiO2 had a bactericidal effect under ultraviolet light. The experimental results showed that with the loading of TiO2 nanoparticles, co-cultured Lactobacillus acidophilus, yeast, and Escherichia coli could be completely killed under the irradiation of a halide lamp for 60–120 min. Their findings opened a new era in the field of sterilization, thus resulting in attempts to use this novel photocatalytic technology for disinfecting drinking water and removing bioaerosols from indoor air environments. In 1993, Ireland et al.18 found that titanium dioxide in the anatase phase could be used as a photocatalyst to generate hydroxyl radicals in a Flow-Through water reactor as shown in Fig. 1.
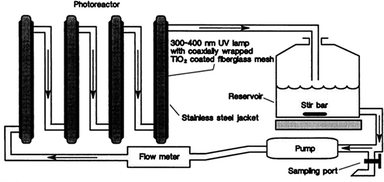 |
| Fig. 1 Schematic diagram of the experimental TiO2 photoreactor showing the flow configuration (this figure has been reproduced from ref. 18 with permission from Elsevier). | |
In 1994, Wei et al.19 found that the irradiation of suspensions containing Escherichia coli (∼106 cells per mL) and TiO2 (anatase) under UV-visible light with a wavelength longer than 380 nm resulted in the killing of the bacteria within minutes. Apart from Escherichia coli, Giardia lamblia is another target in photocatalytic sterilization. In 2004, Lee et al.20 utilized a TiO2 colloidal solution to sterilize the Giardia lamblia. To be more specific, the TiO2 solution was made into films using a modified hydrothermal method, and directly coated on a UV-lamp. As a result, the TiO2 films were transparent until 5-times coating. In 2015, Wang et al.21 reported visible-light-sensitive sub-5 nm TiO2 nanoparticles, which were applied in the degradation of organic pollutants in water and air, and the bacterial disinfection field. Those sub-5 nm TiO2 nanoparticles were fabricated without any doping or calcination treatments, maintaining abundant hydroxyls on the surfaces and subsurface, which indicated a high ratio of surface to bulk defects. Fig. 2(a) shows a lattice space of ∼0.36 nm, which is indexed to the {101} facets of anatase TiO2. The gel (1.0 wt%) shows a high efficiency for visible-light-driven photocatalytic dye degradation (Fig. 2(c)). Significantly, the TiO2 gel also shows impressive durability, with almost no loss of visible-light activity after 5 runs. Finally, qualitative (Fig. 2(b)) antibacterial evaluations are considered for TiO2 gel. It can effectively sterilize bacteria SA under visible light.
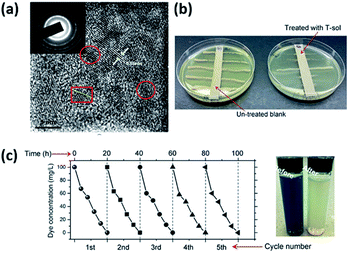 |
| Fig. 2 (a) HRTEM image of T-sol. (b) Visible-light-driven photocatalytic sterilization of bacteria SA through qualitative. (c) Recyclability tests of T-sol for total 5 runs of the dye degradation under visible light (this figure has been adapted from ref. 21 with permission from Royal Society of Chemistry). | |
2.2 The mechanism of the antibacterial properties of TiO2
Advanced Oxidation Processes (AOPs) involving TiO2-mediated photocatalysis technology have attracted considerable attention from scientists. Among them, the photocatalytic oxidation process is mainly started by photogenerated electrons and holes through the irradiation of a semiconductor (e.g., such as TiO2) via sunlight. When irradiated by light, the electrons (e−) are excited into the conduction band (CB), thus leaving a hole (h+) in the valence band (VB) of the photocatalyst. Then, these charge carriers (e−/h+ pair) migrate to the surface/interface of the photocatalyst and participate in the redox reactions. The whole mechanism is illustrated in eqn (1)–(8) as follows, involving reactive oxygen species (ROS) species such as HO˙, O2˙−, HO2˙, etc. It is recognized that hydroxyl radicals are primarily responsible for this disinfection property, especially against bacteria.22 |
TiO2 + hv → TiO2 (e−CB + h+VB)
| (1) |
|
TiO2 (h+VB) + H2Oad → TiO2 + ˙OHad + H
| (2) |
|
TiO2 (h+VB) + −OHad → TiO2 + ˙OH
| (3) |
|
TiO2 (e−CB) + O2 → TiO2 + O2˙−
| (4) |
|
O2˙− + HO2˙ → ˙OH + O2 + H2O2
| (6) |
|
TiO2 (e−CB) + H2O2 → TiO2 + −OH + ˙OH
| (8) |
Furthermore, other ROS species (such as singlet oxygen and hydrogen peroxide) also play a pivotal role in the sterilization, as they can actively participate in the oxidation of cellular components, membrane leakage of the microbial cell wall, and other processes.23 As shown in Fig. 3, the full mechanism was clearly exhibited. Other various types of metal oxide and metal modified TiO2 were also displayed, such as the following example consisting of Ag+ as the Mn+ species.23 Firstly, TiO2 based photocatalysts were mixed with bacteria, and then could be adsorbed onto the surface of cell membranes. After irradiation by sunlight, the ROS/Mn+ would start to attack cells, the cell membranes were destroyed and were finally deactivated.22
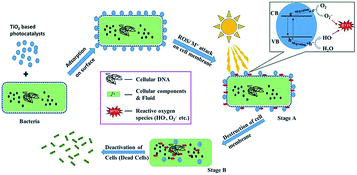 |
| Fig. 3 The mechanism of the photocatalytic disinfection of bacteria (this figure has been reproduced from ref. 23 with permission from Elsevier). | |
2.3 Photocatalytic disinfection of modified TiO2 materials
In addition to the application of pure TiO2 nanoparticles in sterilization, other modified TiO2 materials and TiO2 nanocomposites have been extensively investigated. Trapalis et al.24 studied the bactericidal activity against Escherichia coli of Fe3+ doped TiO2 thin films on glass substrate. Sayılkan et al.25 prepared Sn4+ doped TiO2 (TiO2-Sn4+) particulates by a hydrothermal process. TiO2-Sn4+ was coated onto glass surfaces to investigate the photocatalytic antibacterial effect of Sn4+ doping to TiO2 against Gram negative Escherichia coli (E. coli) and Gram positive Staphylococcus aureus (S. aureus). Fig. 4(a) elaborately shows the preparation steps of TiO2-Sn4+ particulates. Prior to preparing the coating solution, TiO2-Sn4+ sols composed of a large number of TiO2-Sn4+ particulates were prepared by dispersing the particles into deionized water in the absence of dispersant. Then the mixture was merely treated in an ultrasonic bath for a few minutes, thus the transparent TiO2-Sn4+ sols were obtained. The bacterial test results for Escherichia coli are shown in Fig. 4(b). It could be clearly seen that 50% (w/w) TiO2-Sn4+ exhibited the optimal antibacterial effect.
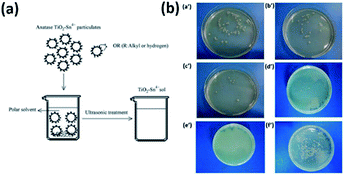 |
| Fig. 4 (a) Preparation steps of transparent TiO2-Sn4+ sol in polar solvent system. (b) Photo images for the results of E. coli test with the coated surfaces containing (a′) 10, (b′) 20, (c′) 30, (d′) 40 and (e′) 50% TiO2-Sn4+, and (f) uncoated surface (this figure has been reproduced from ref. 25 with permission from Elsevier). | |
Furthermore, Wu et al.26 synthesized a photocatalyst called PdO/TiON composed of palladium oxide and nitrogen-doped titanium oxide using a sol–gel process. Moreover, the photocatalytic activity of the PdO/TiON catalyst was investigated by using different bacterial indicators, including Gram-negative cells of Escherichia coli and Pseudomonas aeruginosa, and Gram-positive cells of Staphylococcus aureus. In 2010, Karunakaran et al.27 reported the highly efficient photocatalytic disinfection of bacteria using Cu-doped TiO2 under visible light for the first time. Additionally, a TiO2–NiFe2O4 photocatalyst was synthesized by Misra.28 In this work, the reverse micelle and chemical hydrolysis techniques had been successfully combined to synthesize composite nanoparticles consisting of a photocatalytic shell of titania and a magnetic core of nickel ferrite. Fig. 5(a) shows the TEM and HRTEM images of anatase and brookite titania-coated NiFe2O4 nanoparticles. Fig. 5(b) presents the degradation efficiency of methyl orange under UV light.
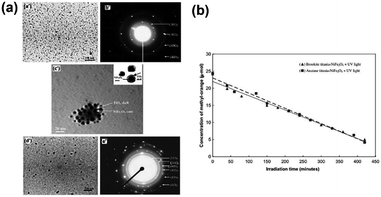 |
| Fig. 5 (a) TEM images (a′) of and (b′) electron diffraction pattern for anatase titania-coated NiFe2O4, (c′) HRTEM image of core–shell structure for anatase titania-coated NiFe2O4 nanoparticles, (d′) TEM image and (e′) the corresponding electron diffraction for brookite titania-coated NiFe2O4. (b) Concentration of methyl-orange versus irradiation time in the presence of anatase- and brookite-TiO2-coated NiFe2O4 nanoparticles (this figure has been reproduced from ref. 28 with permission from Elsevier). | |
Misra and his co-workers29 introduced Nd3+ dopant into the TiO2 shell of titania-coated nickel ferrite composite nanoparticles. As a result, the as-prepared catalyst could significantly enhance the photocatalytic degradation of methyl orange and the anti-microbial performance, which was attributed to the restriction of electron–hole recombination and the decrease of the titania band gap energy. Moreover, the retention of magnetic strength ensured that the movement of the composite nanoparticles could be controlled by a magnetic field, facilitating their application as removable anti-microbial photocatalyst nanoparticles. Fig. 6(a) shows the HRTEM image of the TiO2–NiFe2O4 composite. The NiFe2O4 magnetic core is 4–6 nm and the TiO2 shell is 2–3 nm, whereas the HRTEM image of Nd3+-doped TiO2-coated NiFe2O4 nanoparticles is shown in Fig. 6(c). The NiFe2O4 magnetic core is 8–10 nm and the TiO2 shell is 2–3 nm. The specific degradation performance of methyl orange and E. coli bacterial inactivation activity are exhibited in Fig. 6(b) and (d).
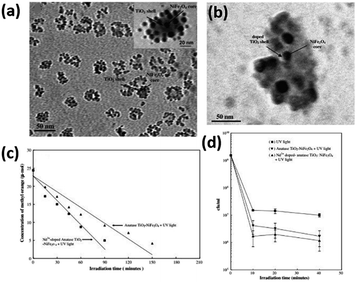 |
| Fig. 6 (a) HRTEM image of core–shell structure for undoped TiO2–NiFe2O4 composite nanoparticles, illustrating the core–shell structure in the inset. (b) Concentration of methyl-orange versus irradiation time in the presence of undoped TiO2-coated NiFe2O4 nanoparticles and Nd3+-doped TiO2-coated NiFe2O4 nanoparticles. (c) HRTEM image of core–shell structure for Nd3+-doped TiO2:NiFe2O4 composite nanoparticles. (d) E. coli bacterial inactivation response of Nd3+-doped TiO2-coated NiFe2O4 nanoparticles and undoped TiO2-coated NiFe2O4 nanoparticles as a function of irradiation time (this figure has been reproduced from ref. 29 with permission from Elsevier). | |
Similarly, Nardi et al.30 investigated functionally graded epoxy composites with various concentration profiles of Fe3O4@TiO2 core–shell nanoparticles (NPs), and focused on their antibacterial properties. It was revealed that the composite surfaces showed effective antibacterial performance but were inactivated in a few hours, which was attributed to the suitable amount of NPs and their anisotropic distribution in the subsurface layers facing the bacteria.
In the above section, we have reviewed the disinfection ability of pure TiO2 and modified TiO2 nanomaterials, accompanied by the disinfection mechanism. It is well known that hydroxyl radicals are primarily responsible for this disinfection property. Besides, other reactive oxygen species (ROS, such as singlet oxygen and hydrogen peroxide) also play a pivotal role in sterilization. What’s more, on one hand, one of the obstacles in employing TiO2 nanomaterials for industrial use is the recycling problem due to the small particle size. On the other hand, the light utilization efficiency of TiO2 was mainly restricted to the UV region, which restrains the application in practical photocatalytic disinfection of contaminated water. Thus, to some extent, the modification of TiO2 nanomaterials, such as non-metal and metal doping, compounded with other semiconductors, is beneficial for fully utilizing solar light.
3 Photocatalytic sterilization of carbon materials
Apart from the conventional photocatalysts of metal oxide semiconductors, carbon based semiconductors, such as g-C3N4, have been well considered to be highly efficient visible-light-driven photocatalysts in recent years. g-C3N4 is a semiconductor material with a narrow band gap and is equipped with visible light response, which can increase the separation efficiency of photogenerated electron–hole pairs.31 Thus, g-C3N4 has been used to modify TiO2 for improving its photocatalytic performance. For instance, Tian et al.32 prepared g-C3N4/TiO2 composite photocatalysts, which were applied to enhance the sterilization performance of fluorocarbon resin (PEVE) coatings. The g-C3N4 was prepared using melamine thermolysis as shown in Fig. 7. The antibacterial activity of coatings under UV and visible light were also investigated. The sterilization performance of the PEVE composite coatings was enhanced by the addition of TiO2 and g-C3N4/TiO2 under UV and visible-light irradiation.
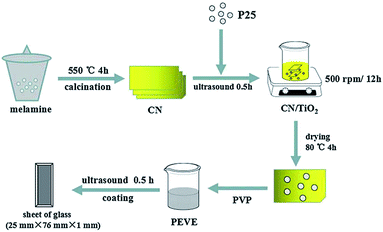 |
| Fig. 7 The preparation progress of CNTP coatings (this figure has been reproduced from ref. 31 with permission from American Chemical Society). | |
What’s more, in 2016, Bi2MoO6/g-C3N4 heterojunctions were fabricated using an in situ solvothermal method via using g-C3N4 nanosheets as precursors by Li et al.33 The photocatalytic activities were tested with hydrogen evolution from water splitting and disinfection of bacteria under visible light irradiation. The results indicated that exfoliating bulk g-C3N4 to g-C3N4 nanosheets greatly enlarged the specific surface area and shortened the diffusion distance for photogenerated charges, which could not only promote the photocatalytic performance but also benefit the sufficient interaction with Bi2MoO6, as shown in Fig. 8. The 20%-BM/CNNs heterojunction exhibited the optimal photocatalytic hydrogen evolution as well as photocatalytic disinfection of bacteria. This work extended the scope of g-C3N4-based photocatalysts to the growing demand of clean new energy and drinking water.
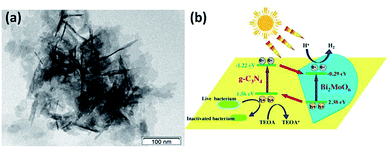 |
| Fig. 8 TEM image (a) and schematic diagram (b) of the photocatalytic mechanism of the Bi2MoO6/g-C3N4 composite (this figure has been reproduced from ref. 33 with permission from Elsevier). | |
Zhu’s group prepared a porous g-C3N4 nanosheet (PCNS) photocatalyst with a thickness of 2.0 nm using a simple two-step template-free approach without the addition of extra reagents.34 In comparison with the bulk g-C3N4 (BCN), PCNSs possess more surface reactive sites, which obviously improve the charge transfer efficiency, and thereby accelerates the separation of photogenerated electron–hole pairs. As a result, the visible-light-driven photocatalytic disinfection performance of the PCNSs was significantly enhanced. Escherichia coli (E. coli) cells could be killed completely by PCNSs within 4 h, whereas only 77.1% of them could be killed by BCN. Fig. 9 clearly illustrates the disinfection of porous g-C3N4 nanosheets (PCNS) and the TEM images of E. coli cells after disinfection for 4 h by using PCNS.
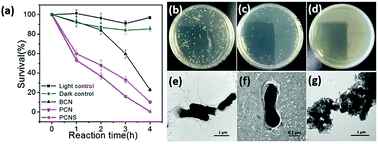 |
| Fig. 9 (a) Visible-light-driven photocatalytic disinfection performance against E. coli between BCN, PCN, and PCNS. Images of E. coli colonies on solid culture medium (b) before irradiation and (c) after disinfection for 2 h and (d) for 4 h using PCNS. TEM images of E. coli cells (e) before irradiation and (f, g) after disinfection for 4 h using PCNS (this figure has been reproduced from ref. 34 with permission from American Chemical Society). | |
Besides g-C3N4, other carbon based antibacterial nanomaterials, including carbon nanotubes (CNTs) and graphene oxide (GO), are always renewable, easier to obtain and cheaper than silver materials and metal oxides.35 CNTs are the type of carbon nanomaterials with a hollow structure and large surface area. However, CNTs have been found to be cytotoxic recently.36,37 Fortunately, graphene, another type of carbon nanomaterial, and its derivatives have high antibacterial activity due to physical damage that occurs upon direct contact of bacterial membranes with sharp edges of the graphene sheets; however, the improved oxidative stress induced by graphene-based materials can lead to the apoptosis of mammalian cells.38 Bao et al.39 successfully fabricated GO nanosheets, which were impregnated with silver nanoparticles (Ag NPs) via the in situ reduction of adsorbed Ag+ by hydroquinone (HQ) in a citrate buffer solution. The Ag NP/GO composites performed efficiently in decreasing the count of E. coli from 106 CFU mL−1 to 0 with 45 mg L−1 GO in water.
A possible mechanism proposed for the GO nanosheet mediated Ag deposition process is schematically illustrated in Fig. 10(a). The Ag NPs are homogeneously assembled on the micron scale GO (Fig. 10(b)). To check the antibacterial effect in aquatic media, GO and composite suspensions were added into bacteria-containing water, respectively. In Fig. 10(c and d), as to the GO samples, no obvious antibacterial effect was observed, whereas the bacteria cells of E. coli or S. aureus were reduced greatly in the presence of the composites.
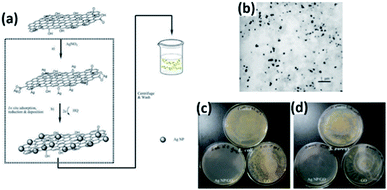 |
| Fig. 10 (a) Proposed scheme for the fabrication of the Ag NP/GO composite suspension. (b) TEM image of Ag NP/GO composites. (c) Photographs of colonies of E. coli and (d) S. aureus cultivated on MH agar plates obtained from incubated suspensions with water (control), GO and Ag NP/GO composites (this figure has been reproduced from ref. 39 with permission from Elsevier). | |
More importantly, as a common medical reagent, H2O2 is widely used in wound disinfection to avoid bacterial infection. Nevertheless, the disinfection ability of H2O2 is lower than that of hydroxyl radicals, so a high concentration of H2O2 is needed to solve this problem.40 Graphene quantum dots (GQDs) are defined as a kind of 0D material, and possess characteristics derived from both graphene and carbon dots (CDs).41 Simultaneously, GQDs display a higher peroxidase-like activity than graphene due to their excellent electron transportation properties.42,43 Sun et al.44 reported an antibacterial system combining the “safe” carbon based nanomaterials of GQDs with a low level of H2O2. All the experimental data in vitro showed that this intrinsic activity realized a high enhancement of the antibacterial activity of H2O2, and the particular system exhibited broad application of antibacterial activity against not only Gram-negative (Escherichia coli) but also Gram-positive (Staphylococcus aureus) bacteria. Fig. 11(a) illustrates a more detailed mechanism. In the presence of GQDs, H2O2 could be converted into hydroxyl radicals (high antibacterial activity), thus improving the antibacterial performance of H2O2, which made it possible to save the cost of a high concentration of H2O2 in wound disinfection. What’s more, GQD-Band-Aids were prepared and used in wound disinfection in vivo (seen from Fig. 11(b)).
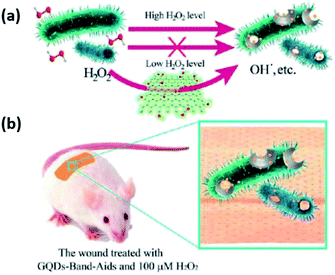 |
| Fig. 11 (a) The system based on GQDs and low levels of H2O2 for antibacterial applications. (b) The GQD Band-Aids used in wound disinfection in vivo (this figure has been reproduced from ref. 44 with permission from American Chemical Society). | |
In this section, we have reviewed novel carbon materials, such as g-C3N4 nanosheets, GO nanosheets and graphene quantum dots in photocatalytic sterilization. It is expected that some other carbon materials and 2D transition metal materials may act as alternative candidates in this field as well.
Table 1 summarizes the ability of different photocatalysts for the inactivation of bacteria including Gram-positive and Gram-negative bacteria. It is revealed that TiO2 nanomaterials need less time to achieve high efficiency under UV light. After the TiO2 materials were modified such as doping with metal ions, the disinfection ability can be extended to visible light region. In comparison with TiO2, the C3N4 based nanomaterials could work for disinfection under visible light irradiation. When the Ag nanoparticles were loaded onto GO sheets or GQDs, it can also realize visible light driven disinfection, however, it always needs a long reaction time. Even so, it is much better than the disinfection efficiency of graphene based nanomaterials (i.e. graphene quantum dots) in the dark. Therefore, TiO2 is still an ideal UV-driven photocatalyst for the inactivation of bacteria, while C3N4 is a potential candidate for visible light-driven disinfection. In order to improve the efficiency of photocatalytic disinfection (or sterilization), composite modification of a variety of catalysts will be the focus of research.
Table 1 Comparison of various photocatalysts for disinfection
Bacteria |
Concentration |
Photocatalyst |
Duration |
Light source |
Ref. |
E. coli |
∼106 CFU mL−1 |
TiO2 (anatase) |
120 min |
UV light |
19 |
G. lamblia |
|
TiO2 film (anatase) |
60 min |
UV light |
20 |
S. aureus |
8.55 × 104 CFU mL−1 |
TiO2 (anatase) |
20 h |
Visible light |
21 |
E. coli |
106 CFU mL−1 |
Fe3+-doped TiO2 |
4 h |
UV light |
24 |
E. coli, S. aureus |
|
Sn4+-doped TiO2 |
10 h |
UV light |
25 |
E. coli |
106 CFU mL−1 |
Nd3+-doped TiO2–NiFe2O4 |
150 min |
UV light |
29 |
E. coli |
2.5 × 107 CFU mL−1 |
Bi2MoO6/g-C3N4 |
5 h |
Visible light |
33 |
E. coli |
5.0 × 106 CFU mL−1 |
g-C3N4 nanosheets |
4 h |
Visible light |
34 |
E. coli |
106 CFU mL−1 |
Ag NP/GO sheets |
24 h |
Visible light |
39 |
E. coli |
106 CFU mL−1 |
Graphene quantum dots |
48 h |
Dark |
44 |
4 Conclusions and outlook
In summary, since the discovery of the application of TiO2 in photocatalytic sterilization, scientists around the world have made many efforts to develop huge amounts of nanomaterials, which are commonly operated under visible light or UV light irradiation for photocatalytic sterilization. Commonly, the most widely used photocatalyst is still TiO2. In addition, after several years of development, some novel carbon based photocatalytic nanomaterials emerged, such as g-C3N4, carbon materials and graphene. These materials can act as independent materials or excellent supporting materials in disinfection. In combination with the present trend, 2D transition metal materials may possess similar or more excellent ability in photocatalytic sterilization, owing to the large surface area and excellent utilization of light. Although photocatalytic sterilization has made great progress in recent years, one of the main obstacles to bringing photocatalytic water disinfection into practical application is photocatalyst recycling and photocatalytic reactor design. This is because most of the synthesized photocatalysts are in powder form, which is difficult to be reunited and recycled in industry. Additionally, the light utilization of semiconductor photocatalysts in water is very limited, leading to low efficiency of disinfection rate. All of these problems greatly limit the possibility of practical application of photocatalytic sterilization in the future. Regarding this, future research direction may focus on the synthesis of photocatalytic membranes, magnetic photocatalysts and three-dimensional catalysts that can be easily recycled. Moreover, it is imperative to develop other coupling techniques with the photocatalytic method, such as Advanced Oxidation Processes (AOPs), including Fenton, Fenton-like and photo-Fenton processes and peroxymonosulfate (PMS) activation etc. Therefore, improving the dispersion of the semiconductor photocatalysts in water and the utilization of light, and the development of the coupling techniques based on the combination of photocatalysis and other sterilization technologies such as adsorption or AOPs are major research areas that need to be further investigated.
Conflicts of interest
There are no conflicts to declare.
Acknowledgements
This work has been supported by the State Key Research Development Program of China (No. 2016YFA0204200), National Nature Science Foundation of China (No. 81401466, 21822603, 81501521).
Notes and references
- W. Raza, S. M. Faisal, M. Owais, D. Bahnemann and M. Muneer, RSC Adv., 2016, 6, 78335–78350 RSC.
- V. Shanmugam and K. S. Jeyaperumal, Appl. Surf. Sci., 2018, 449, 617–630 CrossRef CAS.
- S. Sepahvand and S. Farhadi, RSC Adv., 2018, 8, 10124–10140 RSC.
- A. U. Rahman, A. U. Khan, Q. Yuan, Y. Wei, A. Ahmad, S. Ullah, Z. U. H. Khan, S. Shams, M. Tariq and W. Ahmad, J. Photochem. Photobiol., B, 2019, 193, 31–38 CrossRef CAS PubMed.
- G. R. S. Andrade, C. C. Nascimento, Z. M. Lima, E. Teixeira-Neto, L. P. Costa and I. F. Gimenez, Appl. Surf. Sci., 2017, 399, 573–582 CrossRef CAS.
- J. Liu, Y. Wang, J. Ma, Y. Peng and A. Wang, J. Alloys Compd., 2019, 783, 898–918 CrossRef CAS.
- K. Qi, B. Cheng, J. Yu and W. Ho, J. Alloys Compd., 2017, 727, 792–820 CrossRef CAS.
- L. Midya, A. S. Patra, C. Banerjee, A. B. Panda and S. Pal, J. Hazard. Mater., 2019, 369, 398–407 CrossRef CAS PubMed.
- B. Najma, A. K. Kasi, J. Khan Kasi, A. Akbar, S. M. A. Bokhari and I. R. C. Stroe, Appl. Surf. Sci., 2018, 448, 104–114 CrossRef CAS.
- V. Shanmugam and K. S. Jeyaperumal, Appl. Surf. Sci., 2018, 449, 617–630 CrossRef CAS.
- X. Zeng, Y. Wan, X. Gong and Z. Xu, RSC Adv., 2017, 7, 36269–36278 RSC.
- N. Serpone, Res. Chem. Intermed., 1994, 20, 953–992 CrossRef CAS.
- U. Stafford, K. A. Gray and P. V. Kamat, Res. Chem. Intermed., 1997, 23, 355–388 CrossRef CAS.
- Y. G. Shul, H. J. Kim, S. J. Haam and H. S. Han, Res. Chem. Intermed., 2003, 29, 849–859 CrossRef CAS.
- A. Fujishima, Nature, 1972, 238, 37–38 CrossRef CAS PubMed.
- J. H. Carey, J. Lawrence and H. M. Tosine, Bull. Environ. Contam. Toxicol., 1976, 16, 697–701 CrossRef CAS PubMed.
- T. Matsunaga, R. Tomoda, T. Nakajima and H. Wake, FEMS Microbiol. Lett., 1985, 29, 211–214 CrossRef CAS.
- J. C. Ireland, P. Klostermann, E. W. Rice and R. M. Clark, Appl. Environ. Microbiol., 1993, 59, 1668–1670 CAS.
- C. Wei, W. Y. Lin, Z. Zainal, N. E. Williams, K. Zhu, A. P. Kruzic, R. L. Smith and K. Rajeshwar, Environ. Sci. Technol., 1994, 28, 934–938 CrossRef CAS PubMed.
- J. H. Lee, M. Kang, S.-J. Choung, K. Ogino, S. Miyata, M.-S. Kim, J.-Y. Park and J.-B. Kim, Water Res., 2004, 38, 713–719 CrossRef CAS PubMed.
- X. Wang, H. Hu, Z. Yang, Y. Kong, B. Fei and J. H. Xin, Catal. Commun., 2015, 72, 81–85 CrossRef CAS.
- P. V. Laxma Reddy, B. Kavitha, P. A. Kumar Reddy and K.-H. Kim, Environ. Res., 2017, 154, 296–303 CrossRef CAS PubMed.
- P. A. K. Reddy, P. V. L. Reddy, E. Kwon, K.-H. Kim, T. Akter and S. Kalagara, Environ. Int., 2016, 91, 94–103 CrossRef CAS PubMed.
- C. C. Trapalis, P. Keivanidis, G. Kordas, M. Zaharescu, M. Crisan, A. Szatvanyi and M. Gartner, Thin Solid Films, 2003, 433, 186–190 CrossRef CAS.
- F. Sayılkan, M. Asiltürk, N. Kiraz, E. Burunkaya, E. Arpaç and H. Sayılkan, J. Hazard. Mater., 2009, 162, 1309–1316 CrossRef PubMed.
- P. Wu, R. Xie, J. A. Imlay and J. K. Shang, Appl. Catal., B, 2009, 88, 576–581 CrossRef CAS PubMed.
- C. Karunakaran, G. Abiramasundari, P. Gomathisankar, G. Manikandan and V. Anandi, J. Colloid Interface Sci., 2010, 352, 68–74 CrossRef CAS PubMed.
- S. Rana, J. Rawat and R. D. K. Misra, Acta Biomater., 2005, 1, 691–703 CrossRef CAS PubMed.
- S. Rana, J. Rawat, M. M. Sorensson and R. D. K. Misra, Acta Biomater., 2006, 2, 421–432 CrossRef CAS PubMed.
- T. Nardi, S. Rtimi, C. Pulgarin and Y. Leterrier, RSC Adv., 2015, 5, 105416–105421 RSC.
- W.-J. Ong, L.-L. Tan, Y. H. Ng, S.-T. Yong and S.-P. Chai, Chem. Rev., 2016, 116, 7159–7329 CrossRef CAS PubMed.
- Y. Tian, F. Zhou, S. Zhan, Y. Yang, Y. Liu and Q. He, J. Inorg. Organomet. Polym., 2017, 27, 353–362 CrossRef CAS.
- J. Li, Y. Yin, E. Liu, Y. Ma, J. Wan, J. Fan and X. Hu, J. Hazard. Mater., 2017, 321, 183–192 CrossRef CAS PubMed.
- J. Xu, Z. Wang and Y. Zhu, ACS Appl. Mater. Interfaces, 2017, 9, 27727–27735 CrossRef CAS PubMed.
- W. Hu, C. Peng, W. Luo, M. Lv, X. Li, D. Li, Q. Huang and C. Fan, ACS Nano, 2010, 4, 4317–4323 CrossRef CAS PubMed.
- D. B. Warheit, B. R. Laurence, K. L. Reed, D. H. Roach, G. A. M. Reynolds and T. R. Webb, Toxicol. Sci., 2004, 77, 117–125 CrossRef CAS PubMed.
- A. Magrez, S. Kasas, V. Salicio, N. Pasquier, J. W. Seo, M. Celio, S. Catsicas, B. Schwaller and L. Forró, Nano Lett., 2006, 6, 1121–1125 CrossRef CAS PubMed.
- A. M. Pinto, I. C. Gonçalves and F. D. Magalhães, Colloids Surf., B, 2013, 111, 188–202 CrossRef CAS PubMed.
- Q. Bao, D. Zhang and P. Qi, J. Colloid Interface Sci., 2011, 360, 463–470 CrossRef CAS PubMed.
- E. Hayashi, T. Mokudai, Y. Yamada, K. Nakamura, T. Kanno, K. Sasaki and Y. Niwano, J. Biosci. Bioeng., 2012, 114, 193–197 CrossRef CAS PubMed.
- J. Shen, Y. Zhu, X. Yang and C. Li, Chem. Commun., 2012, 48, 3686–3699 RSC.
- A.-X. Zheng, Z.-X. Cong, J.-R. Wang, J. Li, H.-H. Yang and G.-N. Chen, Biosens. Bioelectron., 2013, 49, 519–524 CrossRef CAS PubMed.
- Y. Zhang, C. Wu, X. Zhou, X. Wu, Y. Yang, H. Wu, S. Guo and J. Zhang, Nanoscale, 2013, 5, 1816–1819 RSC.
- H. Sun, N. Gao, K. Dong, J. Ren and X. Qu, ACS Nano, 2014, 8, 6202–6210 CrossRef CAS PubMed.
|
This journal is © The Royal Society of Chemistry 2019 |