DOI:
10.1039/C9RA00833K
(Paper)
RSC Adv., 2019,
9, 9654-9662
Novel mono-lipidated dimeric glucagon-like peptide-1 receptor agonist with improved long-acting and hypoglycemic activity†
Received
31st January 2019
, Accepted 14th March 2019
First published on 26th March 2019
Abstract
Dimerization is a useful tool to boost ligand–receptor interaction. Both lipidation and dimerization effectively prolong the short half-life (t1/2) of peptides by facilitating binding with serum albumin and increasing hydrodynamic size. Here, we described two novel GLP-1 conjugates with high glucagon-like peptide-1 (GLP-1) receptor activation potencies, dimerized GLP-1 (Di-GLP-1) and lipidated Di-GLP-1 (Lip-Di-GLP-1). Di-GLP-1 and Lip-Di-GLP-1 were prepared through cysteine–maleimide specific coupling reactions using Gly8-Cys31-GLP-1, bis-maleimide amine, and activated palmitic acid. The receptor activation potencies of Di-GLP-1 and Lip-Di-GLP-1 were 13.6-fold and 9.5-fold higher than GLP-1, respectively. The in vivo hypoglycemic and insulinotropic activities of Di-GLP-1 and Lip-Di-GLP-1 were also better than GLP-1 in db/db mice. Furthermore, Lip-Di-GLP-1 was found to have greater circulating t1/2 than synthesized liraglutide by 1.8-fold. Accordingly, the improved pharmacokinetic profiles of Lip-Di-GLP-1 resulted in protracted antidiabetic effects as confirmed by hypoglycemic duration test. Moreover, Lip-Di-GLP-1 administered in mice potently inhibits gastric emptying and reduce food intake. Chronic Lip-Di-GLP-1 treatment in db/db mice resulted in significant improvements in food intake, body weight, pancreatic function and corrected hyperglycemia, which was more effective than synthesized liraglutide. Our research indicated that combined dimerization and lipidation were effectively applied to GLP-1, and the preclinical results suggested the potential usage of Lip-Di-GLP-1 as a long-acting antidiabetic agent.
Introduction
Glucagon-Like Peptide-1 (GLP-1), a naturally occurring incretin hormone, is secreted from intestinal L-cells into the blood stream in response to nutrient intake.1 GLP-1 is a potent anti-hyperglycemic hormone possessing multiple endocrine actions. The stimulation of insulin secretion by GLP-1 is glucose-dependent with suppressed glucagon secretion. The glucose-dependent action of GLP-1 is particularly attractive as GLP-1 no longer stimulates insulin secretion when the fasting blood glucose level is in the normal range to avoid hypoglycemia.2,3 Moreover, GLP-1 stimulates the differentiation and proliferation of insulin-secreting β-cells and inhibits pancreatic β-cell apoptosis.4 In addition, GLP-1 is also known to inhibit gastric secretion and motility which contributes to a satiating effect through delays carbohydrate absorption. Furthermore, GLP-1 could lowering body weight through reducing caloric consumption and feelings of hunger in humans, including overweight people with type 2 diabetes.5 In conclusion, GLP-1 is a promising therapeutic agent that could stabilize or reverse disease progression of type 2 diabetes mellitus (T2DM). However, early attempts to use GLP-1 for the treatment of T2DM met with limited success, due to the short biological half-life of GLP-1. GLP-1 is rapidly degraded in vivo due to rapid enzymatic degradation by dipeptidyl peptidase IV (DPP-IV) and renal clearance. Thus, numerous research efforts were mainly focused on the development of stable GLP-1 analogs and/or the sustained delivery of these analogs.6
In nature, dimeric interactions are frequently occurred to improve the affinity of ligand–receptor interactions.7 Thus, the possibility of using this strategy to improve the therapeutic potencies of peptide has attracted the interest of researchers. As a result, many research groups have using this strategy to produce peptides with enhanced interactions between ligands and target cells. Lee et al. designed dimer exendin-4 analog with enhanced receptor binding activities, and further PEGylation of dimer exendin-4 analog successfully improved its in vivo long-acting antidiabetic activities.8
Lipidization of peptide drugs with long chain fatty acids is a widely used strategy to enhance the stabilities of peptide drugs.9 Lipidization promotes the binding of peptides to albumin through conjugating fatty acid to the peptide sequence. Binding to human serum albumin (HSA) facilitating peptides being sterically shielded from enzyme degradation and rapid renal filtration due to the large molecular weight of HSA.10 However, lipidization increases the lipophilicity of the peptide and may bring solubility issues. The fatty acid moiety, especially for very long fatty acids, may hamper the peptide–receptor interactions, leading to the decreased bioactivities.11
In our previous research, we have successful combined dimerization and lipidization strategies to discover novel Xenopus GLP-1 analogues with improved biological activities and stabilities.12 Considering the similar structure of Xenopus GLP-1 analogues and native GLP-1, we hypothesized that combined dimerization and lipidization strategies was also suitable applied to native GLP-1 to discover novel potent GLP-1 receptor (GLP-1R) agonists with enhanced metabolic stability. In this article, we prepared novel dimerized GLP-1 (Di-GLP-1) and fatty acid conjugated dimerized GLP-1 (Lip-Di-GLP-1, Fig. 1A). The stability of synthesized analogs and their abilities to activate the GLP-1R in vitro, in vivo hypoglycemic and insulinotropic activities were examined. The gastric emptying, anorectic action, long-acting glucose lowering activities and long-term treatment efficacies of Lip-Di-GLP-1 in normal and diabetic mouse models (db/db) were also explored.
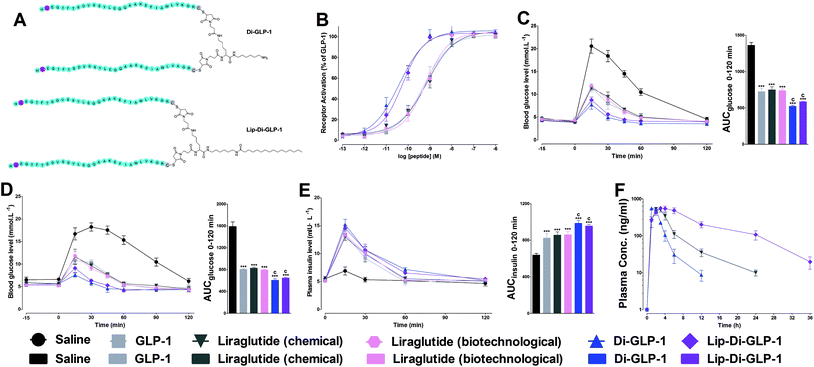 |
| Fig. 1 Structures (A) and biological activity tests of Di-GLP-1 and Lip-Di-GLP-1. (B) Dose–response relationship of GLP-1, Di-GLP-1 and Lip-Di-GLP-1 on GLP-1 receptor. Experiments and performed in triplicate and repeated three times. (C) Acute glucose-lowering effects of GLP-1, Di-GLP-1 and Lip-Di-GLP-1 in Kunming mice and calculated AUCglucose values during 0–120 min. Hypoglycemic and insulinotropic activities of GLP-1, Di-GLP-1 and Lip-Di-GLP-1 were measured by IPGTT in db/db mice. (D) Time–blood glucose and (E) time–plasma insulin in db/db mice and the corresponding AUC values of glucose and insulin. Means ± SD, n = 6. (F) PK profiles of Lip-Di-GLP-1 in Kunming mice. Means ± SD, n = 3. ***P < 0.001 vs. saline, cP < 0.001 vs. GLP-1, liraglutide (chemical) and liraglutide (biotechnological). | |
Results and discussion
Preparation and characterization of Di-GLP-1 and Lip-Di-GLP-1
Dimeric strategy was wildly occurred in nature, to investigate whether this strategy could also employed on GLP-1 to improve the biological activities and stabilities, Di-GLP-1 and Lip-Di-GLP-1 were designed and synthesized as described above. The apparent molecular weights (Mw) of Di-GLP-1 and Lip-Di-GLP-1 were determined by MS. As shown in Fig. S3 and 4, Tables S1 and S2 (see ESI†), their calculated mass-to-charge (m/z) ratios closely matched experimental values.
Biological activity tests of Di-GLP-1 and Lip-Di-GLP-1
The receptor activation potencies of Di-GLP-1 and Lip-Di-GLP-1 were examined in HEK293 cells, which stable expressed high levels of GLP-1R. As shown in Fig. 1B, both Di-GLP-1 (EC50 = 0.039 ± 0.007 nM) and Lip-Di-GLP-1 (EC50 = 0.056 ± 0.018 nM) exhibited higher potency for GLP-1R than native GLP-1 (EC50 = 0.53 ± 0.13 nM), liraglutide (chemical, EC50 = 0.72 ± 0.27 nM) and liraglutide (biotechnological, EC50 = 0.65 ± 0.14 nM).
To investigate whether the higher GLP-1R potencies of Di-GLP-1 and Lip-Di-GLP-1 indicated higher in vivo hypoglycemic effects, we compared their acute glucose-lowering effects in normal Kunming mice by intraperitoneal glucose tolerance test (IPGTT). As shown in Fig. 1C, blood glucose levels in saline treated mice increased rapidly after glucose administration. In consistent with the in vitro biological results, maximum blood glucose levels at 15 min in Di-GLP-1 and Lip-Di-GLP-1 groups were lower than that of in GLP-1, liraglutide (chemical) and liraglutide (biotechnological) groups. The glucose area under the curve (AUC) values further revealed that the hypoglycemic effects of Di-GLP-1 and Lip-Di-GLP-1 were significant better than GLP-1, liraglutide (chemical) and liraglutide (biotechnological) (P < 0.001 and P < 0.001, respectively, Fig. 1C).
The insulinotropic effect of native GLP-1 is key physiologic function that leading insulin secretion in a blood glucose level-dependent manner.13 To examine this function of Di-GLP-1 and Lip-Di-GLP-1, their antidiabetic and insulinotropic activities were examined in db/db mice, a type 2 diabetic model. As shown in Fig. 1D, treatment with Di-GLP-1 and Lip-Di-GLP-1 significantly lower the blood glucose concentrations post the glucose challenge, and the AUC values of glucose showed that the hypoglycemic activities of Di-GLP-1 and Lip-Di-GLP-1 were similar and were significantly better than GLP-1, liraglutide (chemical) and liraglutide (biotechnological) (P < 0.001 and P < 0.001, respectively). Accordingly, Di-GLP-1 and Lip-Di-GLP-1 were found exhibited significantly better insulinotropic activities than GLP-1, liraglutide (chemical) and liraglutide (biotechnological) (P < 0.001 and P < 0.001, respectively, Fig. 1E).
In vitro and in vivo toxicity assays
MTT assay was performed on INS-1 cells as a preliminary evaluation of in vitro toxicity of Di-GLP-1 and Lip-Di-GLP-1. As shown in Fig. 2A and B, no prominent toxicity effects of Di-GLP-1 and Lip-Di-GLP-1 on INS-1 cells were observed under concentrations of 10, 100 and 1000 nM. The in vivo toxicity study was evaluated in db/db mice. Mice were twice daily s.c. injected saline, 500 nmol kg−1 of Di-GLP-1, Lip-Di-GLP-1, liraglutide (chemical) and liraglutide (biotechnological) for 7 days. At the end of the study, four key parameters indicative of liver toxicity (alanine aminotransferase (ALT) and aspartate aminotransferase (AST)) and renal toxicity (blood urea nitrogen (BUN) and serum creatinine (SCr)) were determined by using automatic biochemical analyzer. As shown in Fig. 2C and D, no significant difference was observed in these parameters between control, Di-GLP-1, Lip-Di-GLP-1, liraglutide (chemical) and liraglutide (biotechnological) treated groups, preliminary indicating the safety of Di-GLP-1 and Lip-Di-GLP-1 to the liver and kidney. Since the Lip-Di-GLP-1 and Di-GLP-1 were prepared by chemical synthesis, in the following in vivo biological assessment, the chemical synthesized liraglutide was chosen to further characterise Lip-Di-GLP-1.
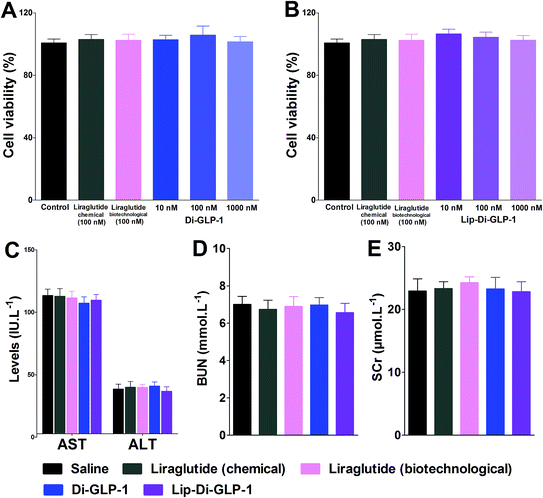 |
| Fig. 2 In vitro toxicity effect of Di-GLP-1 (A) and Lip-Di-GLP-1 (B). Dose–response relationship of Di-GLP-1 and Lip-Di-GLP-1 on INS-1 cells were observed under concentrations of 10, 100 and 1000 nM. Experiments and performed in triplicate and repeated three times. Preliminary in vivo toxicity study of Di-GLP-1 and Lip-Di-GLP-1 was evaluated in db/db mice. ALT and AST (C) were key parameters indicative of liver toxicity, BUN (D) and SCr (E) were key parameters indicative of renal toxicity. These data were determined using automatic biochemical analyzer. Means ± SD, n = 6. | |
PK profiles of Di-GLP-1 and Lip-Di-GLP-1
The PK properties of Di-GLP-1 and Lip-Di-GLP-1 were measured in Kunming mice and compared with liraglutide (chemical). As shown in Fig. 1F and Table S3 (see ESI†), the t1/2 of Lip-Di-GLP-1 (7.0 ± 0.7 h) was 1.8 times higher than liraglutide (3.9 ± 0.3 h), and its AUCinf value (7456.6 ± 564.4) was 2.8 times greater than that of liraglutide (2693.6 ± 175.5). However, both the t1/2 (1.8 ± 0.2 h) and AUCinf value (1637.3 ± 39.3) of Di-GLP-1 was lower than that in liraglutide (chemical) and Lip-Di-GLP-1 groups.
Hypoglycemic duration test of Lip-Di-GLP-1 in non-fasted db/db mice
In view of the significant improved PK behaviors of Lip-Di-GLP-1, a non-fasting mice model with high glucose was used to further test the duration of hypoglycemic abilities of Lip-Di-GLP-1. Since our goal was to develop GLP-1 derivatives with long-acting effects, Di-GLP-1 didn't exhibit favorable long-acting biological activities and thus wasn't studied in this model and the following chronic experiments. As shown in Fig. 3A, the nadir of glucose level in Lip-Di-GLP-1 group was lower than that in synthesized liraglutide group, and mice treated with Lip-Di-GLP-1 exhibited significant delayed rebound time from the nadir to the high glucose level (>18 mmol L−1) compared with mice treated with synthesized liraglutide, indicated the longer duration of hypoglycemic of Lip-Di-GLP-1 than synthesized liraglutide. Next, we performed a dose–response study to examine the dose dependency of the hypoglycemic effect of Lip-Di-GLP-1.
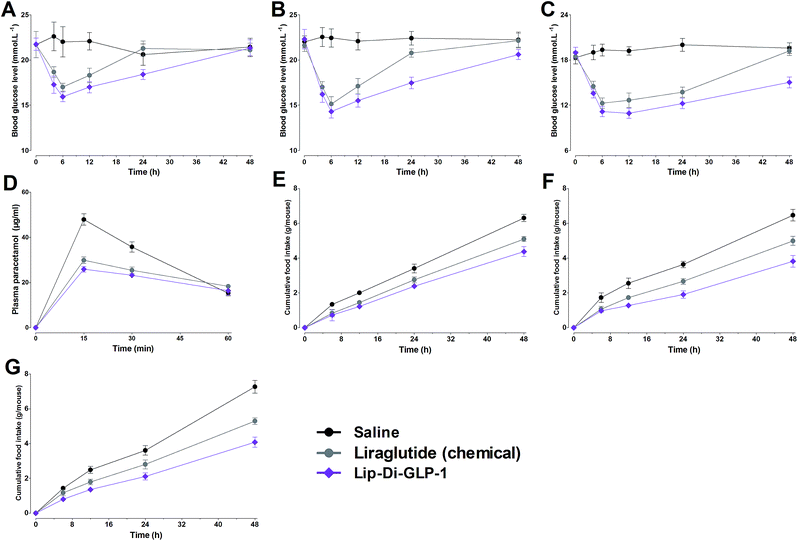 |
| Fig. 3 Dose–response studies for glucose lowering and anorectic effects of Lip-Di-GLP-1 and synthesized liraglutide in db/db mice at doses of 10 nmol kg−1 (A and E), 25 nmol kg−1 (B and F), and 100 nmol kg−1 (C and G). (D) Paracetamol absorption test of Lip-Di-GLP-1 and synthesized liraglutide in Kunming mice. Means ± SD, n = 6. | |
As shown in Fig. 3B and C, accompanied by the rise in the dose of Lip-Di-GLP-1, the rebound time from the nadir to the high glucose level significantly increased, indicated Lip-Di-GLP-1 had a dose-dependent long-acting glucose-lowering efficacy. Compared with Lip-Di-GLP-1, synthesized liraglutide showed a less potent and shorter duration of hypoglycemic activities.
Gastric emptying and feeding studies
Gastric emptying in Kunming mice was measured using paracetamol absorption test. As shown in Fig. 3D, Lip-Di-GLP-1 significantly reduced the concentrations of paracetamol in plasma, suggesting a potent inhibition of gastric emptying. We next assessed the acute effects of Lip-Di-GLP-1 on food intake. The results revealed that Lip-Di-GLP-1 significantly reduced food intake in a dose-dependent manner, and the anorectic actions of Lip-Di-GLP-1 were better than synthesized liraglutide (Fig. 3E–G).
Beneficial effects of chronic treatment of Lip-Di-GLP-1 in db/db mice
To evaluate the chronic treatment effects of Lip-Di-GLP-1, 8 week-old db/db mice were once-daily treated with Lip-Di-GLP-1 or twice-daily treated with synthesized liraglutide for 6 weeks. Compared to the control group at day 45, treatment with Lip-Di-GLP-1 prevented the increasing of reliable glucose marker HbA1c and led to a significantly lower value of ∼3.9%, better than synthesized liraglutide (∼2.3%, Fig. 4A and B). Furthermore, chronic Lip-Di-GLP-1 treatment resulted in significant improvements in food intake, body weight, non-fasting blood glucose and fasting insulin levels as compared with control, and to a greater extent than synthesized liraglutide (Fig. 4C–F). In addition, the IPGTT results revealed that Lip-Di-GLP-1 potently normalized glucose tolerance after 6 weeks treatment (Fig. 4G). The high IOD values of insulin in Lip-Di-GLP-1 group in histological analysis further proved that the improved glucose tolerance induced by Lip-Di-GLP-1 was attributed to its protective effect on pancreas islets (Fig. 5). Significantly, the AST, ALT, Scr and BUN values in Lip-Di-GLP-1 group after the six weeks' treatment were similar to that observed in saline and liraglutide groups, indicating the low toxicities of Lip-Di-GLP-1 on kidney and liver (Fig. 6, more details referring to the ESI†).
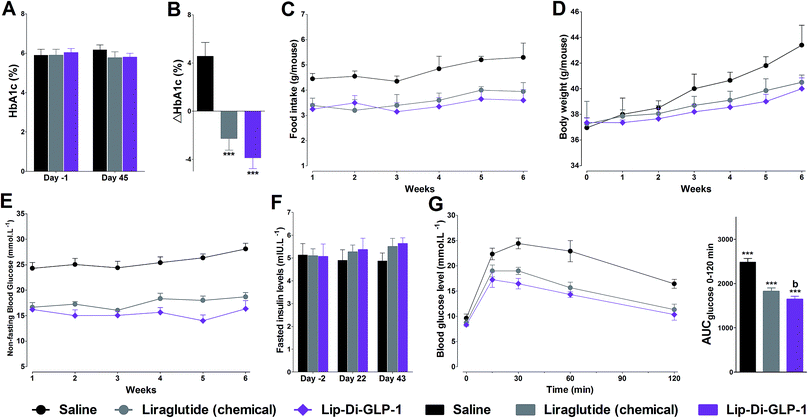 |
| Fig. 4 Chronic treatment effects of Lip-Di-GLP-1 on HbA1c (A and B), food intake (C), body weight (D), blood glucose (E) and fasted insulin (F) in db/db mice. (G) IPGTT was performed at the end of the study. Means ± SD, n = 6. ***P < 0.001 vs. saline, bP < 0.01 vs. GLP-1. | |
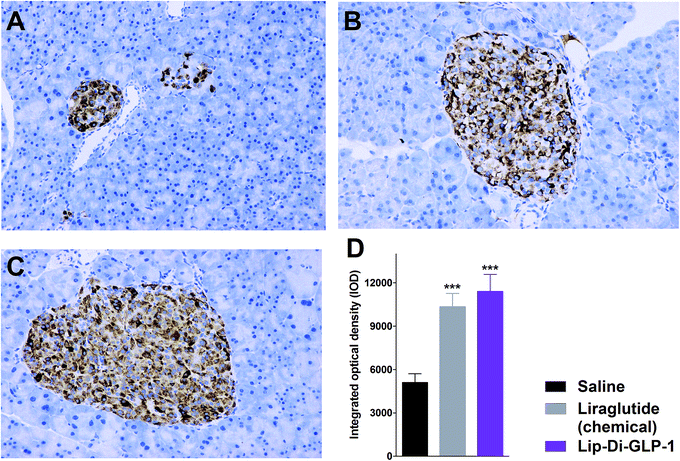 |
| Fig. 5 Treatment effects of Lip-Di-GLP-1 and synthesized liraglutide on pancreatic islets in db/db mice. Representative images of insulin staining in saline (A), liraglutide (B) and Lip-Di-GLP-1 (C) groups. (D) IOD values of insulin in histological analysis. Means ± SD, n = 6. ***P < 0.001 vs. saline. | |
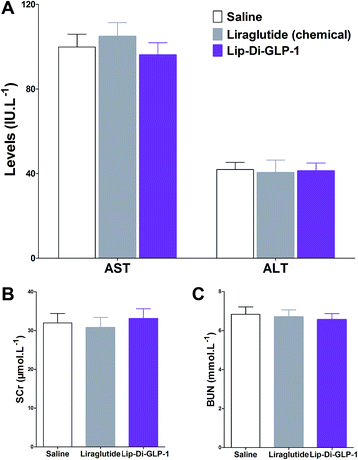 |
| Fig. 6 Biochemical analysis results of synthesized liraglutide and Lip-Di-GLP-1 on serum biomarkers of db/db mice after 6 weeks treatment. (A) ALT and AST. (B) SCr. (C) BUN. Means ± SD, n = 6. | |
Discussion
In the present study, we combined dimerization and lipidization strategies as a novel means to develop efficient GLP-1-based anti-diabetics with enhanced and prolonged hypoglycemic efficacies. We produced high GLP-1R activation potency, long-acting GLP-1 analogues, that is, Di-GLP-1 (dimerized GLP-1) and Lip-Di-GLP-1 (lipidated Di-GLP-1). Firstly, the Ala8 in GLP-1 was replaced with Gly to avoid DPP-IV degradation, and an additional Cys was introduced to the C-terminal of GLP-1, and Gly8-Cys31-GLP-1 was firstly constructed. Then Di-GLP-1 and Lip-Di-GLP-1 were successfully obtained using cysteine–maleimide specific coupling reactions using Gly8-Cys31-GLP-1, bis-maleimide amine, and activated palmitic acid.
Dimerization was viewed as a useful tool to boost ligand–receptor interaction, and peptide drugs were often found possess enhanced binding affinities and higher therapeutic potencies than their monomers.14 We found that Di-GLP-1 had higher GLP-1R activation potency than GLP-1, and the potency of Lip-Di-GLP-1 was only slightly reduced by the presence of palmitic acid, as compared with Di-GLP-1. The conjugation position of fatty acid in peptide has significant impact on potency, and the negligible reductions observed in receptor activation potency for Lip-Di-GLP-1 indicated that using bis-maleimide amine backbone and C-terminal fatty acid conjugations prevented the activity reduction caused by lipidation.
In vivo IPGTT results revealed that the antihypoglycemic efficacies of both Di-GLP-1 and Lip-Di-GLP-1 were significant better than GLP-1. The following assay in db/db mice further revealed the prominent hypoglycemic and insulinotropic activities of Di-GLP-1 and Lip-Di-GLP-1. Significantly, consistent with GLP-1-dependent mechanism, the decreased blood glucose concentrations in Di-GLP-1 and Lip-Di-GLP-1 groups were accompanied by the increased plasma insulin levels. The subsequent PK studies revealed that Lip-Di-GLP-1 has 1.8-fold longer t1/2 and 2.8-fold higher AUCinf value than synthesized liraglutide. PK analysis indicated that dimerization and lipidization not only extended stability but also significantly increased drug utilization of Lip-Di-GLP-1. The physical interactions of palmitic acid group in Lip-Di-GLP-1 with serum albumin and the increased Mw (reduce renal clearance rate) leading by dimerization might be the two main reasons for the enhanced PK behaviors of Lip-Di-GLP-1. The improved PK profiles of Lip-Di-GLP-1 were also manifested in hypoglycemic duration studies. The dose–response studies revealed that the antidiabetic durations of Lip-Di-GLP-1 were significant higher than synthesized liraglutide, and could up to ∼36 h (100 nmol kg−1) in db/db mice. We can envision that the hypoglycemic durations of Lip-Di-GLP-1 would be longer than 2 days in human, as the much faster metabolic rate in mice than human.
GLP-1R agonists induced weight loss is part attributed to their inhibitory effects on gastric emptying.15 The results in paracetamol absorption test showed that Lip-Di-GLP-1 potently inhibited gastric emptying. In feeding studies, Lip-Di-GLP-1 also potently inhibited food intake, and showed better anorectic effects than synthesized liraglutide, and this might attributed to the longer t1/2 and better gastric emptying inhibitory effect of Lip-Di-GLP-1. Finally, the 6 weeks' treatment of Lip-Di-GLP-1 in db/db mice completely prevented the increase in blood glucose levels and HbA1c, suggesting potent effects on ameliorate hyperglycemic state of db/db mice. The protection of β-cell survival is one of the important characteristics of GLP-1, as such, immunohistochemistry analysis was performed. The significantly increased insulin IOD values in Lip-Di-GLP-1 group revealed the potent protective effects of Lip-Di-GLP-1 on pancreas, and also explained the improved glucose tolerance caused by Lip-Di-GLP-1 treatment. The beneficial effects of Lip-Di-GLP-1 in long-term treatment were in agreement with other GLP-1R agonists by chronic peripherally administered.16 The similar values of indexes for kidney and liver toxicity between Lip-Di-GLP-1, saline and synthesized liraglutide groups indicating the safety of Lip-Di-GLP-1.
Conclusion
Summarizing, we described the design and using of Di-GLP-1 and Lip-Di-GLP-1 as novel GLP-1R agonists. Compared with synthesized liraglutide, Lip-Di-GLP-1 has better pharmacokinetics, prominent greater hypoglycemic durations, and more beneficial effects on chronic treatment. Accordingly, more consistent efforts should be taken to discover beneficial effect and potential of Lip-Di-GLP-1 to serve as a long-acting treatment for T2DM.
Experimental
Materials
Chemical synthesized GLP-1(7-36)-NH2 and liraglutide were purchased from the GL Biochem Ltd. (Shanghai, China). Biotechnological liraglutide was purchased from the KMD Bioscience Ltd. (Tianjing, China). Bis-maleimide amine (Mw = 546.3) was obtained from the Xi'an Ruixi Biological Technology Co., Ltd (Xi'an, China). Mouse insulin ELISA kit and cAMP dynamic kit were purchased from Nanjing Jiancheng Bioengineering Institute (Jiangsu, China) and CIS Bio International (Bedford, USA), respectively. Other reagents, unless otherwise indicated, were purchased from Sigma-Aldrich Co. (St. Louis, MO).
Animals
Normal male Kunming mice (20–28 g) were purchased from Qinglongshan Animal Breeding Farm (Nanjing, China). The reason to use Kunming mice included that they have strong disease resistance and adaptability, high reproductive rate and survival rate, and low price. Thus, Kunming mice were widely used in pharmacology, toxicology and other fields in China. BKS.Cg-Dock7m+/+Leprdb/J (db/db) mice (male, 7–8 weeks) were purchased from Model Animal Research Center of Nanjing University (Nanjing, China). Mice (6 per cage) were housed in groups and maintained in a 12 h light/dark cycle and food and water were provided ad libitum. All mice were acclimatized for 1 week before experiment. All experimental procedures were approved by Jiangsu Normal University ethical committee and performed as humane as possible in accordance with the Guide for the Care and Use of Laboratory Animals published by the National Institutes of Health (revised 2011) and the Laboratory Animal Management Regulations in China.
Preparation of Di-GLP-1 and Lip-Di-GLP-1
Di-GLP-1 was synthesized by using chemoselective Michael additions between thiol and maleimide groups using bis-maleimide amine and the cysteine residue, using a pervious described method.8 In brief, Gly8-Cys31-GLP-1 was synthesized using solid phase synthesis using Fmoc synthesis strategy (Fig. S1, see ESI†) on semi-automatic peptide synthesizer (PSI-200). For Di-GLP-1, Gly8-Cys31-GLP-1 (2 eq.) was reacted with bis-maleimide amine (1 eq.) in methanol containing DIPEA. For Lip-Di-GLP-1, NHS activated palmitic acid was reacted with bis-maleimide amine first, then Gly8-Cys31-GLP-1 (2 eq.) was added and using the same synthesis procedure described above (Fig. S2, see ESI†). The reaction solution was directly injected into preparative reversed-phase HPLC to obtain purified products, and the products were characterized by Bruker MicroTOF Q2 using ESI ionization method.
Receptor activation potencies of Di-GLP-1 and Lip-Di-GLP-1 to HEK293 cells
The GLP-1 receptor activation potencies of Di-GLP-1 and Lip-Di-GLP-1 were evaluated using a pervious described method using HEK293 cells which stably expressing GLP-1 receptor.17 Briefly, cells were cultured in DMEM (Invitrogen) with supplement at 37 °C. Serial dilutions of tested peptides were added to 96-well plates containing HEK293 cells. Following incubation, the HTRF reagents (kit components) were added and the plates were incubated for 60 min. The fluorescence ratio at 665/620 nm (expressed as delta F%) were determined on EnVision (PerkinElmer), and cAMP concentrations were calculated using standard curve by plotting delta F% versus cAMP concentration for every experiment. The data for the test compounds were normalized and represented as the percentage (%) of the maximum response induced by saturating GLP-1. EC50 values were calculated by GraphPad Prism.
Intraperitoneal glucose tolerance test on Kunming mice
IPGTT was performed to evaluate the in vivo antihyperglycemic efficacies of GLP-1, liraglutide (chemical), liraglutide (biotechnological), Di-GLP-1 and Lip-Di-GLP-1. In brief, Kunming mice (n = 6) were fasted overnight, and saline (control), GLP-1, liraglutide (chemical), liraglutide (biotechnological), Di-GLP-1 and Lip-Di-GLP-1 (25 nmol kg−1) were injected i.p. 15 min prior to i.p. glucose challenging (2 g kg−1). Tail blood glucose levels were measured using a handheld glucometer (Sannuo, China) at −15, 0, 15, 30, 45, 60 and 120 min.
IPGTT and insulinotropic assay in db/db mice
db/db mice (7 weeks, n = 6) were fasted overnight to ensure the blood glucose levels below 10 mmol L−1, and then i.p. (15 min prior to glucose challenging) administered of saline, GLP-1, liraglutide (chemical), liraglutide (biotechnological), Di-GLP-1 and Lip-Di-GLP-1 (25 nmol kg−1). Glucose challenging (1 g kg−1, i.p.) was performed at 0 min and blood samples were collected at −15, 0, 15, 30, 45, 60, 90 and 120 min, and glucose levels were determined using the above described method. At 0, 15, 30, 60 and 120 min, approximately 8 drops of blood was collected from the cut of the tip tail and plasma was obtained through centrifuging for 5 min at 4 °C. Plasma insulin levels were determined by insulin ELISA kit.18
Pharmacokinetic (PK) assay
Kunming mice (n = 18 for Di-GLP-1, n = 21 for liraglutide (chemical), n = 24 for Lip-Di-GLP-1) were fasted overnight and then 50 nmol kg−1 of liraglutide (chemical), Di-GLP-1 and Lip-Di-GLP-1 were s.c. administrated. At each predetermined times (1, 2, 3, 4, 6, 12, 24 and 36 h), there mice were sacrificed and ∼200 μL of blood samples were collected into EDTA containing tubes through extracting eyeball. Plasma was obtained using the above described method and immediately frozen until analysis via LC-MS/MS using a previous described method.19 The PK parameters were calculated by BAPP 2.2 (China Pharmaceutical University).
Cell viability assay
Rat pancreatic INS-1 cells were used to determine the in vitro toxicity of Di-GLP-1 and Lip-Di-GLP-1. Cells (∼10
000 cells per well) were seeded in 96-well culture plates and directly treated with liraglutide (chemical, 100 nM), liraglutide (biotechnological, 100 nM), Di-GLP-1 and Lip-Di-GLP-1 (10, 100, 1000 nM) and incubated for 24 h. Then MTT solution (5 mg mL−1) was added and incubated for 5 h. The absorbance at 570 nm was measured using the Thermo multiwall spectrophotometer (Waltham, MA, USA). The absorbance in the control cells was set as 100%, and the absorbance values of the saline, liraglutide (chemical), liraglutide (biotechnological), Di-GLP-1 and Lip-Di-GLP-1 were normalized as the percentage of the control value.20
One week toxicity assay in db/db mice
The one week toxicity test of Di-GLP-1 and Lip-Di-GLP-1 was conducted on db/db mice (n = 3), using a previously described method with some modifications. Mice were divided into five groups, before the testing day each group of mice were fasted overnight, and saline, 500 nmol kg−1 of liraglutide (chemical), liraglutide (biotechnological), and Di-GLP-1 and Lip-Di-GLP-1 were s.c. injected and rodent chow were provided immediately. During the testing period, food and water were provided ad libitum. Tested compound and saline were twice daily administered at 8 AM and 8 PM. At the end of the test, the blood was collected through extracting eyeball (approximately 1 mL) and mice were sacrificed. Blood samples were centrifuged to separate serum. The levels of SCr, AST, BUN, and ALT in serum were determined using Beckman Coulter Automatic Analyzer using commercially available kits.20
Hypoglycemic duration test
The glucose homeostasis effects of Lip-Di-GLP-1 were evaluated using db/db mice under non-fasting condition. Mice were given free access to food and water during the whole experiment. On the experiment day, the non-fasting blood glucose levels of mice were assessed, and mice with blood glucose levels over 15 mmol L−1 were selected for experimentation. Then mice were divided into 9 groups (n = 6, 8 weeks) with matching non-fasting blood glucose levels, and saline, liraglutide, and Lip-Di-GLP-1 (10, 25 and 100 nmol kg−1) were s.c. administrated at 0 h. Blood glucose levels were assessed using the same method described above at 0, 4, 6, 12, 24 and 48 h after dosing.
Gastric emptying study
Kunming mice (n = 6) were fasted for 6 h, and mice were injected (i.p.) with saline, liraglutide and Lip-Di-GLP-1 (25 nmol kg−1) 30 min prior to oral administration of paracetamol (100 mg kg−1, 0 min).21 Tail vein blood (∼50 μL) was collected into heparin containing tubes at 15, 30, and 60 min. Plasma were separated using the same method described above and the concentration of paracetamol were analyzed by LC-MS/MS.
Food intake studies
db/db mice (n = 6, 7 weeks) were individual housed and fasted for 16 h before the food intake studies. Mice were s.c. injected with liraglutide, Lip-Di-GLP-1 (10, 25 and 100 nmol kg−1) and saline. Then premeasured amount of food (standard rodent chow) were placed in the cage immediately and mice were allowed free access to food and water. Food intake was measured at 0, 6, 12, 24 and 48 h.
Repeated injections of Lip-Di-GLP-1 in db/db mice
Eight-week-old db/db mice (n = 6) were housed individually and separated to three groups according to their HbA1c levels (%) which measured by DCA2000 chemistry analyzer (Bayer, USA) through collect blood samples from the tail. According to the PK profiles, saline (control) and liraglutide (25 nmol kg−1) were twice daily s.c. administered, and Lip-Di-GLP-1 (25 nmol kg−1) was once daily s.c. administered over 6 weeks. Food intake, body weight and blood glucose levels (non-fasting state) were recorded weekly. Fasted insulin levels were measured at days – 2, 22 and 43 using the same method as described above. One IPGTT was performed after 6 weeks treatment. Mice were fasted overnight (16 h), followed by 1 g kg−1 glucose challenging (i.p.) at 0 min. Blood glucose concentrations were measured at 0, 15, 30, 60 and 120 min. At the end of the study, mice were sacrificed and blood samples were collected. Blood samples were analyzed for HbA1c (%). Furthermore, several key indicators in serum, including SCr, AST, BUN and ALT were determined using Beckman Coulter Automatic Analyzer using commercially available kits. After collect the blood, the pancreases were isolated and insulin immunostaining was carried out using a previously described method.22 The integrated optical density (IOD) values of insulin were recorded using Image-pro plus software (version 6.0, Media Cybernetics Inc., USA).
Statistical analysis
Data are expressed as means ± SD. All pharmacology data analyses were conducted by GraphPad (version 5.0) unless indicated otherwise. Statistical significances between multiple groups were determined using one-way ANOVA with post hoc Tukey's tests. P values < 0.05 were considered statistically significant.
Conflicts of interest
There are no conflicts to declare.
Acknowledgements
This work is supported by the National Natural Science Foundation of China (Grant Nos. 81602964 and 81703346), the Natural Science Foundation of Jiangsu Province (Grant No. BK20150243), the Basic Public Welfare Research Project of Zhejiang Province (Grant No. LGD19H070002), the Science and Technology Projects of Jiaxing (No. 2017AY33074), Zhejiang Provincial Xinmiao Talent Plan (No. 2018R417025) and the Key Project of Jiaxing University Students' Research Training Program (No. 85178550).
Notes and references
- M. A. Nauck, I. Vardarli, C. F. Deacon, J. J. Holst and J. J. Meier, Diabetologia, 2011, 54, 10–18 CrossRef CAS PubMed.
- J. J. Meier, Nat. Rev. Endocrinol., 2012, 8, 728 CrossRef CAS PubMed.
- C. Li, Y. Huan, N. Shen, L. Ji, S. Sun, S. Liu, Q. Liu, L. Gao, F. Tan, Y. Wang and Z. Shen, Biochem. Biophys. Res. Commun., 2010, 400, 563–568 CrossRef CAS PubMed.
- J. J. Meier and M. A. Nauck, Diabetes/Metab. Rev., 2005, 21, 91–117 CrossRef CAS PubMed.
- C. Li, M. Yang, X. Wang, H. Zhang, C. Yao, S. Sun, Q. Liu, H. Pan, S. Liu, Y. Huan, S. Li, J. Cao, X. Wang, Y. Guo, N. Guo, S. Jing, C. Zhang and Z. Shen, Biochem. Pharmacol., 2018, 150, 46–53 CrossRef CAS PubMed.
- B. Manandhar and J.-M. Ahn, J. Med. Chem., 2015, 58, 1020–1037 CrossRef CAS PubMed.
- J. H. Griffin, M. S. Linsell, M. B. Nodwell, Q. Chen, J. L. Pace, K. L. Quast, K. M. Krause, L. Farrington, T. X. Wu, D. L. Higgins, T. E. Jenkins, B. G. Christensen and J. K. Judice, J. Am. Chem. Soc., 2003, 125, 6517–6531 CrossRef CAS PubMed.
- T. H. Kim, H. H. Jiang, S. Lee, Y. S. Youn, C. W. Park, Y. Byun, X. Chen and K. C. Lee, Bioconjugate Chem., 2011, 22, 625–632 CrossRef CAS PubMed.
- E. M. Bech, S. L. Pedersen and K. J. Jensen, ACS Med. Chem. Lett., 2018, 9, 577–580 CrossRef CAS PubMed.
- J. Lau, P. Bloch, L. Schäffer, I. Pettersson, J. Spetzler, J. Kofoed, K. Madsen, L. B. Knudsen, J. McGuire, D. B. Steensgaard, H. M. Strauss, D. X. Gram, S. M. Knudsen, F. S. Nielsen, P. Thygesen, S. Reedtz-Runge and T. Kruse, J. Med. Chem., 2015, 58, 7370–7380 CrossRef CAS PubMed.
- S. B. van Witteloostuijn, K. Mannerstedt, P. Wismann, E. M. Bech, M. B. Thygesen, N. Vrang, J. Jelsing, K. J. Jensen and S. L. Pedersen, Mol. Pharm., 2017, 14, 193–205 CrossRef CAS PubMed.
- J. Han, F. Zhou, Y. Fei, X. Chen, J. Fu and H. Qian, Bioconjugate Chem., 2018, 29, 390–402 CrossRef CAS PubMed.
- S. T. Buckley, T. A. Bækdal, A. Vegge, S. J. Maarbjerg, C. Pyke, J. Ahnfelt-Rønne, K. G. Madsen, S. G. Schéele, T. Alanentalo, R. K. Kirk, B. L. Pedersen, R. B. Skyggebjerg, A. J. Benie, H. M. Strauss, P.-O. Wahlund, S. Bjerregaard, E. Farkas, C. Fekete, F. L. Søndergaard, J. Borregaard, M.-L. Hartoft-Nielsen and L. B. Knudsen, Sci. Transl. Med., 2018, 10, eaar7047 CrossRef PubMed.
- Y. Pan, S. Shi, X. Lao, J. Zhang, S. Tan, Z. Wu and J. Huang, Peptides, 2017, 88, 46–54 CrossRef CAS PubMed.
- K. Raun, P. von Voss, C. F. Gotfredsen, V. Golozoubova, B. Rolin and L. B. Knudsen, Diabetes, 2007, 56, 8 CrossRef CAS PubMed.
- L. L. Baggio and D. J. Drucker, Gastroenterology, 2007, 132, 2131–2157 CrossRef CAS PubMed.
- J. Han, Y. Wang, Q. Meng, G. Li, F. Huang, S. Wu, Y. Fei, F. Zhou and J. Fu, Eur. J. Med. Chem., 2017, 132, 81–89 CrossRef CAS PubMed.
- J. Han, Y. Fei, F. Zhou, X. Chen, Y. Zhang, L. Liu and J. Fu, Br. J. Pharmacol., 2018, 175, 544–557 CrossRef CAS PubMed.
- J. Han, Y. Huang, X. Chen, F. Zhou, Y. Fei and J. Fu, Eur. J. Med. Chem., 2018, 157, 177–187 CrossRef CAS PubMed.
- J. Han, Y. Huang, X. Chen, F. Zhou, Y. Fei and J. Fu, Eur. J. Pharm. Sci., 2018, 123, 111–123 CrossRef CAS PubMed.
- A. Maida, J. A. Lovshin, L. L. Baggio and D. J. Drucker, Endocrinology, 2008, 149, 5670–5678 CrossRef CAS PubMed.
- J. Han, X. Chen, Y. Wang, Y. Fei, F. Zhou, Y. Zhang, L. Liu, P. Si and J. Fu, Biochem. Pharmacol., 2017, 142, 155–167 CrossRef CAS PubMed.
Footnotes |
† Electronic supplementary information (ESI) available. See DOI: 10.1039/c9ra00833k |
‡ Joint first author. |
|
This journal is © The Royal Society of Chemistry 2019 |