DOI:
10.1039/C8RA10646K
(Paper)
RSC Adv., 2019,
9, 8222-8229
Synergetic effect of Na-doping and carbon coating on the electrochemical performances of Li3−xNaxV2(PO4)3/C as cathode for lithium-ion batteries
Received
30th December 2018
, Accepted 28th February 2019
First published on 12th March 2019
Abstract
Carbon coated Li3−xNaxV2(PO4)3/C (x = 0.04, 0.06, 0.10, 0.12, 0.18) cathode materials for lithium-ion batteries were synthesized via a simple carbothermal reduction reaction route using methyl orange as the reducing agent, which also acted as the Na and carbon sources. The influence of various Na-doping levels on the structure and electrochemical performance of the Li3−xNaxV2(PO4)3/C composites was investigated. The valence state of vanadium, the form of residual carbon and the overall morphology of the Li2.90Na0.10V2(PO4)3/C, which showed the highest initial specific discharge capacity of 128 mA h g−1 at the current density of 0.1C (1C = 132 mA g−1) among this series of composites, were further examined by X-ray photoelectron spectroscopy, Raman spectroscopy, scanning electron microscopy and high-resolution transmission electron microscopy, respectively. The results indicated that a well crystallized structure of Na-doped Li2.90Na0.10V2(PO4)3 coated by a carbon matrix is obtained. In the further electrochemical measurements, the Li2.90Na0.10V2(PO4)3/C cathode material shows superior discharge capacities of 124, 118, 113, 106 and 98 mA h g−1 at 0.3, 0.5, 1, 2 and 5C, respectively. High capacity retention of 97% was obtained after 1100 cycles in long-term cyclic performance tests at 5C. The reason for such a promising electrochemical performance of the as-prepared Li2.90Na0.10V2(PO4)3/C has also been explored, which revealed that the synergetic effect of the Na-doping and carbon coating provide enlarged Li+ diffusion channels and the increased electronic conductivity.
1. Introduction
For new large-scale applications such as electric vehicles (EVs), hybrid electric vehicles (HEVs), and power backup, the rechargeable lithium-ion batteries (LIBs) should offer both long-term cyclic stability and high rate capability. Novel cathode materials based on metallic lithium phosphates such as LiMPO4 (M = Fe, Co, Ni, Mn)1–5 and Li3M2(PO4)3 (M = Fe, V)6–13 are promising cathodes for large-scale LIBs due to their superior thermal stability and cyclic performance. Recently, monocline Li3V2(PO4)3 cathode materials have attracted particular interest due to their unique advantages, such as high operation voltage, theoretical specific capacity and ion mobility as well as excellent thermal stability.7–11 However, monocline Li3V2(PO4)3 suffers from the intrinsic disadvantage of poor electronic conductivity (ca. 2.3 × 10−8 S cm−1 at 300 K),11 which limits its applications in large-scale energy storage devices.
To address the aforementioned challenges, different methods, such as metal ion doping10,14–19 and carbon coating20–28 have been adopted. Among them, carbon which can be in situ formed by the decomposition of organic precursor serving as a surface coating of the particles can suppress the growth of grain to reduce the particle size and enhance the electronic conductivity of Li3V2(PO4)3.19,22,23,25–27 Based on this, the carbon plays an irreplaceable role in improving rate capability and cyclic retention of Li3V2(PO4)3 cathode materials. On the other hand, various metallic cations are chosen to substitute the V-site or Li-site in Li3V2(PO4)3 phase.29–34 In the case of V-site substitution, although the bulk electronic conductivity of Li3V2−yMy(PO4)3 is enhanced, the potential plateaus become more slopping because of the different electrochemical activities between V3+ and other metallic cations (Ti4+, Zr4+, Cr3+, Mg2+, Co2+, et. al.).17–19 Compared with the V-site doping, the Li-site doping of Li3V2(PO4)3 can not only maintain the potential plateaus during cycling, but also increase the bulk electronic conductivity and enlarge the diffusion channel of Li+. For examples, the rate capability and cyclic retention of Na-doped Li3−xNaxV2(PO4)3 cathode materials are obviously enhanced.32–34 Nevertheless, only limited cyclic performance (<100 cycles) under moderate rates (≤2C) were reported32–34 In addition, sol–gel method and rheological phase reaction method were the common methods chosen to prepare Na-doped Li3V2(PO4)3,32–34 however, these methods are not suitable for large-scale production compared to the solid-state reaction method because of their complex manufacturing process and time consuming. Moreover, as the doping amount of Na in Li3V2(PO4)3 required a low level, the commonly used inorganic Na sources of Na2CO3,32 NaNO3,33 Na3PO4 (ref. 34) in the synthesis of Na-doped Li3V2(PO4)3 are hardly to achieve homogeneously Na distributed Na-doped Li3V2(PO4)3 via solid-state reaction method as only a little volume of these Na sources is needed due to the large bulk density of inorganic compounds (V = m/ρ, m is the needed mass of Na source, ρ is the bulk density of Na source, and V is the needed volume of Na source).
Herein, a series of Na-doped Li3−xNaxV2(PO4)3/C (x = 0.04, 0.06, 0.10, 0.12, 0.18) cathode materials with carbon coating were synthesized via a simple carbothermal reduction reaction (CTR) synthetic strategy using methyl orange as both reduction agent and Na and carbon sources. Among them, Li2.90Na0.10V2(PO4)3/C composite shows superior physical properties on crystalline structure, carbon matrix, and electronic conductivity which help enhancing its electrochemical performances. The Li2.90Na0.10V2(PO4)3/C composite provides a long-term cyclic stability. Capacity retention of 97% is obtained after 1100 cycles at the current density of 5C. To the best of our knowledge, long-term cyclic performance over 500 cycles especially at 5C of Na-doped Li3−xNaxV2(PO4)3/C cathode is not reported previously.
2. Experimental
Carbon coated Li3−xNaxV2(PO4)3/C (x = 0.04, 0.06, 0.10, 0.12, 0.18) composites with different amounts of Na-doping were synthesized by a solid-state reaction route. Typically, methyl orange was used as both reduction agent and Na and carbon sources, in which LiH2PO4 (AR, 99.9%) and V2O5 (AR, 99.9%) were used to synthesized original Li3NaV2(PO4)3. Stoichiometric raw materials based on the chemical formula of Li3NaV2(PO4)3 were mixed in ethanol and ball milled for 5 h by using zirconia balls. Then, the mixed slurry was dried in an oven at 60 °C for 12 h followed by an annealing process at 300 °C for 1 h under nitrogen. After being calcined at 850 °C for 8 h, Li3−xNaxV2(PO4)3/C sample were obtained.
The crystal structure of the Li3−xNaxV2(PO4)3/C composites was detected by X-ray diffraction (XRD, Rigaku P/max 2200VPC) using Cu Kα radiation. The chemical valence state of V in the Li3−xNaxV2(PO4)3/C composite was determined by X-ray photoelectron spectroscopy (XPS) using Thermo ESCALAB 250 spectrometer with monochromatic Al Kα radiation. Resonant Raman scattering spectra were recorded at room temperature with a JY HR-800 Lab Ram confocal Raman microscope with an excitation wavelength of 325 nm. The microstructure of the composites was observed using scanning electron microscopy (SEM, XL 30 ESEM-FEG, FEI Company) and high resolution transmission electron microscopy (HRTEM, JEM-2010). For the measurement of electronic conductivity, powder sample was pressed in disks of 15 mm in diameter and 2 mm in thickness using a four-point probe meter (SDY-5, Guangzhou). The carbon contents in Li3−xNaxV2(PO4)3/C composites were determined by the VarioEL III (elementar, Germany) element analyzer.
The electrochemical performances of the Li3−xNaxV2(PO4)3/C composites as cathode for LIBs were evaluated using a coin-type 2025 cell using metallic lithium as anode, in which the electrodes were produced by spreading a slurry mixed by 84 wt% active materials (Li3−xNaxV2(PO4)3/C), 8 wt% carbon black, 8 wt% polyvinylidene fluoride (PVDF) and N-methylpyrrolidone (NMP) as solvent onto an aluminum foil and dried in a vacuum oven at 120 °C for 12 h. The loading of Li3−xNaxV2(PO4)3/C in each electrode was 2–3 mg cm−2. The cells were assembled in a glove box filled with high-purity argon using anode of Li metal and separator of micro-porous polypropylene. 1 M LiPF6 in a mixture of ethylene carbonate (EC) and dimethyl carbonate (DMC) (1
:
1 by volume) was used as electrolyte. The cells were galvanostatically charged and discharged between 3.0 and 4.2 V at room temperature (25 °C) on an electrochemical test instrument (CT2001A, Wuhan Land Electronic Co. Ltd., China). The capacity of the prepared cathode is calculated according the discharge capacity of the half-coin cells divided by the loading of active material.
3. Results and discussion
3.1 Structural characterization
The XRD patterns of the Li3−xNaxV2(PO4)3/C (x = 0.04, 0.06, 0.10, 0.12, 0.18) composites are shown in Fig. 1, in which the sharp diffraction peaks indicate a good crystallinity for all the samples. The main diffraction peaks are well indexed to monoclinic structure with the space group of P21/n of Li3V2(PO4)3 (JCPDS no. 80-1515), which are consistent with the previous reports.35,36 The lattice parameters of Li Li3−xNaxV2(PO4)3/C are a = 8.4935 Å, b = 11.8776 Å, c = 8.7463 Å, and V = 882.64 Å3. The change of the lattice parameters can be the clue illustrating the doping of Na ions into the L3V2(PO4)3 lattice. Besides, there is not any extra reflections observed for the Li3−xNaxV2(PO4)3/C with x ≥ 0.10, which indicated that Na enter into the structure of Li3−xNaxV2(PO4)3/C rather than forming impurities.32 However, the impurity of Li3PO4 corresponding the diffraction peak at 22.7° (denoted by “+”) can be observed in Li3−xNaxV2(PO4)3/C with x = 0.04 and 0.06. The carbon-related diffraction peaks especially attributed to crystalline carbon are not detected in the XRD patterns, which indicates that the carbon generated from methyl orange is amorphous in a low level. Notably, high purity of Li3−xNaxV2(PO4)3/C composites can be synthesized when x is higher than 0.06.
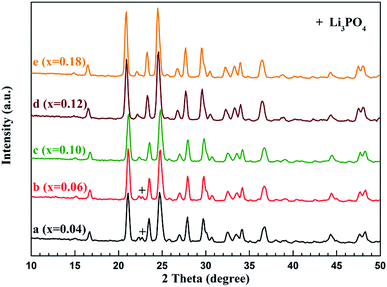 |
| Fig. 1 XRD patterns of the Li3−xNaxV2(PO4)3/C composites. Curves a, b, c, d and e corresponding to x = 0.04, 0.06, 0.10, 0.12 and 0.18, respectively. | |
Fig. 2 shows the initial galvanostatic charge–discharge curves of the Li3−xNaxV2(PO4)3/C as cathode for LIBs at the current density of 0.3C (1C = 132 mA g−1) in the voltage range of 3.0 to 4.2 V. Except for the Li2.82Na0.18V2(PO4)3/C, the samples exhibit three pairs of discharge–charge flat plateaus, corresponding a multi-phase transition processes occurred during the electrochemical reactions. For the Li2.82Na0.18V2(PO4)3/C composite, there is one additional charge–discharge potential plateau located at about 3.7 V (as compared to the curves of “c” and “e” in details in the inset in Fig. 2), the additional one is the characteristic charge–discharge potential plateau of the Li3V2(PO4)3 and Li2NaV2(PO4)3 with rhombohedral structure.37,38 It has been reported that the specific capacity of the rhombohedral Li3V2(PO4)3 or Li2NaV2(PO4)3 is lower than that of the monoclinic Li3V2(PO4)3, although the rhombohedral structure can supply fast ion-mobility. Therefore, the presence of rhombohedral Li3V2(PO4)3 or Li2NaV2(PO4)3 is not beneficial to the capacity output of the Na-doped Li3−xNaxV2(PO4)3/C composite. The initial discharge specific capacities for the Na-doped Li3−xNaxV2(PO4)3/C composite are ca. 112, 117, 124, 119, and 114 mA h g−1 for x of 0.04, 0.06, 0.10, 0.12 and 0.18, respectively. Discharge capacity of the composite increases firstly and then decreases with the increase of x, reaching a maximum when x = 0.10. It has been reported that the cell volume of Li3V2(PO4)3 is enlarged after the substitution of Na for Li, as the radius of Na (r = 0.097 nm) is larger than that of Li (r = 0.068 nm), which results in large channel for rapid transfer of Li+.32–34 Methyl orange provides both Na and carbon to the Li3−xNaxV2(PO4)3/C composites. The carbon content for the Li3−xNaxV2(PO4)3/C composite detected by carbon–sulfur analyzer are ca. 1.1, 1.8, 2.2, 3.2, and 4.3 wt% for x of 0.04, 0.06, 0.10, 0.12 and 0.18, respectively. The electrochemical performance of all the Li3−xNaxV2(PO4)3/C composites is better than that of the Li3V2(PO4)3 without Na doping and carbon coating reported previously.36 As reported in literatures,32–34 the rate performance, cyclic ability and the charge transfer property of Li3−xNaxV2(PO4)3 composite are all enhanced after suitable Na-doping because that the Na-doping can not only enlarges the Li+ diffusion channel, but also increases the bulk electronic conductivity. Moreover, in our previous report,36 the Li3V2(PO4)3/C composite exhibits significantly better electrochemical performances than the pristine Li3V2(PO4)3, which is attributed to the drastically increased electronic conductivity of the composite by carbon coating. Therefore, it can be concluded that it is the synergetic effect of Na-doped and carbon coating that causes the excellent charge/discharge performance of the Li2.90Na0.10V2(PO4)3/C composite.
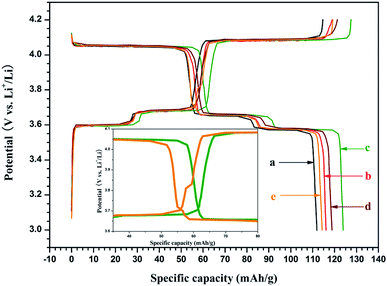 |
| Fig. 2 The initial galvanostatic charge–discharge curves of the Li3−xNaxV2(PO4)3/C composites. Curves a, b, c, d and e corresponding to x = 0.04, 0.06, 0.10, 0.12 and 0.18, respectively. | |
Fig. 3A shows the V 2p XPS core level of the Li2.90Na0.10V2(PO4)3/C composite. The V 2p core level fits to a single peak with a binding energy of 517.3 eV, matching well with the data of V3+ in Li3V2(PO4)3 reported in literature (517.2 eV).10,36 No valance state other than V3+ was detected in the Li2.90Na0.10V2(PO4)3/C composite, indicating that the constant state of V in the composite is not changed by the Na-doping. The Raman spectrum of the Li2.90Na0.10V2(PO4)3/C composite is shown in Fig. 3B, in which the peaks in the range of 800–1200 cm−1 correspond to the stretching modes of the (PO4)3−.39 The peaks in the range of 1550–1660 and 1250–1450 cm−1 are assigned to the graphite band (G-band, sp2 character carbon) and disorder-induced phonon mode (D-band, sp3 character carbon), respectively. Meanwhile, the ID/IG ratio of the Li2.90Na0.10V2(PO4)3/C composite calculated from Fig. 3B is ca. 0.66, demonstrating that the quality of sp2 character carbon is much larger than sp3 character one in the coating carbon. Thus, the coating carbon with much sp2 characters are beneficial to the electronic conductivity and facilitate the diffusion of Li+. The ID/IG ratio of this coating carbon has the similar value as that of the residual carbon which is formation from the frequently used organic carbon precursors, such as PEG, PVA and so on.35,36 It can be concluded from the present study that the methyl orange used as both Na and carbon sources can form the high-quality carbon matrix which help improving the electron conductivity of the materials without any changes in both the valance state of V and the structure of Li2.90Na0.10V2(PO4)3/C composite. Recently, Li3−xNaxV2(PO4)3/C composites can be successfully synthesized after the Na-doping by using different organic carbon precursors and inorganic Na sources,32–34 but these composites can be only synthesized via sol–gel method and rheological phase reaction method attributed to a little volume of Na sources used, which is caused by a low Na-doping level and large bulk density of inorganic Na sources. Based on this, in our work the Li2.90Na0.10V2(PO4)3/C composite can be successful prepared via a simple solid-state reaction synthetic route when the methyl orange was chosen as both Na and carbon sources, which is due to the low bulk density of methyl orange compared to that of inorganic salts, and the synthetic route reported here must be the first choice for commercialized produced.
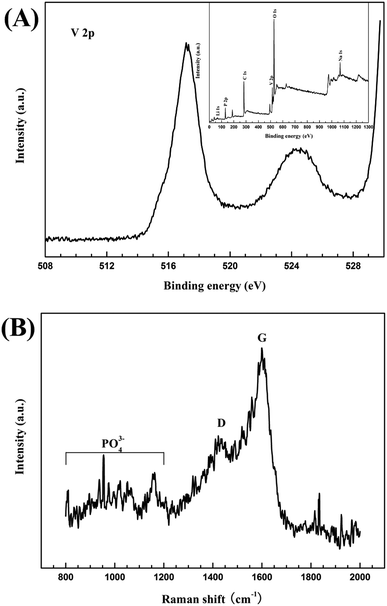 |
| Fig. 3 XPS spectrum of V 2p (A) and Raman spectrum (B) of the Li2.90Na0.10V2(PO4)3/C composite. | |
The morphologies of the Li2.90Na0.10V2(PO4)3/C composite are shown in Fig. 4, in which the Li2.90Na0.10V2(PO4)3/C composite shows primary particle size of ca. 80–150 nm with partially agglomeration. HRTEM images (Fig. 4D) shows that the Li2.90Na0.10V2(PO4)3 particle is uniformly wrapped by a carbon coating in thickness of ca. 4 nm, which has been reported that the in situ formed carbon coating plays an important role in suppressing the bulk growth of inorganic particles.35,36,39,40 Moreover, the carbon coating can help increasing the electronic connecting of the particles and hence enhancing the electronic conductivity of the composite. The electronic conductivity of the Li2.90Na0.10V2(PO4)3/C composite measured is about 9.7 × 10−2 S cm−1, which is much higher than that of the Li3V2(PO4)3 (2.3 × 10−8 S cm−1) at 300 K.11 It is reported that the morphology and specific surface area of the particles have an important influence on the electrochemical performances of Li3V2(PO4)3, so it is necessary to find suitable optimizing particles size or/and introducing conductive additives.35 As aforementioned that both the highly crystallized structure of Na-doped Li2.90Na0.10V2(PO4)3 and the coating carbon with relatively low ID/IG ratio were achieved by using methyl orange as both Na and carbon sources. Although Li3−xNaxV2(PO4)3/C composites have been prepared with different inorganic Na sources (Na2CO3, NaNO3 and Na3PO4) and organic carbon source precursor (citric acid and PEG200) in the previous studies, however, the synthetic routes, which are the sol–gel method32,33 and rheological phase reaction method,34 are not suitable for large-scale preparation compared to the solid-state reaction method that is used in this study because of their manufacturing process complexity and time consuming.
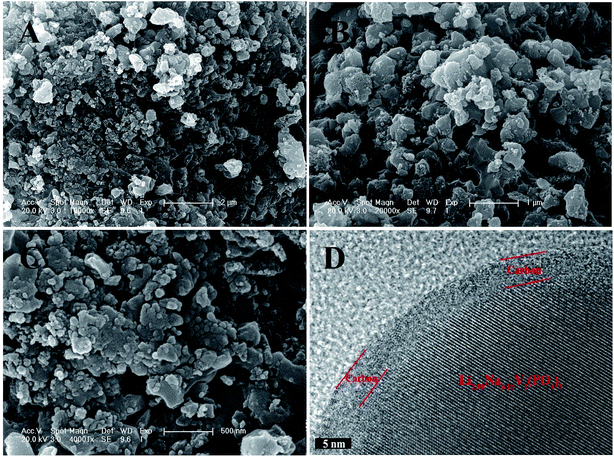 |
| Fig. 4 SEM (A–C) and HRTEM (D) images of the Li2.90Na0.10V2(PO4)3/C composite. | |
3.2 Electrochemical property
Fig. 5A shows the galvanostatic discharge–charge curves of the Li2.90Na0.10V2(PO4)3/C composite at different rates with corresponding capacity and coulombic efficiency listed in Table 1. At a low rate of 0.1C, the electrode shows a high discharge specific capacity of 128 mA h g−1 corresponding to 97% of the theoretical specific capacity (132 mA h g−1) for the reversible (de)intercalation of two lithium ions from Li3V2(PO4)3. The Li2.90Na0.10V2(PO4)3/C composite exhibits excellent rate capability and coulombic efficiency at high rate. At a high rate of 5C, the capacity of the Li2.90Na0.10V2(PO4)3/C composite keeps almost constant within 1100 cycles, as shown in Fig. 5B. The capacity still maintains 95 mA h g−1 after 1100 cycles, retaining approximately 97% of the initial capacity and the coulombic efficiency keeps at 100% during the cycling. Fig. 5C displays the specific charge and discharge curves of the Li2.90Na0.10V2(PO4)3/C composite and the results are in coincident with Fig. 5B. To our knowledge, only limited cyclic performance (<100 cycles) under moderate rates (≤2C) were reported.32,33 The promising electrochemical performance of the Li2.90Na0.10V2(PO4)3/C composite should be attributed to the enlarged Li+ diffusion channel and the improved electronic conductivity resulted from both the Na-doped and carbon coating, as the larger radius of Na+ than that of the Li+, the diffusion channel of Li+ is likely enlarged after the substitution, which enhances the mobility of lithium ions.32–34 Without the doping of Na and coating of carbon, the cycling performance of Li3.0V2(PO4)3 composite at the rate of 5C are poor. The specific capacity of the composite decreases to less than 20 mA h g−1 after 900 cycles. By means of the in situ introduced carbon, not only nano-particles of Li2.90Na0.10V2(PO4)3 are produced, but also the electrical contact between the individual Li2.90Na0.10V2(PO4)3 particles is increased, which are beneficial to the cyclic performance of Li2.90Na0.10V2(PO4)3/C composite. The (de)intercalation ability of Li+ for Li2.90Na0.10V2(PO4)3 is likely enhanced by the Na-doping, which not only enlarges the Li+ diffusion channel, but also enhances the bulk electronic conductivity, and the carbon coating, which also increases the electronic conductivity. In addition, the nano-particles shorten the Li+ diffusion lengths. All the above factors lead to improved electrochemical performances of the Li2.90Na0.10V2(PO4)3/C composite, especially the ultrahigh coulombic efficiency at high rates. To further investigate the structure ability of the Li2.90Na0.10V2(PO4)3/C composite, the XRD patterns and SEM images of the cathode electrodes after 1000 cycles are acquired as displayed in Fig. 6. It is seen that the peak intensities and positions of the cycled electrodes have limited difference of the result of the electrode before cycling. The SEM images after 1000 cycling also indicates the stability of the Li2.90Na0.10V2(PO4)3/C composite.
Table 1 The charge/discharge capacity and coulombic efficiency of the Li2.90Na0.10V2(PO4)3/C composite at different charge/discharge rates
Charge/discharge rate |
0.1C |
0.3C |
0.5C |
1C |
2C |
5C |
The charge capacity (mAh g−1) |
131 |
128 |
121 |
115 |
107 |
99 |
The discharge capacity (mAh g−1) |
128 |
124 |
118 |
113 |
106 |
98 |
Columbic efficiency |
98% |
97% |
97% |
98% |
99% |
99% |
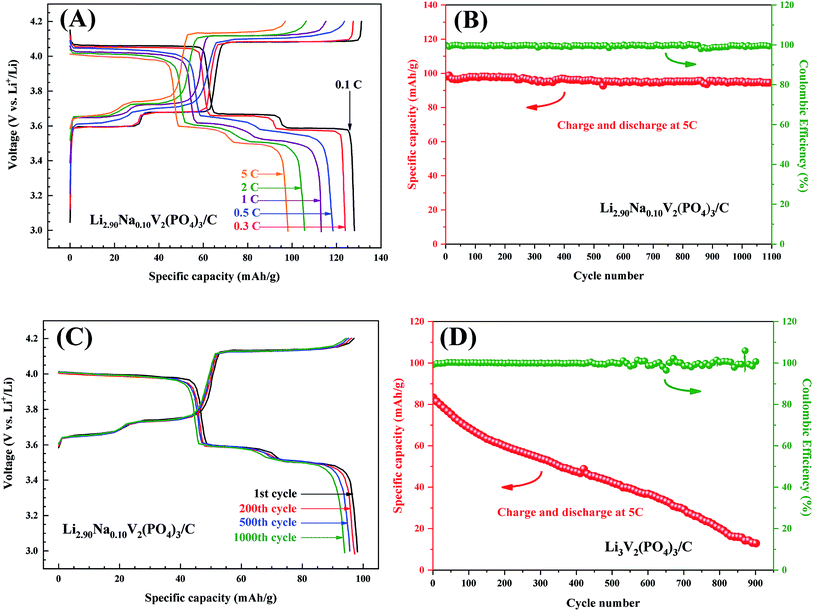 |
| Fig. 5 Initial discharge/charge curves at different rates (A) of the Li2.90Na0.10V2(PO4)3/C composite, the cycling performance at 5C (B) and the discharge/charge curves (C) during cycling of the Li2.90Na0.10V2(PO4)3/C composite, the cycling performance at 5C (D) of Li3.0V2(PO4)3/C composite. | |
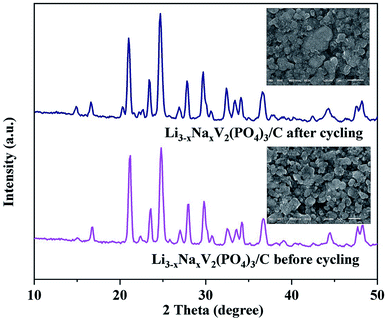 |
| Fig. 6 XRD patterns and SEM images (the inset figures) of the Li2.90Na0.10V2(PO4)3/C composite before and after cycling, respectively. | |
4. Conclusions
Na-doped Li3−xNaxV2(PO4)3/C (x = 0.04, 0.06, 0.10, 0.12, 0.18) used as cathode for lithium-ion batteries were successfully synthesized via a simple carbothermal reduction reaction route by using methyl orange as reduction agent and, Na and carbon sources. Among them, the Li2.90Na0.10V2(PO4)3/C composite with a small amount doping of x = 0.10 exhibits excellent rate capability and long-term cyclic stability, in which a specific capacity of 95 mA h g−1 is obtained at the current density of 5C after 1100 cycles corresponding to the capacity retention of 97%. The promising electrochemical performance of the Li2.90Na0.10V2(PO4)3/C composite is attributed to the synergetic effect of Na-doping and carbon coating, which supplies enlarged the diffusion channel of Li+ and increased electronic conductivity. As the excellent electrochemical performance of Li2.90Na0.10V2(PO4)3/C, and further considering its high safety, it is hopefully that the composite shows a promising candidate as cathode for lithium-ion batteries of hybrid electric vehicles (HEVs) and electric vehicles (EVs) in the future.
Conflicts of interest
There are no conflicts to declare.
Acknowledgements
This work was supported by the Public Projects of Zhejiang Province [No. LGG19E020001], the Opening Foundation of Application Technology Collaborative Innovation Center of Zhejiang Province [No. NZXT2018206], and the Talent Introduction Research Projects of Ningbo Polytechnic [No. RC201802].
References
- J. B. Goodenough, How we made the Li-ion rechargeable battery, Nat. Electron., 2018, 1, 204 CrossRef.
- A. K. Padhi, K. S. Nanjundaswamy and J. B. Goodenough, Phospho-olivines as positive-electrode materials for rechargeable lithium batteries, J. Electrochem. Soc., 1997, 144, 1188–1194 CrossRef CAS.
- A. K. Padhi, K. S. Nanjundaswamy, C. Masquelier, S. Okada and J. B. Goodenough, Effect of structure on the Fe3+/Fe2+ redox couple in iron phosphates, J. Electrochem. Soc., 1997, 144, 1609–1613 CrossRef CAS.
- S. Brutti, J. Manzi, A. De Bonis, D. Di Lecce, F. Vitucci, A. Paolone, F. Trequattrini and S. Panero, Controlled synthesis of LiCoPO4 by a solvo-thermal method at 220 C, Mater. Lett., 2015, 145, 324–327 CrossRef CAS.
- S. Karthickprabhu, G. Hirankumar, A. Maheswaran, C. Sanjeeviraja and R. S. Daries Bella, Structural and conductivity studies on LiNiPO4 synthesized by the polyol method, J. Alloys Compd., 2013, 548, 65–69 CrossRef CAS.
- M. Sato, S. Tajimi, H. Okawa, K. Uematsu and K. Toda, Preparation of iron phosphate cathode material of Li3Fe2(PO4)3 by hydrothermal reaction and thermal decomposition processes, Solid State Ionics, 2002, 152–153, 247–251 CrossRef.
- A. K. Padhi, K. S. Nanjundaswamy, C. Masquelier and J. B. Goodenough, Mapping of transition metal redox energies in phosphates with NASICON structure by lithium intercalation, J. Electrochem. Soc., 1997, 144, 2581–2586 CrossRef CAS.
- S. C. Yin, H. Grondey, P. Strobel, H. Huang and L. F. Nazar, Charge Ordering in Lithium Vanadium Phosphates: Electrode Materials for Lithium-Ion Batteries, J. Am. Chem. Soc., 2003, 125, 326–327 CrossRef CAS PubMed.
- S. C. Yin, H. Grondey, P. Strobel, M. Anne and L. F. Nazar, Electrochemical property: structure relationships in monoclinic Li3-yV2(PO4)3, J. Am. Chem. Soc., 2003, 125, 10402–10411 CrossRef CAS PubMed.
- X. H. Rui, Q. Y. Yan, M. Skyllas-Kazacos and T. M. Lim, Li3V2(PO4)3 cathode materials for lithium-ion batteries: a review, J. Power Sources, 2014, 285, 19–38 CrossRef.
- X. F. Zhang, R. S. Kühnel, H. T. Hu, D. Eder and A. Balducci, Going nano with protic ionic liquids—the synthesis of carbon coated Li3V2(PO4)3 nanoparticles encapsulated in a carbon matrix for high power lithium-ion batteries, Nano Energy, 2015, 12, 207–214 CrossRef CAS.
- Z. Zhang, Y. Han, J. Xu, J. Ma, X. Zhou and J. Bao, Construction of amorphous FePO4 nanosheets with enhanced sodium storage properties, ACS Appl. Energy Mater., 2018, 1, 4395–4402 CrossRef CAS.
- E. L. Gu, S. H. Liu, Z. Z. Zhang, Y. Y. Fang, X. S. Zhou and J. C. Bao, An efficient sodium-ion battery consisting of reduced graphene oxide bonded Na3V2(PO4)(3) in a composite carbon network, J. Alloys Compd., 2018, 767, 131–140 CrossRef CAS.
- L. Chen, B. Yan, Y. F. Xie, S. M. Wang, X. F. Jiang and G. Yang, Preparation and electrochemical properties of Li3V1.8Mn0.2(PO4)3 doped via different Mn sources, J. Power Sources, 2014, 261, 188–197 CrossRef CAS.
- Q. L. Wei, Q. Y. An, D. D. Chen, L. Q. Mai, S. Y. Chen, Y. L. Zhao, K. M. Hercule, L. Xu, A. Minhas-Khan and Q. J. Zhang, One-pot synthesized bicontinuous hierarchical Li3V2(PO4)3/C mesoporous nanowires for high-rate and ultralong-life lithium-ion batteries, Nano Lett., 2014, 14, 1042–1048 CrossRef CAS PubMed.
- L. L. Zhang, Z. Li, X. L. Yang, X. K. Ding, Y. X. Zhou, H. B. Sun, H. C. Tao, L. Y. Xiong and Y. H. Huang, Binder-free Li3V2(PO4)3/C membrane electrode supported on 3D nitrogen-doped carbon fibers for high-performance lithium-ion batteries, Nano Energy, 2017, 34, 111–119 CrossRef CAS.
- Y. X. Yang, W. W. Xu, R. S. Guo, L. Liu, S. S. Wang, D. Xie and Y. Z. Wan, Synthesis and electrochemical properties of Zn-doped, carbon coated lithium vanadium phosphate cathode materials for lithium-ion batteries, J. Power Sources, 2014, 269, 15–23 CrossRef CAS.
- M. M. Ren, M. Z. Yang, W. L. Liu, M. Li, L. W. Su, X. B. Wu and Y. H. Wang, Co-modification of nitrogen-doped graphene and carbon on Li3V2(PO4)3 particles with excellent long-term and high-rate performance for lithium storage, J. Power Sources, 2016, 326, 313–321 CrossRef CAS.
- X. J. Yang, L. Liu and H. R. Jia, Study on structure and electrochemical performance of Tm3+-doped monoclinic Li3V2(PO4)3/C cathode material for lithium-ion batteries, Electrochim. Acta, 2014, 150, 62–67 CrossRef CAS.
- W. F. Mao, Y. B. Fu, H. Zhao, G. Ai, Y. L. Dai, D. C. Meng, X. H. Zhang, D. Y. Qu, G. Liu, V. S. Battaglia and Z. Y. Tang, Rational design and facial synthesis of Li3V2(PO4)3@C nanocomposites using carbon with different dimensions for ultrahigh-rate lithium-ion batteries, ACS Appl. Mater. Interfaces, 2015, 7(22), 12057–12066 CrossRef CAS.
- S. L. Wang, Z. X. Zhang, Z. T. Jiang, A. Deb, L. Yang and S. I. Hirano, Mesoporous Li3V2(PO4)3@CMK-3 nanocomposite cathode material for lithium ion batteries, J. Power Sources, 2014, 253, 294–299 CrossRef CAS.
- S. L. Wang, Z. X. Zhang, A. Deb, C. C. Yang, L. Yang and S. I. Hirano, Nanostructured Li3V2(PO4)3/C composite as high-rate and long-life cathode material for lithium ion batteries, Electrochim. Acta, 2014, 143, 297–304 CrossRef CAS.
- Y. Wu, Z. Y. Tang, X. Y. Guo, C. Q. Du and X. H. Zhang, An alginic acid assisted rheological phase synthesis of carbon coated Li3V2(PO4)3 with high-rate performance, J. Alloys Compd., 2014, 616, 32–41 CrossRef CAS.
- Q. Z. Ou, Y. Tang, Y. J. Zhong, X. D. Guo, B. H. Zhong, H. Liu and M. Z. Chen, Submicrometer porous Li3V2(PO4)3/C composites with high rate electrochemical performance prepared by sol-gel combustion method, Electrochim. Acta, 2014, 137, 489–496 CrossRef CAS.
- Y. Z. Luo, X. Xu, Y. X. Zhang, Y. Q. Pi, Y. L. Zhao, X. C. Tian, Q. Y. An, Q. L. Wei and L. Q. Mai, Hierarchical carbon decorated Li3V2(PO4)3 as a bicontinuous cathode with high-rate capability and broad temperature adaptability, Adv. Energy Mater., 2014, 4, 1400107 CrossRef.
- X. Y. Cao and J. J. Zhang, Rheological phase synthesis and characterization of Li3V2(PO4)3/C composites as cathode materials for lithium ion batteries, Electrochim. Acta, 2014, 129, 305–311 CrossRef CAS.
- W. F. Mao, N. N. Zhang, Z. Y. Tang, Y. Q. Feng and C. X. Ma, High rate capability of Li3V2(PO4)3/C composites prepared via a TPP-assisted carbothermal method and its application in Li3V2(PO4)3||Li4Ti5O12, J. Alloys Compd., 2014, 588, 25–29 CrossRef CAS.
- Y. Cheng, W. Zhou, K. Feng, H. Z. Zhang, X. F. Li and H. M. Zhang, One-pot synthesis of 3D hierarchical porous Li3V2(PO4)(3)/C nanocomposites for high-rate and long-life lithium ion batteries, RSC Adv., 2017, 7, 38415–38423 RSC.
- S. Zhang, Q. Wu, C. Deng, F. L. Liu, M. Zhang, F. L. Meng and H. Gao, Synthesis and characterization of Ti-Mn and Ti-Fe codoped Li3V2(PO4)3 as cathode material for lithium ion batteries, J. Power Sources, 2012, 218, 56–64 CrossRef CAS.
- J. S. Huang, L. Yang, K. Y. Liu and Y. F. Tang, Synthesis and characterization of Li3V(2-2x/3)Mgx(PO4)3/C cathode material for lithium-ion batteries, J. Power Sources, 2010, 195, 5013–5018 CrossRef CAS.
- C. S. Dai, Z. Y. Chen, H. Z. Jin and X. G. Hu, Synthesis and performance of Li3(V1−xMgx)2(PO4)3 cathode materials, J. Power Sources, 2010, 195, 5775–5779 CrossRef CAS.
- Q. Kuang, Y. M. Zhao and Z. Y. Liang, Synthesis and electrochemical properties of Na-doped Li3V2(PO4)3 cathode materials for Li-ion batteries, J. Power Sources, 2011, 196, 10169–10175 CrossRef CAS.
- Q. Q. Chen, X. C. Qiao, Y. B. Wang, T. T. Zhang, C. Peng, W. M. Yin and L. Liu, Electrochemical performance of Li3-xNaxV2(PO4)3/C composite cathode materials for lithium ion batteries, J. Power Sources, 2012, 201, 267–273 CrossRef CAS.
- R. H. Wang, S. H. Xiao, X. H. Li, J. X. Wang, H. J. Guo and F. X. Zhong, Structural and electrochemical performance of Na-doped Li3V2(PO4)3/C cathode materials for lithium-ion batteries via rheological phase reaction, J. Alloys Compd., 2013, 575, 268–272 CrossRef CAS.
- H. T. Tan, L. H. Xu, H. B. Geng, X. H. Rui, C. C. Li and S. M. Huang, Nanostructured Li3V2(PO4)3 cathodes, Small, 2018, 14, 1800567 CrossRef PubMed.
- J. F. Zhang, H. X. Wei, Y. Cao, C. L. Peng and B. Zhang, Hierarchical LiMnPO4·Li3V2(PO4)3/C/rGO nanocomposites as superior-rate and long-life cathodes for lithium ion batteries, J. Alloys Compd., 2018, 769, 332–339 CrossRef CAS.
- Z. Y. Wang, W. He, X. D. Zhang, Y. Z. Yue, G. H. Yang, X. L. Yi, Y. Y. Wang and J. C. Wang, Li2NaV2(PO4)3/hard carbon nanocomposite cathodes for high-performance Li- and Na-ion batteries, ChemElectroChem, 2017, 4(3), 671–678 CrossRef CAS.
- S. Q. Liang, X. X. Cao, Y. P. Wang, Y. Hu, A. Q. Pan and G. Z. Cao, Uniform 8LiFePO4·Li3V2(PO4)3/C nanoflakes for high-performance Li-ion batteries, Nano Energy, 2016, 22, 48–58 CrossRef CAS.
- X. L. Wu, L. Y. Jiang, F. F. Cao, Y. G. Guo and L. J. Wan, LiFePO4 nanoparticles embedded in a nanoporous carbon matrix: superior cathode material for electrochemical energy-storage devices, Adv. Mater., 2009, 21, 2710–2714 CrossRef CAS.
- H. Liu, F. C. Strobridge, O. J. Borkiewicz, K. M. Wiaderek, K. W. Chapman, P. J. Chupas and C. P. Grey, Capturing metastable structures during high-rate cycling of LiFePO4 nanoparticle electrodes, Science, 2017, 344(6191), 1252817 CrossRef PubMed.
|
This journal is © The Royal Society of Chemistry 2019 |