DOI:
10.1039/C8RA10330E
(Paper)
RSC Adv., 2019,
9, 3734-3739
Enhanced fluorescent effect of graphitic C3N4@ZIF-8 nanocomposite contribute to its improved sensing capabilities†
Received
17th December 2018
, Accepted 21st January 2019
First published on 28th January 2019
Abstract
A novel graphitic carbon nitride (g-C3N4)@ZIF-8 nanocomposite was synthesized by a facile approach and applied as a fluorescent sensor. The fluorescent quenching and enhancing effect of g-C3N4@ZIF-8 nanocomposite was explored for potential applications in sensing metal ions and solvents based on photoluminescence (PL) measurements. Compared with g-C3N4 nanosheets alone, the combined g-C3N4@ZIF-8 nanostructure greatly improved the sensitivity for the detection of metal ions due to the special fluorescent quenching effect. In particular, the sensitivities of the detection of Cu2+ and Ag+ improve from 29.1 to 11.2 ppm and from 40 to 16.5 ppm, respectively, which were significantly improved compared to that of g-C3N4 nanosheets alone. The sensitivity could increase about 100% and 250% for Cu2+ and Ag+ by the g-C3N4@ZIF-8. The limit of detection (LOD) for Cu2+ and Ag+ by g-C3N4@ZIF-8 was 11.2 ppm and 13.2 ppm, respectively. Both g-C3N4 nanosheets and g-C3N4@ZIF-8 nanocomposite can be used for distinguishing Fe2+ and Fe3+ in solutions. The LOD of Fe2+ by g-C3N4@ZIF-8 was as low as 65.4 ppm. On the other hand, the relative increased luminescence of g-C3N4@ZIF-8 to the g-C3N4 in tetrahydrofuran (THF) was selective, which was successfully applied for detecting this specific solvent. An excellent linear relationship (0–1.0 v/v of THF/water) between the THF fraction and PL intensities was obtained. These results demonstrate that the g-C3N4@ZIF-8 nanocomposite provide a convenient and novel approach for the enhance fluorescence detection of metal ions and distinguishing of specific solvents.
1 Introduction
Heavy metal ions and their derivatives are of great current interest owing to their severe adverse impact on the environment and human health.1 For example, copper is an essential trace element for the living organisms, but an excessive copper is toxic when it accumulated in living organism.2 Similar to copper ion, silver ion can accumulate in the living organism and cause severe physiological problem.3 Through various absorption channels such as soil, reservoir water, food, these heavy metal ions can gather in developing brains, or binding to protein/peptide, leading to debilitating diseases.4 As a result, a variety of methods have been developed for detecting the harmful heavy metal ions, for example, coupled plasma mass spectroscopy,5 atomic absorption spectroscopy,6 Raman spectroscopy,7 electrochemical method,8 and fluorescent methods.1 Among these methods, fluorescent method is facial and highly sensitive.9 Organic dyes and semiconductor quantum dots are the most commonly used fluorescent probes.1 However, these materials suffer from some intrinsic detects such as easy oxidation, photobleaching, high toxicity and cost, which hampered their practical applications greatly.10 Therefore, it is highly desirable to develop novel materials to address these issues, which are suitable to be used as sensitive elements for the detection of metal ions.
Metal–organic frameworks (MOFs), self-assembled by the coordination of metal ion/cluster with organic linkers, is a new porous material.11 The structural rigidity, high porosity, and turntable component support its potential applications in a variety of fields, such as gas separation and storage,12 catalysis,13 sensing,14 and biomedical applications.15 ZIF-8 is often composited by a zinc ion center and 2-methylimidazole (Hmim) ligand, which belongs to the superfamily of MOFs and possess a sodalite (SOD)-type structure.16 Pyridyl nitrogen sites have been reported to be able to selectively bind to metal ions and used as metal ion sensor.17 With similar structure, imidazole-based conjugated polymer has also been reported to be used as a metal ion sensor.18 Since the imidazole nitrogen has specific affinity to metal ions, ZIF-8 can be used for detecting the metal ions.19 However, the fluorescence of ZIF-8 is low and thus limits its selectivity and detection sensitivity. Thus, ZIF-8 encapsulated AlQ3 (tris(8-hydroxyquinoline)-aluminium), FITC (fluorescein isothiocyanate) and Eu(III)-complex-functionalized Fe3O4, and Zn2GeO4:Mn2+ nanorods were reported as a luminescent ions sensor.20–22
Recently, graphitic carbon nitride (g-C3N4) has attracted much attention due to its decisive advantages such as easy preparation, high thermal stability, high visible photocatalysis activity, and high water splitting activity.23–25 Fluorescent g-C3N4 quantum dots used for detecting heavy metal ions have been reported very recently.26–29 The selectivity and sensitivity of g-C3N4 for detecting copper ions are reasonable because the photoinduced electron transfer (PET) between the CB of g-C3N4 to the complexed Cu2+ leading to the fluorescent quenching.26 Additionally, g-C3N4 decorated ball-like Co3O4 composite exhibited 1.6 times higher sensitivity than that of pure Co3O4.30 The g-C3N4/Bi2MoO6 composite which displayed nearly 3-fold and 6-fold enhanced photocurrent intensity than pure g-C3N4 and Bi2MoO6, could be applied in copper ion sensor with a linear range from 3 nM to 40 μM by a photoelectrochemical method.31 The C3N4-tyrosinase hybrid was a sensitive fluorescent probe for the detection of dopamine with detection limit as low as 3 × 10−8 mol L−1.32 Many carbon nitride nanohybrid, including C3N4/metal oxide nanoparticles, metal nanoparticles, metal–organic frameworks, graphene have been applied in the sensor application.33–38
It is thus tempted to combine the advantages of g-C3N4 and ZIF-8 to develop novel materials that are suitable for sensing metal ions. Herein, a facile method was developed for the preparation of g-C3N4@ZIF-8 nanocomposite that is suitable to be used as sensitive elements for the detection of metal ions and solvents. The morphology and structure of g-C3N4@ZIF-8 nanocomposite was characterized. Photoluminescence (PL) measurements were carried out to test the performance of g-C3N4@ZIF-8 nanocomposite for sensing metal ions. The fluorescent quenching effect can not only be found during sensing copper and silver ions, but also be observed during sensing Fe2+. On the contrary, the Fe3+ has little fluorescent quenching effect. The comparative phenomenon on Fe2+ and Fe3+ fluorescent quenching effect could be used for distinguishing the Fe2+ and Fe3+. In addition, compare to other solvents, tetrahydrofuran (THF) has the most enhancive fluorescence for g-C3N4@ZIF-8, which could be potentially applied for distinguishing this THF from other solvents.
2 Materials and methods
2.1 Materials
Melamine, Zn(NO3)2·6H2O, HgCl2, CaCl2, NaCl, FeCl2, FeCl3, KCl, MnCl2·4H2O, Li(CH3COO)2, SnCl2, CoCl2·6H2O, AgNO3, CuCl2, Ni(NO3)2·6H2O were ordered from Sinopharm Chemical Reagent Co., Ltd. Tetrahydrofuran (THF), 2-methylimidazole (Hmim), dimethylformamide (DMF), ethanol, methanol, and acetone were purchased from Sigma Aldrich. All the other chemicals were analytical pure grade or better quality. Deionized water (18.2 MΩ cm−1) produced by a Milli-Q system (Bedford, MA) was used throughout.
2.2 Synthesis of the g-C3N4 nanosheet
The g-C3N4 nanosheet was synthesized according to previous report.39 For the first, bulk C3N4 was firstly synthesized by pyrolysis of melamine. Typically, 5 g melamine was heated to 550 °C with a ramp rate of 3°C min−1 and maintained at the final temperature for 4 h in the air environment. For the next, the resultant yellow powder was milled for thermal treatment. The thermal treatment was conducted in an open ceramic container with a heating rate of 5 °C min−1 to 500 °C and maintained for 2 h. Through the process, the yellow powder turned into a light-yellow product.
2.3 Synthesis of the g-C3N4@ZIF-8
Graphitic C3N4@ZIF-8 was synthesis by an in situ method. Briefly, 10 mg of g-C3N4 was firstly dissolved into a 5 mL of 75 mM Hmim methanol solution and stirred for 10 min. Then, 5 mL of 150 mM Zn(NO3)2·6H2O methanol solution was added. For the next, the solution was left undisturbed for 2 h. The finally product was collected by the centrifugation method and washed with methanol for 3 times.
2.4 PL measurements
For sensing various metal ions, 0.2 mL of 1 mM g-C3N4@ZIF-8 DMF solution was added to 6 mL of DMF to form a homogeneous solution. Certain volumes of 1 mM DMF solution with metal ions were added to the g-C3N4@ZIF-8 solution. The excitation wavelength was 330 nm and the emission peak were 450 nm. Since silver ions can be reduced by DMF,40 silver ion test was conducted in aqueous solution.
For sensing properties with respect to various solvents, 0.2 mL g-C3N4@ZIF-8 solution was added to 6 mL solvent (DMF, distilled water, acetone, methanol, ethanol, THF). After shaking 2 min, 3 mL of the solution was used for PL measurements.
2.5 Characterization
X-ray diffraction (XRD) patterns of the samples were recorded on a D2 PHASER (BRUKER) diffractometer with Cu Kα irradiation, 30 kV, 10 mA. The structure and morphology of the samples were determined by scanning electron microscopy (SEM) and transmission electron microscopy (TEM) performed on PHILIPS XL30 and PHILIPS Tecnai 12, respectively. Fourier transform infrared spectroscopy (Spectrum 100, PerkinElmer) spectrum of samples were recorded using a KBr disk in the range of 4000 to 400 cm−1. Nitrogen physisorption isotherms were measured at 77 K on automatic Nova Station A. The UV-visible spectrum was performed on Agilent Cary 4000. The PL spectroscopy of the samples was carried on Hitachi F 4600.
3 Results and discussion
3.1 Characterization of g-C3N4@ZIF-8
Enable to test the PL spectrum, the stability of the nanoparticles is of great importance. Therefore, the dispersion of g-C3N4@ZIF-8 nanocomposite and g-C3N4 nanosheet in DMF solution was investigated at different times. The result indicates that the g-C3N4@ZIF-8 nanocomposite solution was more homogeneous and stable than that of bulk g-C3N4 solution (Fig. 1), which indicating the PL intensity change was only induced by the metal ions but not the nanoparticles sedimentation. This suggested a good stability of g-C3N4@ZIF-8 nanocomposite was suitable for PL spectrum measurements.
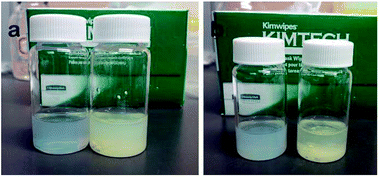 |
| Fig. 1 Dispersion of g-C3N4@ZIF-8 nanostructure (left) and bulk g-C3N4 (right) in DMF solution at different times: (a) 0 min, (b) 10 min. | |
The morphologies of g-C3N4 nanosheet and g-C3N4@ZIF-8 were observed by SEM and TEM, respectively. As shown in Fig. 2, the results indicate the g-C3N4 dispersed well and was the analogue of wrinkled graphene. After in situ decorated with ZIF-8, small nanoparticles assembled on the g-C3N4 nanosheet, leaving fewer margins. This ensured that the metal ions or solvents must cross the pores of ZIF-8 to interact with g-C3N4. The high resolution TEM images indicated that all nanoparticles assembled on the nanosheet with average diameter of 50 nm. In addition, N2 absorption and desorption isotherms of the g-C3N4@ZIF-8 (Fig. S1†) further confirm its surface area and the pore structure.
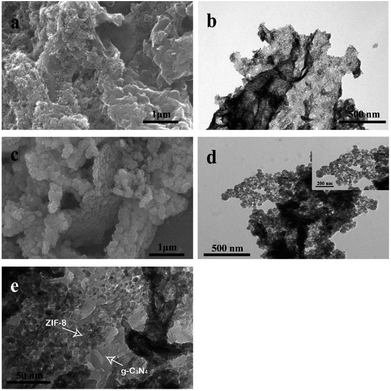 |
| Fig. 2 (a) SEM image and (b) TEM image of g-C3N4 nanosheets; (c) SEM image and (d) and (e) TEM images of g-C3N4@ZIF-8 nanostructures. | |
The structure of the nanocomposite was confirmed by XRD pattern. As shown in Fig. 3a, a peak of 7.0° was the characteristic of ZIF-8. The sharp peak at 27.7° corresponded to the nanosheet of g-C3N4.39 The further FTIR spectrum confirmed the component (Fig. 3b). The sharp peak at around 810 cm−1 was originated from heptazine ring system. The peaks range from 900 to 1800 cm−1 were associated to C–N–C or C–NH–C units.39 The peak at 421 cm−1 was attributed to Zn–N stretching. And peaks 1350–1500 cm−1 were attributed to the entire ring stretching of imidazole.41 The UV-visible spectra results indicated that after decorated with ZIF-8, the absorption edge and peak of g-C3N4 did not shift (Fig. 3c). The strong peak at 319 nm was the characteristic peak of g-C3N4.26 Besides this peak, g-C3N4 also had two peaks at 370 nm and 380 nm, respectively. When excited at 320 nm, the emission peak appeared at 450 nm, which indicated the nanocomposite was fluorescent. To check whether the g-C3N4@ZIF-8 was an excitation-independent PL material, different excitation wavelength was applied. As showed in the Fig. 3d, when the excitation wavelength changed from 310 nm to 370 nm, the PL spectra did not shift, and a peak around 450 nm was invariable.
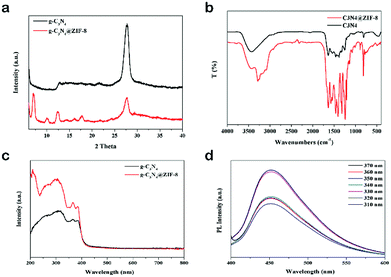 |
| Fig. 3 (a) XRD patterns and (b) FTIR spectra of g-C3N4 and g-C3N4@ZIF-8; (c) UV-visible spectra of g-C3N4 and g-C3N4@ZIF-8; (d) PL spectra of g-C3N4@ZIF-8 with excitation of different wavelengths. | |
3.2 Detection of metal ions
Screening experiments with different metal ions (66 ppm) were carried out by investigating the changes in PL intensity of the g-C3N4@ZIF-8. As showed in Fig. 4, the PL intensities of g-C3N4@ZIF-8 were greatly decreased by Fe2+, Cu2+ and Ag+, while the other ions presented less or no effect. This indicated that g-C3N4@ZIF-8 was sensitive to the specific ions and can be used as sensitive elements for the fluorescent detection of metal ions. The sensitivity of g-C3N4@ZIF-8 for Fe2+, Cu2+ and Ag+ may be due to the higher binding affinity of these ions to N of the ZIF-8 and g-C3N4.17–19,42,43 Due to the high porosity of ZIF-8, a large number of metal ions can interact with g-C3N4. Therefore, g-C3N4@ZIF-8 had a higher sensitivity than g-C3N4, especially for Ag+ and Cu2+. Additionally, using DMF replacing water could limit the hydrolysis of transition metal ions.
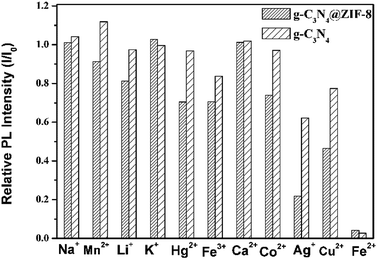 |
| Fig. 4 Differences in PL intensities of g-C3N4 and g-C3N4@ZIF-8 between blank and solutions containing different metal ions (λex = 330 nm, metal ion concentration of 66 ppm). | |
To evaluate the g-C3N4@ZIF-8 and g-C3N4's sensitivities toward different concentrations of Cu2+, Ag+ and Fe2+, PL intensities were monitored by the titration method. The enhanced selectivity was observed for Cu2+ and Ag+. Therefore, these two ions were tested by g-C3N4@ZIF-8 and g-C3N4, individually. It can be seen from Fig. 5a and b that the PL intensity of g-C3N4@ZIF-8 and g-C3N4 decreased gradually with increasing of Cu2+ concentrations. The limit of detection (LOD) for sensing Cu2+ by g-C3N4@ZIF-8 was about 11.2 ppm because less Cu2+ did not influence the PL intensity. A linear relationship between Cu2+ concentration and PL intensity in the range of 5 ppm to 11 ppm was obtained (Fig. 5g). The LOD of Cu2+ was lower than the maximum allowable level of Cu2+ (∼20 μM) in the drinking water regulated by US Environmental Protection Agency (EPA).44 While for the g-C3N4, the LOD for sensing Cu2+ was about 22.8 ppm. It indicated that the using of g-C3N4@ZIF-8 for sensing Cu2+ was able to increase the LOD by about 100%. Similar to Cu2+, with increasing in the concentrations of Ag+, the PL intensities of g-C3N4@ZIF-8 and g-C3N4 gradually decreased (Fig. 5c and d). The LOD for sensing Ag+ by g-C3N4@ZIF-8 and g-C3N4 was 13.2 ppm and 33.8 ppm, respectively. The LOD for sensing Ag+ by g-C3N4@ZIF-8 was enhanced by about 250%. A linear response was also obtained for sensing Ag+ by g-C3N4@ZIF-8 (Fig. 5h).
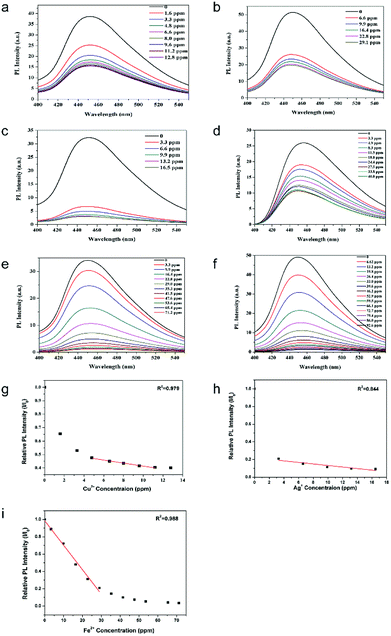 |
| Fig. 5 (a) and (b) PL intensity evolution of (a) g-C3N4@ZIF-8 and (b) g-C3N4 in the presence of different concentrations of Cu2+; (c) and (d) PL intensity evolution of (c) g-C3N4@ZIF-8 and (d) g-C3N4 in the presence of different concentrations of Ag+; (e) and (f) PL intensity evolution of (c) g-C3N4@ZIF-8 and (d) g-C3N4 in the presence of different concentrations of Fe2+; linear regression line of PL intensities of g-C3N4@ZIF-8 for sensing (g) Cu2+, (h) Ag+ and (i) Fe2+. I and I0 are PL intensities of in the presence and absence of metal ions, respectively. | |
Organic dyes were the most commonly used sensing material to distinguish Fe2+ and Fe3+ due to its convenient and selectively.45 However, organic dyes are only specific to one kind of metal ions, which can't be used for the detection of several kinds of metal ions. In this work, g-C3N4 nanosheet and g-C3N4@ZIF-8 can be easily prepared, which show the capability of sensing Fe2+ with high selectivity (Fig. 5e and f). It indicated that the LOD of Fe2+ by g-C3N4@ZIF-8 was about 65.4 ppm with a linear range of 0–30 ppm.
3.3 Detection of specific solvents
Changes in the PL intensities of g-C3N4@ZIF-8 and g-C3N4 in different solvents were also investigated. Various solvents were tested as a comparison to the PL intensity of g-C3N4@ZIF-8 and g-C3N4 in DMF. The PL intensity of H2O was same to the DMF, indicating the replace of DMF to H2O for the further solvent sensor. As shown in Fig. 6a, the relative PL intensities of g-C3N4@ZIF-8 to g-C3N4 were higher in THF than that of in other solvents. This selectivity can be used for detecting THF solvent. As shown in Fig. 6b, when the THF concentration was increased, the intensity of g-C3N4@ZIF-8 was increased gradually. An fitting linear relationship between the THF fraction and PL intensities (THF volume fraction from 0–1.0) was found by this method as shown in Fig. 6c. All the results suggested that this method provide a novel approach for the detection of THF in water solution.
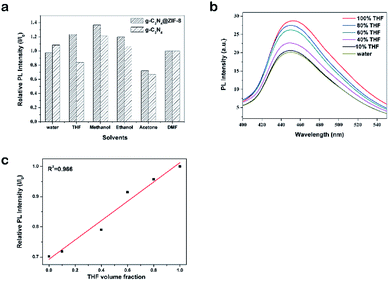 |
| Fig. 6 (a) Relative PL intensities of g-C3N4 and g-C3N4@ZIF-8 in various solvents (λex = 330 nm, particles concentration 60 ppm, I and I0 are PL intensities of in the presence and absence of metal ions, respectively); (b) PL spectrum of different THF concentration in water (λex = 330 nm); (c) linear regression line of relative intensities of g-C3N4@ZIF-8 with various THF volume fraction (λem = 450 nm). | |
4 Conclusions
In summary, g-C3N4@ZIF-8 nanocomposite was facial prepared, characterized and applied for the enhanced fluorescent detection of metal ions and solvents based on the PL measurement. The enhanced selectivity of g-C3N4@ZIF-8 to g-C3N4 was obvious in detecting Cu2+ and Ag+. The sensitivities for the detection of Cu2+ and Ag+ improve from 29.1 to 11.2 ppm and from 40 to 16.5 ppm, respectively. Comparing to Fe3+, Fe2+ can selectively quench the fluorescence of g-C3N4@ZIF-8 and g-C3N4, which was successfully applied for distinguishing Fe3+ and Fe2+. The LOD of Fe2+ by g-C3N4@ZIF-8 was as low as 65.4 ppm. The enhanced PL intensities of g-C3N4@ZIF-8 by the THF can be used for detecting THF in water with a good linear relationship (THF volume fraction from 0–1.0). With further development and optimization, it is expected that g-C3N4@ZIF-8 nanocomposite have great potential to be applied as fast and selective sensitive elements for enhanced fluorescence detection of metal ions and solvents.
Conflicts of interest
There are no conflicts to declare.
Acknowledgements
This work was supported by the National Natural Science Foundation of China (Grant No. 31470956, 31661143030, 31700859, 51861145307), the Doctoral Fund of Education Ministry of China (Grant No. 2016M602832), and the Fundamental Research Funds for the Central Universities.
References
- G. Aragay, J. Pons and A. Merkoçi, Chem. Rev., 2011, 111, 3433 CrossRef CAS PubMed
. - K. G. Daniel, R. H. Harbach, W. C. Guida and Q. P. Dou, Front. Biosci., 2004, 9, 2652 CrossRef CAS
. - H. T. Ratte, Environ. Toxicol. Chem., 1999, 18, 89 CrossRef CAS
. - L. Järup, Br. Med. Bull., 2003, 68, 167 CrossRef
. - S. Su, B. Chen, M. He and B. Hu, Talanta, 2014, 123, 1 CrossRef CAS PubMed
. - B. Dai, M. Cao, G. Fang, B. Liu, X. Dong, M. Pan and S. Wang, J. Hazard. Mater., 2012, 219, 103 CrossRef PubMed
. - Y. X. Yuan, L. Ling, X. Y. Wang, M. Wang, R. A. Gu and J. L. Yao, J. Raman Spectrosc., 2007, 38, 1280 CrossRef CAS
. - G. Aragay, J. Pons and A. Merkoci, J. Mater. Chem., 2011, 21, 4326 RSC
. - S. Goswami and R. Chakrabarty, Tetrahedron Lett., 2009, 50, 5910 CrossRef CAS
. - K. T. Yong, W. C. Law, R. Hu, L. Ye, L. Liu, M. T. Swihart and P. N. Prasad, Chem. Soc. Rev., 2013, 42, 1236 RSC
. - H. C. Zhou, J. R. Long and O. M. Yaghi, Chem. Rev., 2012, 112, 673 CrossRef CAS PubMed
. - J. R. Li, J. Sculley and H. C. Zhou, Chem. Rev., 2011, 112, 869 CrossRef PubMed
. - J. Lee, O. K. Farha, J. Roberts, K. A. Scheidt, S. T. Nguyen and J. T. Hupp, Chem. Soc. Rev., 2009, 38, 1450 RSC
. - L. E. Kreno, K. Leong, O. K. Farha, M. Allendorf, R. P. Van Duyne and J. T. Hupp, Chem. Rev., 2011, 112, 1105 CrossRef
. - P. Horcajada, R. Gref, T. Baati, P. K. Allan, G. Maurin, P. Couvreur, G. Férey, R. E. Morris and C. Serre, Chem. Rev., 2011, 112, 1232 CrossRef PubMed
. - R. Banerjee, A. Phan, B. Wang, C. Knobler, H. Furukawa, M. O'Keeffe and O. M. Yaghi, Science, 2008, 319, 939 CrossRef CAS PubMed
. - B. Chen, L. Wang, Y. Xiao, F. R. Fronczek, M. Xue, Y. Cui and G. Qian, Angew. Chem., Int. Ed., 2009, 48, 500 CrossRef CAS PubMed
. - A. Salinas-Castillo, M. Camprubi-Robles and R. Mallavia, Chem. Commun., 2010, 46, 1263 RSC
. - S. Liu, Z. Xiang, Z. Hu, X. Zheng and D. Cao, J. Mater. Chem., 2011, 21, 6649 RSC
. - T. T. Han, H. L. Bai, Y. Y. Liu and J. F. Ma, J. Solid State Chem., 2019, 269, 588 CrossRef CAS
. - J. Wang, H. Chen, F. Ru, Z. Zhang, X. Mao, D. Shan, J. Chen and X. Lu, Chem.–Eur. J., 2018, 24, 3449 Search PubMed
. - Y. Xu, S. Wu, X. Li, Z. Wang, Y. Han, J. Wu and X. Zhang, Mater. Lett., 2018, 210, 235 CrossRef CAS
. - F. Su, S. C. Mathew, G. Lipner, X. Fu, M. Antonietti, S. Blechert and X. Wang, J. Am. Chem. Soc., 2010, 132, 16299 CrossRef CAS PubMed
. - D. J. Martin, K. Qiu, S. A. Shevlin, A. D. Handoko, X. Chen, Z. Guo and J. Tang, Angew. Chem., Int. Ed., 2014, 53, 9240 CrossRef CAS PubMed
. - S. C. Yan, Z. S. Li and Z. G. Zou, Langmuir, 2010, 26, 3894 CrossRef CAS PubMed
. - J. Tian, Q. Liu, A. M. Asiri, A. O. Al-Youbi and X. Sun, Anal. Chem., 2013, 85, 5595 CrossRef CAS
. - S. Barman and M. Sadhukhan, J. Mater. Chem., 2012, 22, 21832 RSC
. - H. Huang, R. Chen, J. Ma, L. Yan, Y. Zhao, Y. Wang, W. Zhang, J. Fan and X. Chen, Chem. Commun., 2014, 50, 15415 RSC
. - S. Zhang, J. Li, M. Zeng, J. Xu, X. Wang and W. Hu, Nanoscale, 2014, 6, 4157 RSC
. - Y. Gong, Y. Wang, G. Sun, T. Jia, L. Jia, F. Zhang, L. Lin, B. Zhang, J. Cao and Z. Zhang, Nanomaterials, 2018, 8, 132 CrossRef PubMed
. - S. Chen, N. Hao, D. Jiang, X. Zhang, Z. Zhou, Y. Zhang and K. Wang, J. Electroanal. Chem., 2017, 787, 66 CrossRef CAS
. - H. Li, M. Yang, J. Liu, Y. Zhang, Y. Yang, H. Huang, Y. Liu and Z. Kang, Nanoscale, 2015, 7, 12068 RSC
. - S. Ansari, M. S. Ansari, H. Devnai, S. P. Satsangee and R. Jain, Sens. Actuators, B, 2018, 273, 1226 CrossRef CAS
. - C. Sun, M. Zhang, Q. Fei, D. Wang, Z. Sun, Z. Geng, W. Xu and F. Liu, Sens. Actuators, B, 2018, 256, 160 CrossRef CAS
. - S. Hu, W. Quyang, L. Guo, Z. Lin, X. Jiang, B. Qiu and G. Chen, Biosens. Bioelectron., 2017, 92, 718 CrossRef CAS PubMed
. - S. Zhang, N. Hang, Z. Zhang, H. Yue and W. Yang, Nanomaterials, 2017, 7, 12 CrossRef PubMed
. - H. Wang, Z. Guo, W. Hao, L. Sun, Y. Zhang and E. Cao, Mater. Lett., 2019, 234, 40 CrossRef CAS
. - S. De, N. Venkataramani, S. Prasad, R. O. Dusane, L. Presmanes, Y. Thimont, P. Taihades, V. Baco-Carles, C. Bonningue, S. T. Pisharam and A. Barnabe, IEEE Sens. J., 2018, 18, 6937 CAS
. - P. Niu, L. Zhang, G. Liu and H. M. Cheng, Adv. Funct. Mater., 2012, 22, 4763 CrossRef CAS
. - I. Pastoriza-Santos and L. M. Liz-Marzán, Langmuir, 1999, 15, 948 CrossRef CAS
. - Y. Hu, H. Kazemian, S. Rohani, Y. Huang and Y. Song, Chem. Commun., 2011, 47, 12694 RSC
. - Y. Zhang, S. Meng, J. Ding, Q. Peng and Y. Yu, Analyst, 2019, 144, 504 RSC
. - S. Lee, Y. Jun, E. Lee, N. Heo, W. Hong, Y. Huh and Y. Chang, Carbon, 2015, 95, 58 CrossRef CAS
. - Y. T. Su, G. Y. Lan, W. Y. Chen and H. T. Chang, Anal. Chem., 2010, 82, 8566 CrossRef CAS PubMed
. - J. W. Stucki, Soil Sci. Soc. Am. J., 1981, 45, 638 CrossRef CAS
.
Footnote |
† Electronic supplementary information (ESI) available. See DOI: 10.1039/c8ra10330e |
|
This journal is © The Royal Society of Chemistry 2019 |