DOI:
10.1039/C8RA10091H
(Paper)
RSC Adv., 2019,
9, 11092-11100
Immunomodulatory activity of a fructooligosaccharide isolated from burdock roots
Received
8th December 2018
, Accepted 25th March 2019
First published on 9th April 2019
Abstract
A novel burdock fructooligosaccharide (BFO-1) was extracted from fresh burdock roots. In our study, we found that BFO-1 possessed immunoenhancing activity in vitro and in vivo. The proliferation activities of splenocytes were significantly stimulated at a BFO-1 dose of 1000 μg ml−1 (p < 0.05), peritoneal macrophages showed increased activities of phagocytosis and acid phosphatase and increased production of NO at a BFO-1 dose of 1000 μg ml−1 (p < 0.01) in vitro. In normal mice, BFO-1 promoted the activities of peritoneal macrophages at dosages of 250 and 500 mg per kg per day (p < 0.01). Similarly, in immunosuppressed mice, BFO-1 enhanced the activities of peritoneal macrophages at the dosage of 1000 mg per kg per day (p < 0.05). Furthermore, S180 tumor-bearing mice that were pre-administered BFO-1 solution showed improved immunological function and suppressed tumor growth. These data indicate that BFO-1 can improve the functioning of the immune system and has the potential to be developed as a dietary supplement and medicinal food.
Introduction
Currently, some common diseases are closely associated with a compromised immune system, which is due to problems of the immune system and nonspecific protection mechanisms being damaged in disease and cancer. For the treatment of immunocompromised patients, immunotherapy has attracted increasing attention. Plant polysaccharides are an important active ingredient in Chinese traditional medicine and have been widely studied for their safety and nontoxic characteristics. A large number of studies have shown that plant polysaccharides enhance the immune functions of the body and are one of the main mechanisms for their medicinal use. Plant polysaccharides not only activate immune cells such as T cells, B cells, macrophages, and NK cells but also promote the production of cytokines such as IL-1, IL-2, tumor necrosis factor and interferon, regulate the formation of antibodies and complement and exert various regulatory effects on the immune system.1–4
Burdock (Arctium lappa L.), which is a famous traditional Chinese medicine, has long been cultivated as a popular vegetable in Japan, Korea, Taiwan and has yet to be shown to cause side effects.5 Burdock roots contain large amounts of fructooligosaccharides, phenolic compounds and caffeoylquinic acid derivatives;6–8 fructooligosaccharides are usually considered to be their main bioactive components.9 Some studies have suggested that burdock has a variety of biological properties, such as antioxidant, antiallergic, immunoregulatory or immunostimulatory or immunostimulation10–15 and anti-inflammatory activities.16,17
Approximately 15% of angiosperms store carbohydrates in the form of fructooligosaccharides. Fructooligosaccharides, as burdock root's main bioactive components, are a kind of fine functional food material that has been used globally. In addition to being a sugar alcohol, fructooligosaccharide also regulates intestinal flora,18–21 lowers blood sugar,22 regulates fat metabolism,23 promotes the absorption of minerals, increases the number of Peyer's patch,24 promotes the production of short chain fatty acids (SCFAs)25 and so on.
Our laboratory isolated and purified a homogeneous fructo-oligosaccharide from fresh burdock roots named BFO-1, which consisted of glucose and fructose, composed of D-fructose and D-glucose in a molar ratio of approximately 12
:
1. BFO-1 is confirmed to be composed of 12 fructose residues linked by U (2→1) glucoside bonds and 1 glucose residue linked by a T (1→2) glucoside bond at the end of the linear straight sugar chain. Preliminary experiments confirmed that BFO-1 could promote plant growth and inhibit plant diseases. In addition, BFO-1 induced the growth of Bifidobacterium in vitro, but the immunomodulatory activity of BFO-1 has not been studied. Accordingly, in this study, we investigated the immunomodulatory activity of BFO-1 for the first time. We then investigated the effect of BFO-1 on immune cells, normal mice, and immunosuppressed mice treated with cyclophosphamide and S180 tumor-bearing mice. Our results provide insights into the understanding of BFO-1 from burdock roots and may facilitate its application in biomedical fields.
Materials and methods
Materials
D301R resin was provided by Nankai University. Sephadex G-50 was purchased from General Electric Healthcare Life Sciences. Fetal bovine serum and RPMI-1640 media were obtained from GIBCO GRL (USA). Lipopolysaccharide (LPS), 3-(4,5-dimethylthiazol-2-y1)-2,5-diphenyltetrazoliumbromide (MTT), concanavalin A (ConA) and p-nitrophenyl phosphate (pNPP) were purchased from Sigma-Aldrich Chemical Co. (St. Louis, MO, USA). Sheep red blood cells (SRBCs) were obtained from Solarbio.
Isolation and fractionation of burdock fructooligosaccharide
The roots of fresh burdock were cut into pieces and immersed in hot water at a solid
:
liquid ratio of 1
:
10, at a temperature of 70 °C, and an extraction time of 90 min, which was repeated twice. The liquid was concentrated by rotary evaporation at 60 °C to 1/3 of the original volume and mixed with three volumes of 95% ethanol for 24 h at 4 °C. The samples were deproteinized according to the method of Sevage (1938), then decolorized with D301R and freeze-dried to obtain crude burdock fructooligosaccharide. The crude burdock fructooligosaccharide was fractionated by Sephadex G-50 column chromatography (1.6 cm × 60 cm) and eluted with distilled water with a flow rate of 0.5 ml min−1. The fraction of the major peak was collected, lyophilized and pure burdock fructooligosaccharide named BFO was obtained.
Removal of bacterial endotoxins from burdock fructooligosaccha-ride
DEAE-cellulose column chromatography was used to remove bacterial endotoxins. The DEAE-cellulose was weighed, and three times the amount of water was added to dissolve the DEAE-cellulose, which was stabilized by 2–3 times the volume of the column after the alkali-acid-alkali treatment. BFO was eluted with distilled water at a flow rate of 1 ml min−1, and the concentrated liquor was lyophilized and named BFO-1. The endotoxin level was measured at the end point by the chromogenic reagent TAL.
Animal
Kunming mice (20 ± 2 g, female) were obtained from the Animal Centre of Shandong University. Mice were kept in a room under standard housing conditions (12 h light/12 h dark, 25 ± 1 °C with 55 ± 5% relative humidity). All experiments were performed in compliance with the relevant laws and approved by the Ethics Committee of Shandong University with the approval/protocol number SYDWLL-2018-12, which is according to the regulations for the Administration of Affairs Concerning Experimental Animals of China.
The proliferation of splenocytes
Kunming mice were sacrificed by cervical dislocation, and the spleens were aseptically acquired. The single-cell suspensions were obtained by pressing the suspension with a syringe core and filtering through a 200-mesh steel sieve. The erythrocytes were removed by the hypotonic method and then washed three times with phosphate buffered saline (PBS) and centrifuged at 1000 rpm for 5 min. Finally, splenocytes were suspended at 1 × 106 ml−1 in RPMI 1640 medium supplemented with 10% fetal bovine serum. The cells were seeded in 96-well plates with different concentrations of BFO-1 or ConA (5 μg ml−1), followed by incubation for 72 h at 37 °C with 5% CO2 in a humidified incubator. A 5 mg ml−1 MTT solution (10 μl) was added to each well before the end of the culture for 4 h and incubated continuously for 4 h. The supernatant was removed carefully, and DMSO (150 μl) was added to each well until the blue-purple crystallization of formazan was completely dissolved. Then, the absorbance was measured with a microplate reader at 570 nm.
The production of NO in peritoneal macrophages
Mice were injected with 1 ml of aseptic 3% starch broth in the abdominal cavity for 3 days. Five millilitres of cold PBS solution was injected into the abdominal cavity, and the abdomen was gently massaged. Then, the peritoneal macrophages were collected and washed twice with PBS. The purified peritoneal macrophages (1 × 106 ml−1) were seeded in 96-well plates and treated with different concentrations of BFO-1 (0, 25, 50, 100, 500, 1000 μg ml−1) or LPS (1 μg ml−1) for 24 h. Then, the supernatant (50 μl) was placed in another 96-well cell culture plate, and Griess reagent (50 μl) was added, gently mixed and settled at room temperature for 10 min. Then, the absorbance of each well was measured at 490 nm with a microplate reader.
The determination of acid phosphatase activity in macrophage
Purified peritoneal macrophages (1 × 106 ml−1) were seeded in 96-well plates and treated with different concentrations of BFO-1 (0, 25, 50, 100, 500, 1000 μg ml−1) or LPS (1 μg ml−1) for 24 h. The supernatant was removed, and 100 μl of pNPP was added to every well. Then, the cells were sequentially cultivated for 30 min, and 10 μl of NaOH (1.0 mol l−1) was added to terminate the reaction. The absorbance of each well was measured by a microplate reader at 405 nm.
Phagocytic activity of peritoneal macrophages
The purified peritoneal macrophages were incubated alone for 24 h. Subsequently, the supernatant was discarded, and 100 μl of 0.1% neutral red dissolved in normal saline was added. Then, the cells were sequentially cultivated for 20 min, and 200 μl of cell lysis buffer composed of equal volumes of absolute ethanol and acetic acid was added to every well. The absorbance was measured at 490 nm under a microplate reader.
B cell-mediated hemolysis reaction
Mice were injected with 20% SRBC (0.2 ml) and sacrificed on day 8, and splenocytes were prepared according to the methods described above. One milliliter of spleen cell suspension (1 × 107 ml−1), 0.5 ml of 0.2% SRBC and 0.5 ml of 1
:
10 guinea pig complement was mixed and incubated for 60 min at 37 °C; finally, the reaction was stopped in an ice bath. The cell suspension was then centrifuged (3000 rpm for 5 min), and the absorbance of the supernatant was measured by a microplate reader at 413 nm.
The determination of serum hemolysin
Mice were injected with 20% SRBC (0.2 ml) and sacrificed on day 8. The serum was separated and diluted with physiological saline at a ratio of 1
:
100. One millilitre of serum was mixed with 5% SRBC (0.5 ml) and 1
:
10 guinea pig complement (0.5 ml). After a 60 min incubation at 37 °C, the reaction was terminated in an ice bath and the mixture was centrifuged at 3000 rpm for 5 min. The absorbance was measured at 540 nm using a microplate reader.
Experimental design for normal mice
The mice were randomly divided into five groups (n = 6/group): the control group and the four experimental groups. After acclimation for 1 week, in the experimental groups, the mice were orally administered BFO-1 (100, 250, 500, and 1000 mg kg−1) every day for a week, and the control group was administered the equivalent volume of saline. After 1 week, all groups were euthanized to prepare splenic cells and peritoneal macrophages as described above. Then, the proliferation of splenocytes, the phagocytic activity of peritoneal macrophages, the production of NO and the acid phosphatase activity, B cell-mediated hemolysis reaction and the production of serum hemolysin were measured as described above.
Experimental design for cyclophosphamide-treated immunosuppressed mice
The Kunming mice were randomly divided into five groups (n = 6/group). In the experimental groups, the mice were injected with 75 mg kg−1 d−1 cyclophosphamide (CY) for 3 days, and the control group was injected with the equivalent volume of saline. Then, the experimental groups were treated with normal saline and different concentrations of BFO-1 (250, 500, 1000 mg kg−1) by lavage every day for 7 days. Then, the proliferation of splenocytes, the phagocytic activity of peritoneal macrophages, the production of NO and the acid phosphatase activity, B cell mediated hemolysis reaction and the production of serum hemolysin were measured as described above.
Experimental design for S180 tumor-bearing mice
The Kunming mice were randomly divided into five groups (n = 8/group): the control group and the four experimental group. In the experimental groups, the mice were orally administered BFO-1 (250, 500, and 1000 mg kg−1) every day for a week and the control group was administered the equivalent volume of saline. The five groups of mice were subcutaneously inoculated with 0.2 ml of S180 cell suspension (1 × 107 ml−1) in the right upper limb on day 8, and then BFO-1 and saline were administered on the other 9 days. The mice were sacrificed, and the tumor tissue was detached and weighed. The tumor inhibition rate was calculated according to the following formula:
Tumor inhibition rate (%) = (Wcontrol − Wtreated)/Wcontrol × 100%, where Wtreated and Wcontrol were the average tumor weights of the treated and control groups, respectively. The phagocytic activity of the peritoneal macrophages and the production of NO were measured as described above.
Statistical analysis
All data are presented as the mean ± SD. The statistical calculations were performed using the Statistical Package for Social Sciences version 12.0 (SPSS Inc., Chicago, IL, USA). Significant values were evaluated by one-way ANOVA, and p-values of less than 0.05 were considered statistically significant.
Results
Isolation and removal of bacterial endotoxin from BFO
Endotoxin is the source of pollution of most biomaterials, and its presence has clouded the results of biological and drug tests and caused many production difficulties.26 The removal of endotoxin is a difficult problem, due to its high thermal stability and relatively large molecular weight; the stability and recovery rate of biological macromolecules should also be considered.27
BFO-1 was separated by a DEAE-cellulose column to obtain loose white lumps. DEAE cellulose is one of the most commonly used methods in the separation and purification of sugars. Results have shown that the method of removing bacterial endotoxin by DEAE cellulose is low cost, effective, has a high recovery rate and is also a suitable method for removing BFO-1 contaminating endotoxin. This study selected an appropriate method to determine the content of endotoxin. After treatment, the endotoxin measurement changed from 1.6702 EU ml−1 to 0.1065 EU ml−1. The endotoxin content was reduced by approximately 25 times, and the BFO-1 can therefore be used for further experiments. The removal of bacterial endotoxin by DEAE cellulose is effective and feasible.
The effect of BFO on the proliferation of splenocytes in vitro
In this study, the proliferation activity of splenic cells was determined by MTT assay. As shown in Fig. 1, compared with the control, BFO-1 effectively stimulated splenic cell proliferation in a dose-dependent manner and reached maximum proliferation at 1000 μg ml−1. This result indicates that BFO-1 can stimulate lymphocyte proliferation within a certain concentration range.
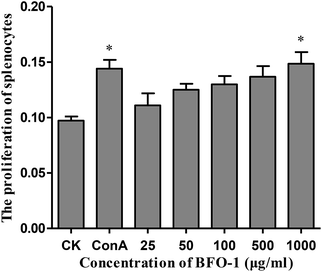 |
| Fig. 1 Effect of BFO-1 on the proliferation activity of spleen cells in vitro. Supernatants of ConA or BFO-1 treated spleen cells were collected, and the OD value was measured with a microplate reader at 570 nm. Data are presented as the means ± SD of six independent experiments. Significant differences from the control group are indicated by *p < 0.05, **p < 0.01. | |
The effect of BFO-1 on peritoneal macrophages in vitro
The acid phosphatase activity and the production of NO in peritoneal macrophages could not be enhanced by BFO-1 stimulation alone in vitro (data not shown), but LPS alone can stimulate peritoneal macrophages to produce NO. In this study, the synergistic effect of BFO-1 and LPS on the production of NO in macrophages was investigated. The results showed that NO was significantly increased after combined stimulation with BFO-1 and LPS. When the concentration of BFO-1 was 25–100 μg ml−1, the NO production tended to decrease as the concentration increased. When the concentration of BFO-1 was 500–1000 μg ml−1 in the test group, the production of NO was higher than in the control and LPS groups with increasing concentrations (Fig. 2A). It is possible that the mechanism of NO production stimulated by BFO-1 is different. How BFO-1 and LPS synergistically impact the production of NO is unclear. BFO-1 could enhance the activity of acid phosphatase in peritoneal macrophages. As the concentration increased, the activity of acid phosphatase also increased; at the concentration of 1000 μg ml−1, the acid phosphatase activity was dramatically higher than the control group (p < 0.05). The results are shown in Fig. 2B.
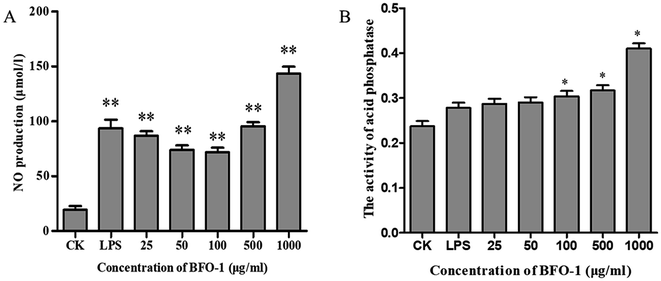 |
| Fig. 2 The effects of BFO-1 on peritoneal macrophages in vitro. (A) Effects of BFO-1 on the production of NO in macrophages. (B) Effect of BFO-1 on the activity of acid phosphatase in macrophages. Macrophages were treated with the indicated concentrations of BFO or LPS (1 μg ml−1) for 24 h. The results are expressed as the mean values ± SD (n = 6, *p ≤ 0.05 versus control group). | |
The immunostimulatory effects of BFO on normal mice
To further evaluate the immunostimulatory effect of BFO-1 on normal mice, BFO-1 was orally administered at dosages of 100, 250, 500 and 1000 mg per kg per day to normal mice for 7 days. The production of NO, the phosphatase activity and the phagocytic activity of peritoneal macrophages from normal mice are shown in Fig. 3. At concentrations of 250 and 500 mg per kg per day, the acid phosphatase activity, NO production and phagocytic activity of the BFO-1 groups were dramatically higher than those of the control group (p < 0.01). However, at 1000 mg per kg per day, the acid phosphatase activity and NO production of the BFO-1 groups were decreased, probably because the concentration of BFO-1 was too high. BFO-1 did not show toxic effects despite the high BFO-1 concentration. BFO-1 increased the proportion of activated macrophages at concentrations of 100–1000 mg per kg per day.
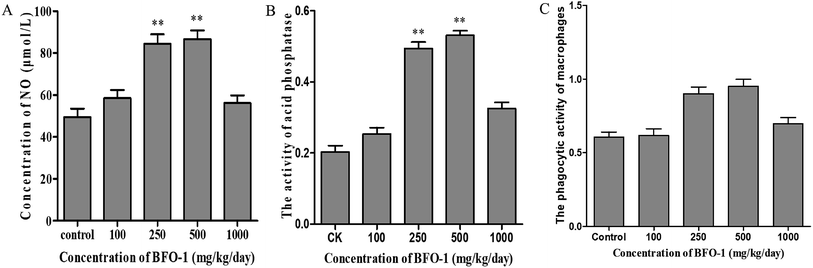 |
| Fig. 3 Effect of BFO-1 on peritoneal macrophages from normal mice. (A) Effect of BFO-1 on the production of NO in macrophages. (B) Effect of BFO-1 on the activity of acid phosphatase. (C) Effect of BFO-1 on the phagocytic activity of macrophages. The mice were intragastrically pretreated with the indicated concentrations of BFO-1 for 7 days. The results are expressed as the mean values ± SD (n = 6, *p ≤ 0.05 versus control group). | |
However, intragastric treatment of normal mice with BFO-1 for 7 days did not change the proliferation of splenic cells, the B cell-mediated hemolysis reaction or the determination of serum hemolysin compared with the control group mice. The phagocytic activity of macrophages, the production of cytokines, the ability of splenic cells to secrete antibodies, and the proliferation of splenic lymphocytes reflect the cellular and humoral immune functions of the immune system.
The immunostimulatory effects of BFO-1 in immunosuppressed mice
Proliferation of splenocytes. The splenocyte proliferation results are shown in Fig. 4. The proliferation activity of splenic cells was increased in the BFO-1 groups relative to the model group. Compared with the model group, splenic cells proliferation was stimulated in the BFO-1 treatment groups in a dose-dependent manner and proliferation reached peaked at 1000 mg per kg per day.
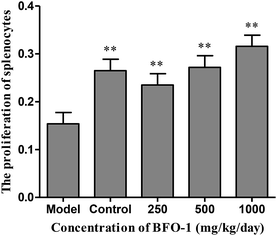 |
| Fig. 4 Effect of BFO-1 on the proliferation of splenocytes in immunosuppressed mice. The results are expressed as the mean values ± SD (n = 6, *p ≤ 0.05 versus model group). | |
Acid phosphatase activity. The acid phosphatase activity in the BFO-1 treated group at 500 and 1000 mg kg−1 was significantly higher than that in the model group (p < 0.01) (Fig. 5A). This result suggests that BFO-1 could improve the activity of acid phosphatase to enhance the function of macrophages.
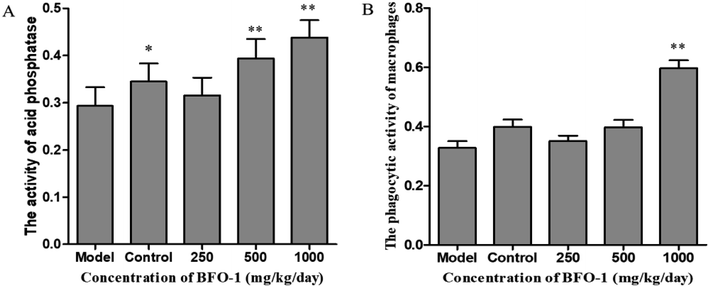 |
| Fig. 5 Effect of BFO-1 on peritoneal macrophages in immunosuppressed mice. (A) Effect of BFO-1 on acid phosphatase activity. (B) Effect of BFO-1 on the phosphatase activity. The results are expressed as the mean values ± SD (n = 6, *p < 0.05 versus model group). | |
Phosphatase activity. BFO-1 was orally administered at dosages of 250, 500 and 1000 mg per kg per day, and the phosphatase activity in peritoneal macrophages of immunosuppressed mice was improved. At 1000 mg kg−1, the phosphatase activity was dramatically higher than that of the control group (p < 0.01) (Fig. 5B).
The production of serum hemolysin. To evaluate the immunoregulatory effects of BFO-1 in cyclophosphamide induced immunosuppressed mice, the production of serum hemolysin was measured by a microplate reader at 540 nm (Table 1). At BFO-1 concentration of 1000 mg kg−1, the production of serum hemolysin was dramatically higher than that of the control group (p < 0.05). This result suggests that BFO increased the production of serum hemolysin in immunosuppressed mice.
Table 1 Effect of BFO-1 on the level of serum hemolysin and B cell mediated hemolytic activity in immunosuppressed micea
Groups |
Concentration (mg per kg per day) |
Serum hemolysin A540 |
Hemolytic activity A413 |
Date are expressed as the mean ± SD. Significantly different from the control stimulated:*p < 0.05, **p < 0.01. |
Model |
|
0.0182 ± 0.0112 |
0.1548 ± 0.0513 |
Normal control |
|
1.3720 ± 0.2416 |
0.7358 ± 0.0316** |
BFO |
250 |
0.0891 ± 10.2416 |
0.4009 ± 0.0372** |
500 |
0.2307 ± 0.1846 |
0.4986 ± 0.0592** |
1000 |
0.3340 ± 0.2760* |
0.6353 ± 0.0319** |
B cell mediated hemolytic activity. To evaluate the immunoregulatory effects of BFO-1 on cyclophosphamide-induced immunosuppression, B cell-mediated hemolytic activity was measured by a microplate reader at 413 nm (Table 1). The BFO-1 groups had significantly higher hemolytic activity than the model group (p < 0.01). This result suggests that BFO-1 improves B cell mediated hemolytic activity in immunosuppressed mice.
The effect of intragastric pretreatment with BFO-1 on the S180 mice
The macrophage activation induced by BFO-1 in vitro prompted us to evaluate the anti-tumor and immunomodulatory properties in vivo. We established a subcutaneous S180 tumor-bearing model in Kunming mice to evaluate the immunoregulatory effect of BFO-1.
Tumor inhibition. After intragastric pretreatment with BFO-1, the tumor growth of S180-bearing mice was significantly inhibited compared with the tumors from control group (Table 2). The tumor inhibition rate in the treatment groups (250 and 500 mg per kg per day) was approximately 25%, while the high dosage group (1000 mg per kg per day) reached an inhibition rate of 44%. This result indicates that BFO-1 has preventive effect on the occurrence of tumors. Currently, many studies in the anticancer field are devoted to finding materials with immunoregulatory and antitumor effects from natural biological materials, because traditional chemotherapies kill tumor cells and have a toxic effect on normal cells.28 Therefore, ideal antitumor drugs should have tumor suppression function, be harmless to the host or cause limited damage and specifically regulate immune cells to kill tumor cells.
Table 2 Effects of BFO-1 pretreatment on tumor weight in S180 tumor-bearing mice (n = 8)a
Treatment |
Concentration (mg kg−1) |
Tumor inhibition rate (%) |
Date are expressed as the mean ± SD. Significantly different from the control stimulation:*p < 0.05, **p < 0.01. |
Control |
|
|
BFO |
250 |
24.26 |
500 |
25.53 |
1000 |
44.14 |
Macrophage phagocytic. Intragastric pretreatment with BFO-1 can enhance phosphatase activity and the production of NO in macrophages. Compared with the control group of tumor-bearing mice, the BFO-1 groups (250, 500 and 1000 mg per kg per day) had improved macrophage phagocytic activity and an increased amount of NO (Fig. 6). This result suggests that the intragastric pretreatment effect of BFO-1 has a significant antitumor effect, which may be related to the recovery of immune function in S180 tumor-bearing mice. The immunodeficiency of tumor-bearing mice is closely related to the occurrence and development of tumors.29 The results of this study confirmed that BFO-1 can improve the function of macrophages in tumor-bearing mice and exert a positive immunoregulation effect. Therefore, BFO-1 has good application potential in tumor prevention.
Discussion
In our previous study, we found that BFO-1 is linear inulin-type fructooligosaccharides and acts as prebiotic that passes undigested through the upper part of the gastrointestinal tract. Inulin and fructooligosaccharides are partially hydrolyzed by gut microbes, may either bind to the intestinal epithelium and exert immune reactions locally and/or be absorbed into the bloodstream where they can potentially exert systemic effect.30 The current data indicate that BFO-1 enhanced immune function by affecting the activities of lymphocytes and peritoneal macrophages. The activities of splenocytes and peritoneal macrophages were stimulated in vitro (at the dose of 1000 μg ml−1) and in immunosuppressed mice (at the dosage of 1000 mg per kg per day). The activities of peritoneal macrophages were significantly stimulated at dosages of 250 and 500 mg per kg per day in normal mice. Tumor growth was inhibited, and peritoneal macrophages were significantly stimulated at dosages of 250, 500 and 1000 mg per kg−1 per day in S180 tumor-bearing mice. In general, BFO-1 can improve immunological function, but the optimal dose for functioning in different models is different.
It is well established that functional foods may exert their beneficial effects on health promotion through cell-to-cell communication networks.31 In these communication networks, the immune system plays an important role in the body by protecting the organism from infectious disease and tumors. The immune system is mainly composed of immune organs and cells, including the spleen, thymus, lymphocytes and so on.
Lymphocytes play an important role in immune system regulation, which is an important indicator of lymphocyte activation and function.32 Splenocytes are key effector cells of the innate immune response.33 In our study, BFO-1 effectively stimulated splenocyte proliferation in vitro, in immunosuppressed mice and in S180 tumor-bearing mice. These results indicated that BFO-1 could restore the function of humoral immunity. In normal mice, BFO-1 had no significant effect on the proliferation of splenocytes. These results indicated that BFO-1 had no significant effect on humoral immunity and T cell proliferation under these experimental conditions.
Macrophages together with neutrophils represent the first line of host defense after the epithelial barrier.34 Moreover, macrophages have different cellular morphologies with different functional conditions and play a vital role in the innate immune system by increasing the secretion of inflammatory molecules, such as NO and proinflammatory molecules, to enhance the immunity.35 Recent studies have shown that most fructooligosaccharides, such as the FOS isolated from Yacon,36 FOS and inulin,37–39 could invoke the activity of immunocyte.
The production of NO can activate macrophages and stimulate immune system to protect the host from infection and tumors.40 Nonetheless, an excess of NO in the cell may damage the DNA and have a proinflammatory effect.41 In our study, BFO-1 significantly increased the production of NO in normal mice and S180 mice, indicating that BFO-1 can enhance the innate immunity and activate the microbicidal processes inside cells. However, in normal mice, the production of NO was enhanced but did not continually increase with the increase of BFO-1 concentration. The production of NO at 1000 mg per kg per day was reduced compared to the concentrations of 250 and 500 mg per kg per day (Fig. 3A). In contrast, in S180 mice, the production of NO was concentration-dependent. This result indicates that the optimal concentration of BFO-1 required for improving immunity is different in normal and S180 mice.
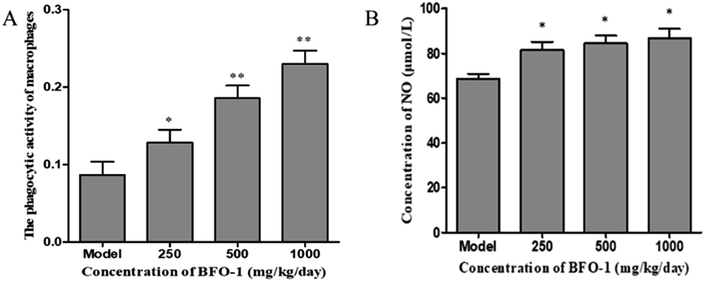 |
| Fig. 6 Effect of BFO-1 on the activities of peritoneal macrophages in S180 tumor-bearing mice. (A) Effect of BFO-1 on phosphatase activity. (B) Effect of BFO-1 on the production of NO in macrophages. The results are expressed as the mean values ± SD (n = 8, *p ≤ 0.05 versus model group). | |
The microbicidal activity in macrophages is related to a rapid and deep shift of the pH toward acidic values in phagolysosomes and to the action of lysozyme, hydrolytic enzymes and defensins.41 The selection of acid phosphatase and phagocytic function are important indicators of macrophages that reflect the state of cellular immunity. In this study, BFO-1 significantly increased the phagocytic function and acid phosphatase activity of peritoneal macrophages in normal mice, immunosuppressed mice and S180 mice. These results suggest that BFO can enhance the nonspecific immune and immunomodulatory effects of the body by activating macrophages.
Serum hemolysin and B-cell-mediated hemolysis demonstrate the ability of B lymphocytes to differentiate into plasma cells and produce antibodies when stimulated by antigens. In addition, B-cell mediated hemolysis reflects the number of lymphocyte antibodies, reflecting the ability of the immune system to synthesize antibodies.42 In our study, serum hemolysin levels and B-cell mediated hemolysis were used as indicators to detect humoral immunity. When observing the effect of BFO-1 on cyclophosphamide-treated immunosuppressed mice, it was found that BFO-1 had a restorative effect on cellular immunity and humoral immune function in mice. The enhancement effect of BFO-1 on immunosuppressed mice indicates that BFO-1 will be used as an auxiliary food for cyclophosphamide chemotherapy patients hopefully by improving the immune function of patients and preventing damage to the immune system.
In recent years, increasing focus has been placed on the biological activities of inulin and fructooligosaccharide especially the immunostimulatory and antitumor activities.43 However, there are no reports on the antitumor and immunomodulatory activity of BFO-1. In our study, intragastric pretreatment with BFO-1 significantly delayed the tumor growth in S180 tumor-bearing mice (Table 2). BFO-1 also enhanced the phagocytic function of macrophages and their ability to produce NO, which should mediate macrophages killing of tumor cells. Research has shown that some immunosaccharides primed macrophages for an enhanced respiratory burst, directly stimulated NO production via induction of nitric oxide synthase, and induced macrophages to secrete both inflammatory (IL-1, IL-6, TNF-alpha, and IL-12) and anti-inflammatory (IL-10) cytokines.29,41,43 Our results demonstrate that BFO-1 possesses immunoenhancement activity. However, the mechanisms of the immunoenhancement properties of BFO-1 are not clear and require further study to support the present findings.
Conclusions
In this study, we isolated BFO from burdock roots, and the bacterial endotoxin in BFO was removed by DEAE-cellulose column chromatography. Our data show that BFO-1 stimulated the production of NO by macrophages and improved acid phosphatase activity in peritoneal macrophages in vitro. Intragastric of BFO-1 significantly increased the phagocytic function of peritoneal macrophages and the production of NO and acid phosphatase in vivo. Moreover, intragastric pretreatment with BFO-1 significantly delayed tumor growth in S180 tumor-bearing mice, which confirmed that BFO-1 could promote the function of macrophages. These findings would be beneficial for the discovery of BFO-1 as a dietary supplement and functional food.
Conflicts of interest
The authors declare that they have no conflicts of interest.
Acknowledgements
This work was supported by Major Program of Shandong Province (No. 2015ZDJS04002), the Major State Basic Research Development Program of China (973 Program) (No. 2012CB822102) and the High Technology Research and Development Program of China (863 Program) (No. 2012AA021501).
References
- T. Yang, M. Jia, J. Meng, H. Wu and Q. Mei, Int. J. Biol. Macromol., 2006, 39, 179–184 CrossRef CAS PubMed.
- L. Guo, J. Liu, Y. Hu, D. Wang, Z. Li, J. Zhang, T. Qin, X. Liu, C. Liu, X. Zhao, Y. P. Fan, G. Han and T. L. Nguyen, Carbohydr. Polym., 2012, 90, 1055–1060 CrossRef CAS PubMed.
- Y. Chen, M.-Y. Xie, S.-P. Nie, C. Li and Y.-X. Wang, Food Chem., 2008, 107, 231–241 CrossRef CAS.
- M. C. Kang, S. Y. Kim, Y. T. Kim, E. A. Kim, S. H. Lee, S. C. Ko, W. A. Wijesinghe, K. W. Samarakoon, Y. S. Kim, J. H. Cho, H. S. Jang and Y. J. Jeon, Carbohydr. Polym., 2014, 99, 365–371 CrossRef CAS PubMed.
- T. M. A. Moro, C. M. Celegatti, A. P. A. Pereira, A. S. Lopes, D. F. Barbin, G. M. Pastore and M. T. P. S. Clerici, LWT, 2018, 90, 540–546 CrossRef CAS.
- X. Tian, S. Sui, J. Huang, J. P. Bai, T. S. Ren and Q. C. Zhao, Environ. Toxicol. Pharmacol., 2014, 38, 189–198 CrossRef CAS PubMed.
- Z. Zheng, X. Wang, P. Liu, M. Li, H. Dong and X. Qiao, Molecules, 2018, 23, 429 CrossRef PubMed.
- D. Tousch, L. P. Bidel, G. Cazals, K. Ferrare, J. Leroy, M. Faucanie, H. Chevassus, M. Tournier, A. D. Lajoix and J. Azay-Milhau, J. Agric. Food Chem., 2014, 62, 7738–7745 CrossRef CAS PubMed.
- G. Lee, Y. Son, Y. Jeon, H. Kang and I. Hwang, Korean J. Food Sci. Technol., 2015, 47, 336–344 CrossRef.
- W. S. Yang, S. R. Lee, Y. J. Jeong, D. W. Park, Y. M. Cho, H. M. Joo, I. Kim, Y. B. Seu, E. H. Sohn and S. C. Kang, J. Agric. Food Chem., 2016, 64, 3564–3573 CrossRef CAS PubMed.
- J. R. de Oliveira, R. B. de Aguiar Almeida, P. das Gracas Figueiredo Vilela, F. E. de Oliveira, R. F. da Rocha, A. O. Jorge and L. D. de Oliveira, Arch. Oral Biol., 2014, 59, 808–814 CrossRef PubMed.
- K.-Y. Ji, J.-H. Jang, E.-H. Lee, S.-M. Kim, H.-W. Song, W.-K. Yang, H.-Y. Kim, K.-H. Kim, Y.-S. Lee, D.-S. Kim, H.-S. Kang and S.-H. Kim, J. Funct. Foods, 2018, 45, 24–33 CrossRef CAS.
- J. M. F. Rodriguez, A. R. C. de Souza, R. L. Krüger, M. C. M. Bombardelli, C. S. Machado and M. L. Corazza, J. Supercrit. Fluids, 2018, 135, 25–33 CrossRef CAS.
- R. C. Fierascu, M. I. Georgiev, I. Fierascu, C. Ungureanu, S. M. Avramescu, A. Ortan, M. I. Georgescu, A. N. Sutan, A. Zanfirescu, C. E. Dinu-Pirvu, B. S. Velescu and V. Anuta, Food Chem. Toxicol., 2018, 111, 44–52 CrossRef CAS PubMed.
- Y. Gao, C. Gu, K. Wang, H. Wang, K. Ruan, Z. Xu and Y. Feng, Phytother. Res., 2018, 32, 631–642 CrossRef CAS PubMed.
- C. H. Yen, H. F. Chiu, S. Y. Huang, Y. Y. Lu, Y. C. Han, Y. C. Shen, K. Venkatakrishnan and C. K. Wang, Helicobacter, 2018, 23, e12469 CrossRef PubMed.
- F.-A. Chen, A.-B. Wu and C.-Y. Chen, Food Chem., 2004, 86, 479–484 CrossRef CAS.
- D. Li, J. M. Kim, Z. Jin and J. Zhou, Anaerobe, 2008, 14, 29–34 CrossRef CAS PubMed.
- W. S. Chung, A. W. Walker, P. Louis, J. Parkhill, J. Vermeiren, D. Bosscher, S. H. Duncan and H. J. Flint, BMC Biol., 2016, 14, 3 CrossRef PubMed.
- B. Mao, D. Li, J. Zhao, X. Liu, Z. Gu, Y. Q. Chen, H. Zhang and W. Chen, J. Agric. Food Chem., 2015, 63, 856–863 CrossRef CAS PubMed.
- C. J. Zheng, R. Liu, B. Xue, J. Luo, L. Gao, Y. Wang, S. Ou, S. Li and X. Peng, Food Funct., 2017, 8, 1925–1932 RSC.
- G. Flamm, W. Glinsmann, D. Kritchevsky, L. Prosky and M. Roberfroid, Crit. Rev. Food Sci. Nutr., 2001, 41, 353–362 CrossRef CAS PubMed.
- S. A. Kumar, L. C. Ward and L. Brown, Br. J. Nutr., 2016, 116, 1502–1511 CrossRef CAS PubMed.
- P. D. Schley and C. J. Field, Br. J. Nutr., 2007, 87, S221 CrossRef.
- M. Roberfroid, G. R. Gibson, L. Hoyles, A. L. McCartney, R. Rastall, I. Rowland, D. Wolvers, B. Watzl, H. Szajewska, B. Stahl, F. Guarner, F. Respondek, K. Whelan, V. Coxam, M. J. Davicco, L. Leotoing, Y. Wittrant, N. M. Delzenne, P. D. Cani, A. M. Neyrinck and A. Meheust, Br. J. Nutr., 2010, 104(suppl 2), S1–S63 CrossRef CAS PubMed.
- B. van Duuren-Stuurman, M. Grollers-Mulderij, A. van de Runstraat, A. Duisterwinkel, J. Terwoert and S. Spaan, Ann. Work Exposures Health, 2018, 62, 157–166 CrossRef CAS PubMed.
- P. Prasad, S. Sachan, S. Suman, G. Swayambhu and S. Gupta, Langmuir, 2018, 34, 7396–7403 CrossRef CAS PubMed.
- J. Tu, H. X. Sun and Y. P. Ye, J. Ethnopharmacol., 2008, 119, 266–271 CrossRef CAS PubMed.
- Z. Cai, W. Li, H. Wang, W. Yan, Y. Zhou, G. Wang, J. Cui and F. Wang, Int. J. Biol. Macromol., 2012, 51, 484–488 CrossRef CAS PubMed.
- G. Pang, J. Xie, Q. Chen and Z. Hu, Food Sci. Hum. Wellness, 2012, 1, 26–60 CrossRef.
- H. Kim, K.-W. Yu, H.-D. Hong and K.-S. Shin, J. Funct. Foods, 2017, 35, 384–390 CrossRef CAS.
- S. M. Poznanski, N. G. Barra, A. A. Ashkar and J. D. Schertzer, et al., Inflammation Res., 2018, 67, 813–828 CrossRef CAS PubMed.
- Z. Yu, M. Kong, P. Zhang, Q. Sun and K. Chen, Carbohydr. Polym., 2016, 148, 318–325 CrossRef CAS PubMed.
- L. Bai, L. Y. Zhu, B. S. Yang, L. J. Shi, Y. Liu, A. M. Jiang, L. L. Zhao, G. Song and T. F. Liu, Int. J. Biol. Macromol., 2012, 51, 705–709 CrossRef CAS PubMed.
- I. A. Schepetkin and M. T. Quinn, Int. Immunopharmacol., 2006, 6, 317–333 CrossRef CAS PubMed.
- L. L. R. Paredes, F. R. Smiderle, A. P. Santana-Filho, A. Kimura, M. Iacomini and G. L. Sassaki, Int. J. Biol. Macromol., 2018, 108, 1074–1081 CrossRef CAS PubMed.
- Y. Bai, P. Zhang, G. Chen, J. Cao, T. Huang and K. Chen, Int. Immunopharmacol., 2012, 12, 611–617 CrossRef CAS PubMed.
- Z. Wei, G. Chen, P. Zhang, L. Zhu, L. Zhang and K. Chen, Carbohydr. Polym., 2018, 198, 302–312 CrossRef CAS PubMed.
- H. Kim, B. S. Kwak, H. D. Hong, H. J. Suh and K. S. Shin, Int. J. Biol. Macromol., 2016, 87, 308–316 CrossRef CAS PubMed.
- M. A. Rahat and B. Hemmerlein, Front Physiol., 2013, 4, 144 CAS.
- I. V. Yegorenkova, A. A. Fomina, K. V. Tregubova, S. A. Konnova and V. V. Ignatov, Arch. Microbiol., 2018, 200, 1471–1480 CrossRef CAS PubMed.
- S. Saravanan, N. Prakash Babu, P. Pandikumar, M. Karunai Raj, M. Gabriel Paulraj and S. Ignacimuthu, J. Ethnopharmacol., 2012, 140, 239–246 CrossRef CAS PubMed.
- A. Nawaz, A. Bakhsh Javaid, S. Irshad, S. H. Hoseinifar and H. Xiong, Fish Shellfish Immunol., 2018, 76, 272–278 CrossRef CAS PubMed.
|
This journal is © The Royal Society of Chemistry 2019 |