DOI:
10.1039/C8RA10051A
(Paper)
RSC Adv., 2019,
9, 8628-8637
Retracted Article:
Miscanthus grass-derived carbon dots to selectively detect Fe3+ ions†
Received
6th December 2018
, Accepted 23rd January 2019
First published on 14th March 2019
Abstract
Novel fluorescent carbon dots (CDs) were synthesized using an economically feasible and green one-step heating process. Miscanthus, a perennial grass and an inexpensive sustainable biomass, was utilized as the starting material to prepare CDs and doped CDs (nitrogen, phosphorous and nitrogen-phosphorous dual doped). The abundance of oxygen-containing functional groups in Miscanthus-derived CDs (MCD) and doped MCD was confirmed via Fourier-transform infrared (FTIR) and energy dispersive X-ray spectroscopy (EDS). The average size of MCD, N-doped MCD, P-doped MCD and dual-doped MCDs was found to be 7.87 ± 0.27, 4.6 ± 0.21, 6.7 ± 0.38 and 5.3 ± 0.32 nm, respectively. The synthesized MCD and doped MCD exhibited a quantum yield (QY) of 4.71, 11.65, 2.33 and 9.63% for the MCD, N-doped MCD, P-doped MCD and dual-doped MCD, respectively. MCD and doped MCD exhibited excellent excitation-dependent photoluminescence properties, with strong blue fluorescence upon irradiation with UV-light (365 nm). N-doped MCD exhibited superb selectivity towards Fe3+ ions, with a detection limit of 20 nM and a detection range from 0.02 to 2000 μM. The normalized linear relationship between the intensity of fluorescence emission of the prepared N-doped MCD and the concentration of Fe3+ ions was utilized to selectively and sensitively detect Fe3+ ions.
1 Introduction
Fluorescent carbon dots (CDs) have generated attention in various fields such as optoelectronic devices,1 drug delivery,2 bioimaging,3,4 and biosensors,5etc. Their unique and fascinating properties, such as superb photostability, photoabsorption, photobleaching resistance, chemical resistance, biocompatibility, low toxicity, and excellent water solubility, make them appropriate for many advanced applications.6–9 Numerous top-down and bottom-up methods have been studied for CD synthesis.4,7 However, most of the top-down approaches are burdened by manufacturing difficulties, large-scale production expenses due to the use of costly equipment and tedious processes.10 In contrast, the bottom-up approaches are cost-effective, simple and environmentally friendly. Not only is the method of synthesis important, but the quantum yield (QY) of the CDs is also an important aspect of advanced applications of CDs. If proper functional groups are used for surface passivation and samples are doped with heteroatoms, then usually there is an enhancement in the QY of CDs.11,12 Of these two approaches, doping is the more promising technique, since it can efficiently tailor the inherent properties of CDs such as electronic properties, optical properties, etc. Recently, development of sustainable precursors for synthesis of nanomaterials has been of significant interest to replace conventional chemical feedstocks.
Many sustainable carbon-based starting materials have been investigated for CD synthesis because these feedstocks are environmentally friendly and economically feasible materials.13 In many cases, sustainable materials are derived from biobased precursors.14–19 Such carbon sources can be generalized under the following categories: fruits/vegetables, food/beverage wastes, animal/human derivatives and plant/agricultural biomass materials.13 The use of agricultural biomass and wastes has a positive impact on the environment as the materials repurpose waste into value-added products.20 Furthermore, the waste products can be obtained at little to no cost.21 Different wastes, such as used frying oil and kitchen waste, were used as potential precursor for CD synthesis, but the CDs obtained had a very low QY of 3.1%.22,23 Purkayastha et al.23 and Park et al.24 have used agricultural and food waste, respectively, to synthesize CDs. Park and his coworker successfully obtained 120 g of CD from 100 kg of food waste by a room temperature, ultrasonic-assisted method of synthesis.24 CDs were also synthesized from different vegetable wastes, like onion peel,25 cornstalks,21 pseudo banana stems,26etc. Paper wastes were also used to synthesize CDs via microwave-assisted pyrolysis, using ionic liquid as solvent.27 However, this work features the novel use of an agricultural residue.
Miscanthus, a perennial grass grown abundantly in Canada, is an environmentally sustainable carbon source.28Miscanthus is a biomass source which contains high-quality lignocellulosic material.29 To the best of our knowledge, there is no study on synthesis of CDs from Miscanthus grass. Furthermore, the material can be obtained cost effectively28 and would make a perfect starting material for the synthesis of CDs.
CDs have displayed good potential for use in metal ion sensing applications. Detection of Fe3+ ions is vital from the viewpoint of clinical research and for environmental concerns.30,31 Fe3+ ions serve a crucial role in biological systems as components of the cell metabolism, oxygen delivery systems, enzymatic co-factors, and electron transfer systems.32 In addition, iron is extensively used in industrial metal production, as it is one of the most abundant metals on the earth.10 Detection of metal ions via fluorescence probes possesses many advantages, including ease of operation, high selectivity and excellent sensitivity.21 In comparison to conventional fluorescent materials, which use a sensor probe, CDs demonstrate improved properties, including strong size and excitation-dependent photoluminescence (PL), excellent biocompatibility and low toxicity.22 Until now, many studies have been completed to explore the effective detection of Fe3+ using CDs as a fluorescent material. For example, CDs made from citric acid and tris(hydroxymethyl)aminomethane (as starting material) were synthesized by a hydrothermal method and showed high sensitivity for Fe3+ ions.33 In another study, folic acid-derived CDs also detected Fe3+ ions and the samples possessed a detection limit of 0–7.16 mM L−1.34 Qu et al.35 also found that dopamine-derived CDs had selective and sensitive detection towards Fe3+ ions, and samples possessed a detection limit of 0.32 μM L−1. Nitrogen-doped Magnolia liliiflora flower-derived CDs,36 and Chionanthus retusus fruit-derived CDs37 also showed good sensitivity towards Fe3+ ions. Even though extensive research has been conducted on CD synthesis, work is still required to make fluorescent probe materials with high photoluminescence QY and sensitivity for Fe3+ ions. Moreover, there is an urgent need for fluorescent materials which can provide a wide detection range and a low limit of detection.
In this work, we synthesized Miscanthus-derived CDs (MCDs) and doped MCDs by conventional heating methods to achieve a fluorescent material which can address the existing limitations. The chemical structure, optical properties and morphology of MCDs and doped MCDs were investigated by spectroscopic and microscopic techniques. In addition, Fe3+ detection efficiency and sensitivity of the as-synthesized MCDs and doped MCDs were evaluated and compared to find a suitable MCD-based fluorescent probe.
2 Methods and materials
2.1 Materials
Miscanthus grass fibers (<2 mm) were received from Competitive Green Technologies (Leamington, Ontario). Analytical grade chemicals: ethylenediamine (EDA, C2H4(NH2)2), ortho-phosphoric acid (PA, H3PO4), potassium hydroxide (KOH), iron(III) chloride (FeCl3), nickel(II) acetate tetrahydrate (Ni(CH3CO2)2·4H2O), cobalt(II) acetate tetrahydrate (Co(CH3CO2)2·4H2O) and quinine sulfate (C40H54N4O10S) were purchased from Sigma-Aldrich (Ontario, Canada). Deionized water (DI) was provided from on-site facilities at the University of Guelph (Ontario, Canada).
2.2 Preparation of Miscanthus extract
Miscanthus extract was prepared by placing 50 g of Miscanthus fibers in 700 mL DI water. This ratio of Miscanthus to water was the minimum amount required to completely emerge the fiber and maintain a fluidic consistency for stirring. The mixture was covered with aluminum foil and placed on a hot plate which was then heated to 80 °C and stirred at 500 rotations per min (rpm). After 1 hour of heating, the solution was vacuum filtrated. The recovered filtrate was used as a precursor for the CD synthesis by conventional heating methods.
2.3 Synthesis of Miscanthus-derived CDs (MCDs) and doped MCDs
MCDs were synthesized via a conventional heating method. Approximately 100 mL of Miscanthus extract was transferred to an Erlenmeyer flask and pH of the solution was adjusted to 11 using KOH aqueous solution (1 N). The flask was capped with a cotton plug and placed in an oven at 180 °C for 4 hours. Then, the flask was allowed to cool down and the dark brown dispersion of MCD was collected by filtration. After that, samples were neutralized and centrifuged at high speed to remove the insoluble and large particles (8000 rpm) for 10 minutes. Nitrogen-doped samples were synthesized with 100 mL of Miscanthus extract combined with 6 mL of EDA. Phosphorus-doped MCDs were synthesized by combining 100 mL of Miscanthus extract and 2 mL of PA. Lastly, dual-doped MCDs were synthesized with 6 mL of EDA and 2 mL of PA added to approximately 600 mL of Miscanthus extract and synthesized via conventional heating methods. The amounts of EDA and PA chosen for this study were based upon previous work by Liu et al.38 to prepare nitrogen- and phosphorous-doped CDs. The doped MCDs were also filtered, neutralized and centrifuged following the same method. A small amount of MCDs and doped MCDs were dried in the freeze drier for characterization with the remaining parts of the samples kept as aqueous dispersions.
2.4 Characterization of CDs
The ultraviolet-visible (UV-visible) spectra of MCDs and doped MCDs in aqueous solution were recorded on a Cary 300 spectrometer (Agilent Technologies, California, USA) over the range 200–500 nm. Fourier-transform infrared (FTIR) spectroscopy analysis was performed by a Nicolet 6700 (Thermo Scientific, Massachusetts, USA) over a small orbit diamond window with 164 scans and a resolution of 4 cm−1. A Phenom ProX scanning electron microscope (SEM) equipped with energy dispersive X-ray (EDS) was used to evaluate the elemental composition of MCDs and doped MCDs. The transmission electron microscopy (TEM) images were taken on a JEOL 2010F working at 200 keV. MCDs and doped MCDs were placed onto holey carbon TEM grids and dried at room temperature under vacuum before imaging. Furthermore, the Raman spectral analysis was performed via DXR™ 2 Raman microscope (Thermo Scientific, Massachusetts, USA) through a 50 μm pinhole at 10× magnification and laser wavelength of 532 nm. The X-ray diffraction (XRD) study was carried out at room temperature (ca. 25 °C) by a Rigaku Automated Powder X-ray Diffractometer with Cuκα irradiation (λ = 0.154 nm) over a range of 2θ = 10–70° at scan rate 1/min.
All fluorescent spectra were taken with a Cary Eclipse Spectrophotometer (Agilent Technologies, California, USA) at different excitation wavelengths (320, 350, 370, 390 and 410 nm) and varying pH values. Dilute 0.01 M KOH and 0.01 M PA were prepared and used to adjust the pH of MCD solutions. The QYs of MCD and doped MCD solutions were calculated using eqn (1),
| 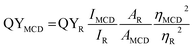 | (1) |
where intensity of fluorescence spectra, optical density at excited wavelength, and the refractive index of the used solvent (water) are represented by
I,
A and
η, respectively. Quinine sulfate in 0.1 M H
2SO
4 solution was used as the reference material. The subscript ‘R’ in
eqn (1) corresponds to quinine sulfate.
The detection of Fe3+ ions was carried out in aqueous solution at room temperature by measuring the quenching of fluorescence spectra with different concentrations of Fe3+ ions. The fluorescence spectra of other metal ion solutions (Co2+, Ni2+ and Mn2+) were also recorded in a similar way to confirm the selectivity for Fe3+ ions.
3 Results and discussion
3.1 Synthesis of MCD and doped MCDs
Miscanthus grass is a perennial herbaceous plant with lignocellulosic structure.39 Different-aged Miscanthus grasses contain cellulose (41.7–53.6%), lignin (20.1–23.8%), ash (3.57–6.30%), pentosans (20.0–25.3%), and extractives (2.8 to 5.7%).39,40 The aqueous extract of Miscanthus grass contained these extractives and soluble oligomers.41 The detailed possible mechanism for the formation of MCDs is shown in Scheme 1. The major steps during synthesis included polymerization, aromatization, nucleation, and growth of particles.42,43 Initially, the extractives and soluble oligomers reacted with each other through intermolecular dehydration under basic conditions. This aided in the formation of large-sized polymer nanoparticles. The polymer nanoparticles formed, continuously contracted due to further intramolecular dehydration. At this stage, aromatic clusters with a number of C
O and C
C groups formed inside the polymer nanoparticles. After reaching the critical supersaturation concentration, a nuclear burst of the aromatic clusters takes place to form the MCDs.27
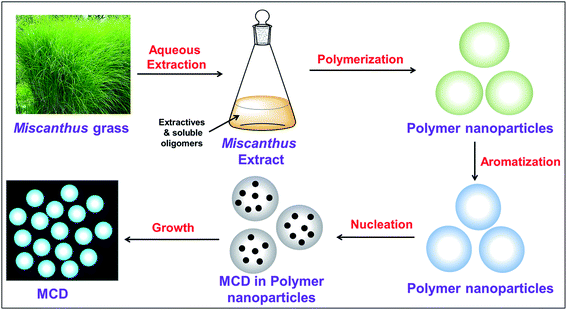 |
| Scheme 1 A proposed mechanism for the formation of MCDs. | |
3.2 Characterization of MCD and doped MCDs
The UV-visible spectra of MCD and doped MCD solutions showed a maximum absorption peak at 277–284 nm, as well as a prolonged tail in the visible region (Fig. 1). De et al.42 found a similar peak at 283 nm for banana extract-derived CDs. Peaks in this region occurred as a result of the n–π* transition of electrons for the C
O bond, in addition to the π–π* transition of electrons for C
C or C
N bonds, respectively.42 All the doped MCDs (N-doped, P-doped, and dual-doped) possessed two absorption peaks. The presence of a secondary peak was also found in N-doped samples prepared by Yuan et al.44 The second peak most likely resulted from samples trapping energy in the excited states via the surface states;45 which was previously reported in the literature and assigned to the n–π* transition of electrons in the carbogenic core.46
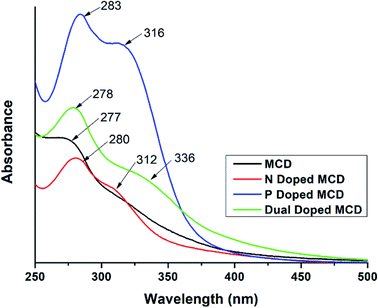 |
| Fig. 1 UV-visible spectra of MCDs and doped MCDs. | |
FTIR spectra were recorded to confirm the presence of specific functionality in the MCDs and doped MCDs (Fig. 2). The broad peak at 3274 cm−1 was due to the N–H stretching47 and vibrations of O–H groups.48 The smaller peak at 2930 cm−1 existed due to stretching of C–H groups.48 In the FTIR spectra obtained from MCDs, prominent peaks at 1683, 1126 and 1033 cm−1 were found for carbonyl (C
O), C–N and C–O stretching, respectively. Shi et al.21 also found similar kinds of FTIR peaks in cornstalk-based CDs. FTIR peaks for N-doped MCDs and dual-doped MCDs showed a strong NH2 scissoring peak at 1582 cm−1.44 This indicated the presence of amine groups in N-doped MCDs and dual-doped MCDs. Yuan et al.44 also noticed NH2 scissoring peaks at the same position for citric acid-based N-doped CDs. P-doped MCDs demonstrated a characteristic peak for the P–O–H stretching bond at 944 cm−1.38 This suggests successful doping in MCDs. Based on the peaks observed on the FTIR spectra from the MCD aqueous solutions, it can be deduced that the samples possessed various surface functionalities, including amine groups, hydroxyl, carbonyl and carboxylate groups.
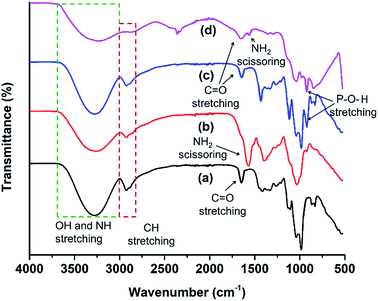 |
| Fig. 2 FTIR spectral analysis of (a) MCDs, (b) N-doped MCDs, (c) P-doped MCDs and (d) dual-doped MCDs. | |
In order to find out the elemental composition of MCDs and doped MCDs, the weight of the elements and the atomic ratios of the elements were determined from SEM/EDS data and are displayed in Table 1. The nitrogen content in N-doped MCDs was estimated to be ca. 28.8%, which indicated that N-doped MCDs were nitrogen rich in nature. The content of phosphorous in P-doped MCD was found to be ca. 7.4%. Also, significant amounts of phosphorous and nitrogen were found in dual-doped MCDs. This suggested successful doping of heteroatoms in the MCDs.
Table 1 EDS elemental compositions of MCDs (atomic%)
Sample |
Carbon |
Nitrogen |
Oxygen |
Phosphorus |
MCD |
43.1 ± 1.0 |
8.4 ± 0.9 |
46.5 ± 1.7 |
— |
N-doped MCD |
33.9 ± 9.4 |
28.8 ± 4.5 |
38.4 ± 9.4 |
— |
P-doped MCD |
32.2 ± 3.6 |
10.4 ± 5.9 |
50.0 ± 7.6 |
7.4 ± 1.8 |
Dual-doped MCD |
31.1 ± 2.5 |
18.2 ± 1.3 |
43.0 ± 1.6 |
7.7 ± 0.5 |
The XRD patterns of MCDs and doped MCDs displayed a broad peak at 2θ = 21.7 with a lattice space of 0.41 nm, which corresponded to the graphite lattice spacing of (002) (see Fig. 3a). The larger lattice space in comparison to graphite (0.34 nm) may be caused by the presence of an enormous amount of functional groups.15 The broad peak suggests that the synthesized MCDs and doped MCDs were moderately crystalline in nature.18
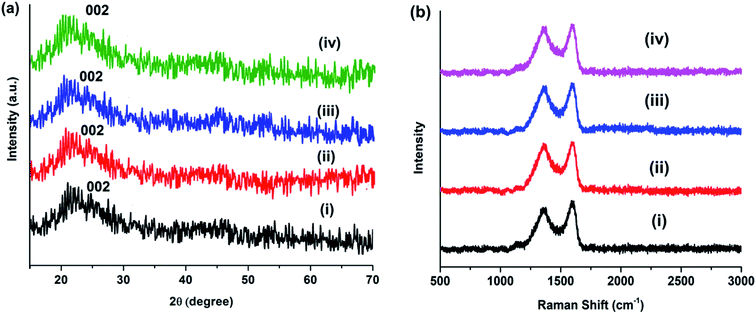 |
| Fig. 3 (a) XRD patterns and (b) Raman spectra of (i) MCDs, (ii) N-doped MCDs, (iii) P-doped MCDs and (iv) dual-doped MCDs. | |
Raman spectra of MCDs and doped MCDs are presented in Fig. 3b. Two Raman peaks were found at 1362 and 1598 cm−1, which were attributed to D- and G-band, respectively. The D-band and G-band correspond to defects and the E2g mode of graphitic structure, respectively.10 The position of the peak was unchanged after doping of MCDs; however, the intensity ratio (ID/IG) changed upon doping. The ID/IG for MCDs was measured and found to be 0.81; however, the value increased to 0.89 for N-doped MCDs. This indicated that the doping attached more functional groups and, hence, the defects in the structure increased.14,16
3.3 TEM analysis of MCDs and doped MCDs
The morphology and particle size of MCDs and doped MCDs were characterized and measured by TEM, as the photoluminescence (PL) properties of CDs are mostly dependent on particle size. The TEM images of MCDs and N-doped MCDs are shown in Fig. 4, which demonstrates that both MCDs and doped MCDs were mainly orbicular in their morphology and were distributed evenly without any agglomeration. The average size of MCDs, N-doped MCDs, P-doped MCDs and dual-doped MCDs was found to be 7.87 ± 0.27, 4.6 ± 0.21, 6.7 ± 0.38 and 5.3 ± 0.32 nm, respectively. This suggested that MCDs and N-doped MCDs may have wavelength-dependent PL properties. We further investigated the wavelength-dependent PL properties and these are discussed in the next section.
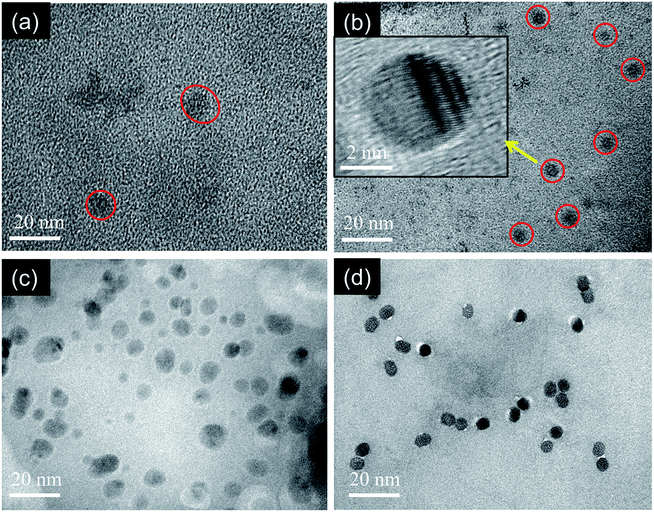 |
| Fig. 4 TEM images of the as-prepared (a) MCDs, (b) N-doped MCDs (inset showing high resolution TEM image), (c) P-doped MCDs and (d) dual-doped MCDs. | |
In the high resolution TEM image of the N-doped MCDs, the lattice fringes were clearly observed (Fig. 4b, inset). This further indicated the presence of graphitic layers in the N-doped MCD structure.
3.4 Optical properties of MCD and doped MCD
A thorough study on the fluorescence properties of MCDs and doped MCDs was carried out at regular intervals from 320 nm to 410 nm (for excitation wavelengths). The excitation-dependent emission spectra of MCDs and doped MCDs are shown in Fig. 5. The strength of the PL emission was calculated by the intensity of the peaks. The intensity of the samples was strongly dependent on the number of excited particles at the excitation wavelength.42 The synthesized MCDs and doped MCDs experienced excitation-dependent emission and a red-shift in emission peak values with increased excitation wavelength. The maximum emission wavelengths were found at 431 nm, 512 nm, 455 nm and 485 nm for MCDs, N-doped MCDs, P-doped MCDs and dual-doped MCDs, respectively. The N-doped MCDs exhibited a unique and systematic increase in PL properties. As the excitation wavelength increased, both the intensity and emission wavelengths increased. According to previous work by Ding et al.,48 the improvement in fluorescence of the samples was most likely attributed to nitrogen doping.
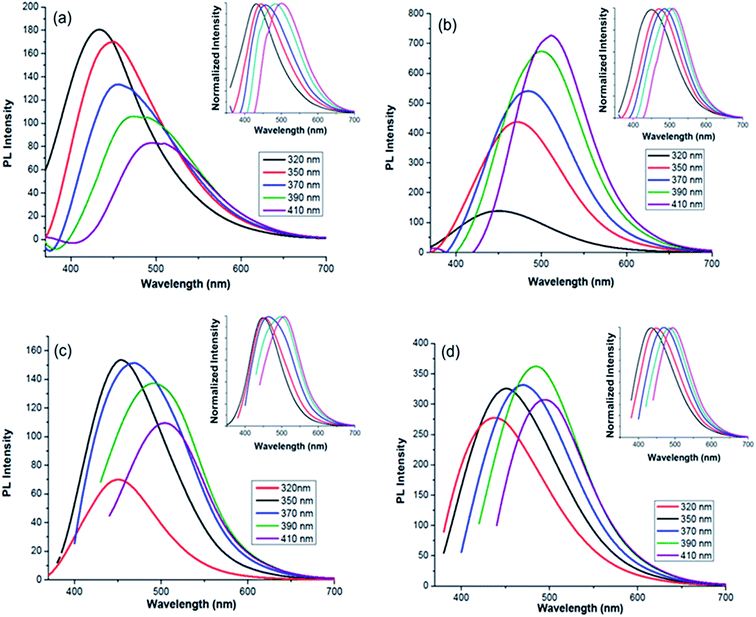 |
| Fig. 5 Photoluminescence (PL) spectra for: (a) MCDs; (b) N-doped MCDs; (c) P-doped MCDs; (d) dual-doped MCDs (insets showing excitation-dependent-normalized PL intensity). | |
P-doped MCDs experienced similar PL properties for excitation wavelengths of 350, 370 and 390 nm. The dual-doped MCDs also exhibited relatively similar intensities, with the strongest emission at 485 nm (390 nm excitation). Overall, these samples possessed good PL properties, especially N-doped MCDs which showed the strongest PL emission. According to literature, excitation-dependent PL properties are controlled by various factors, including: the carbogenic cores that are present in the samples, the surface states and the quantum effects possessed by all samples.48 The QYs of MCDs and doped MCDs were evaluated using a reference fluorescence material (quinine sulfate). QYs were found to be 4.71, 11.65, 2.33 and 9.63% for the MCDs, N-doped MCDs, P-doped MCDs and dual-doped MCDs, respectively. Since the N-doped MCDs demonstrated a high QY, it is suggested that there is potential use of these CDs as excellent fluorescent probe material.
3.5 pH-dependent PL properties
To investigate the pH-dependent PL emissions of the MCDs and doped MCDs, the fluorescence spectra of MCDs with different pH values, ranging from 1 to 12, were taken and are shown in Fig. 6. The excitation wavelength was chosen based on achieving optimal fluorescence during the previous analysis (Fig. 6). A full range of the pH scale was studied to determine the influence of acidic and basic environments on all the prepared samples. It is suggested that this work may increase the applications of MCDs in pH sensing applications. Furthermore, it was quite interesting to find that both acidic and basic environments resulted in fluorescence quenching. Fluorescence quenching has been used to describe the decrease in PL properties as a result of the changed environment for the CDs.49 The PL intensity was higher under neutral conditions, while decreasing significantly in either strongly acidic or basic solutions. This indicated that the MCDs and doped MCDs were sensitive to acid–base conditions. The PL spectra shifted towards higher wavelengths after changing the pH and this phenomenon was most pronounced under alkaline conditions (Fig. 6).
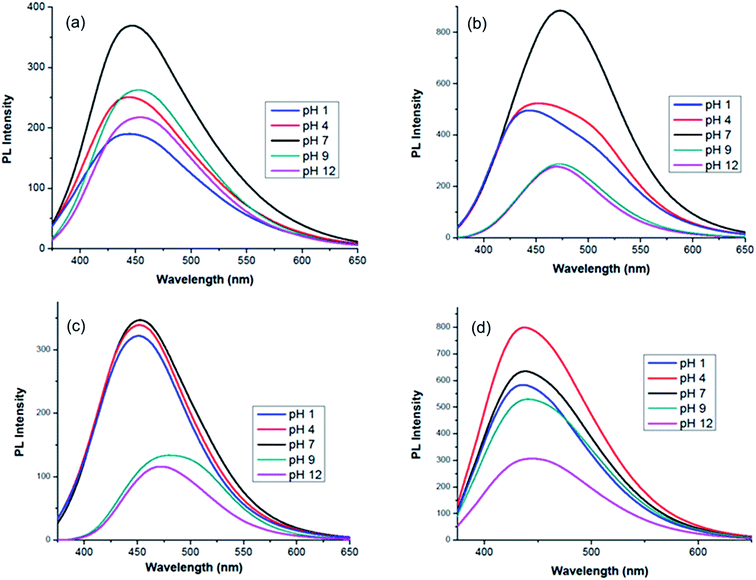 |
| Fig. 6 pH-dependent photoluminescence spectra of: (a) MCDs; (b) N-doped MCDs; (c) P-doped MCDs; and (d) dual-doped MCDs. | |
The change in PL emission was attributed to protonation of functional groups (hydroxyl/carboxyl) in acidic conditions and deprotonation in basic conditions.46,49 According to research, under neutral conditions the carboxylic groups were more likely to exist without the hydrogen attached, since the pKa is between 4 and 5, whereas the pKa value for the hydroxyl group is between 8 and 10.46 Due to the newly generated surface state, MCDs displayed PL emissions at longer wavelengths under alkaline conditions. In doped MCD samples, it was found that significant quenching occurred in alkaline pH. The performance of these samples may be attributed to the different degrees of deprotonation in the doped MCDs at alkaline pH.
3.6 Application of the MCDs for Fe3+ detection
To find the selective fluorescence-sensing efficiency of the synthesized MCDs and doped MCDs towards Fe3+ ions, the quenching properties were obtained in the presence of different metal ions (Co2+, Ni2+, and Mn2+), each with a concentration of 100 μM (Fig. 7). In Fig. 7, it is clearly seen that the MCDs and N-doped MCDs possess excellent sensitivity and selectivity for Fe3+ ions, especially N-doped MCDs. In the case of N-doped MCDs, the fluorescence intensity remained almost unchanged after the addition of other metal ions (Co2+, Ni2+ and Mn2+).
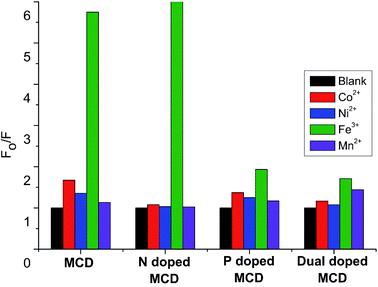 |
| Fig. 7 Selectivity of the MCDs and doped MCDs for Fe3+ detection. | |
In contrast, the fluorescence intensity of the MCDs was slightly changed by the presence of Co2+ and Ni2+. However, P-doped MCDs and dual-doped MCDs showed no significant influence on the PL properties after addition of metal ions. This indicated that N-doped MCDs were most suitable for sensing Fe3+ ions over the other synthesized MCDs.
The PL intensity of the N-doped MCDs progressively reduced after addition of Fe3+ ions over the concentration range of 10 μM to 2 mM (Fig. 8). The remarkable quenching of PL intensities of N-doped MCDs may have resulted from strong bonding affinity between Fe3+ ions and N-doped MCDs. The Fe3+ ions could coordinate with amino and carboxyl groups which are present in the N-doped MCDs (as confirmed in the FTIR spectra as well).50 The radiative transition was interrupted; and this led to quenching of fluorescence emission.
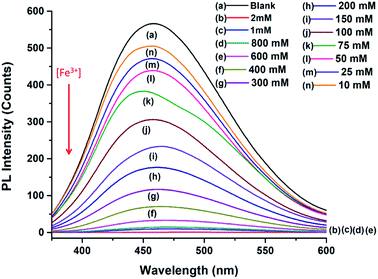 |
| Fig. 8 The PL emission spectra of N-doped MCDs after the addition of Fe3+ ions. | |
In Fig. 9a, the relationship between the Fe3+ ion concentration and PL intensity is demonstrated. The fluorescence intensity was almost quenched (fluorescence change ratio ∼98%) after adding 1 mM Fe3+ ions. The relationship between the Fe3+ ion concentration and the (F0 − F)/F0 value (F is the fluorescence intensity after metal ions are added and F0 is the fluorescence intensity without metal ions) is shown in Fig. 9b. A linear correlation was found for the lower concentrations (<200 μM), whereas the curve became saturated at high concentrations of Fe3+ ions. Fluorescence emission of N-doped MCDs was completely quenched after adding 2 mM Fe3+ ions. The results clearly reflect that N-doped MCDs possess a wide detection range which could be beneficial for selective Fe3+ ion detection.
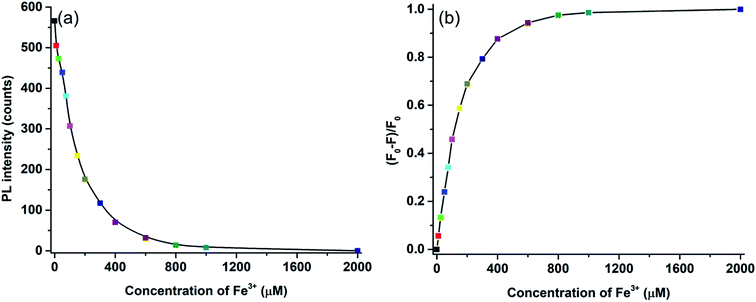 |
| Fig. 9 (a) The relationship between Fe3+ ion concentrations and fluorescence intensity, and (b) Stern–Volmer plot of fluorescence quenching for N-doped MCDs after addition of Fe3+ ions. | |
The intensity of the fluorescence spectra for N-doped MCDs was measured in the presence of Fe3+ concentrations over the range 10–100 μM to determine the limit of detection (LOD) (Fig. 10). The (F0 − F)/F0 value was plotted against Fe3+ ion concentration; a good linear relationship was determined between both variables. The LOD was found to be 0.02 μM based on eqn (2).
|  | (2) |
where
σ represents the standard deviation of the PL intensities and S represents the slope of the linear calibration plot shown.
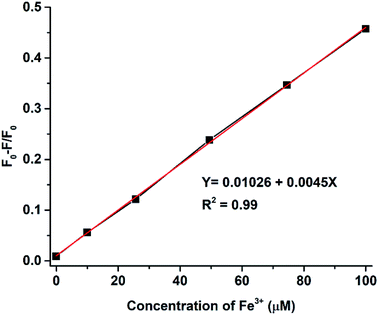 |
| Fig. 10 The linear calibration graph for (F0/F)/F0 with different Fe3+ ion concentrations. | |
The Stern–Volmer constant, K, was determined using eqn (3) and adapted from the literature.51,52
|  | (3) |
where
F and
F0 are the fluorescence intensities without and with the presence of Fe
3+, respectively.
K is the quenching constant and [Q] is the concentration of the quencher. Due to the linear nature of
Fig. 10, the literature suggested only one type of quenching occurred.
53K was found to be 7.1 × 10
4 M
−1 which, according to literature, is attributed to static quenching.
54
Static quenching was in response to the formation of a non-fluorescent fluorophore while the CDs were in their ground state.51 In fact, Scheme 2 shows the possible fluorescence quenching mechanism of N-doped MCDs by Fe3+ ions. The presence of polar functional groups, including hydroxyl, amine and carboxylic, in N-doped MCDs was confirmed by FTIR spectroscopy. These functional groups could form a complex with the Fe3+ ions.30 Therefore, the electron or energy was transferred between Fe3+ ions and N-doped MCDs.11 The non-radiative energy or electron transfer may be the reason for the quenched fluorescence of MCDs in the presence of Fe3+.
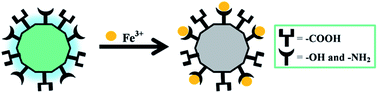 |
| Scheme 2 Schematic illustration of a possible fluorescence-quenching mechanism. | |
Furthermore, we compared the reported CDs and carbon-related materials for detection of Fe3+ (Table S1 in ESI†). It was determined that the N-doped MCDs exhibited comparable or even better performance, including wider linear range and LOD (0.02 μM), than those previously reported materials for the detection of Fe3+ ions.
The results clearly indicated that the N-doped MCDs possessed a wide range of detection capability of Fe3+ ions which may be valuable for the sensitive, selective and label-free Fe3+ ion detection from industrial waste and biological applications.
4 Conclusions
A simple and scalable one-pot technique was adopted to synthesize highly fluorescent MCDs and doped MCDs using an inexpensive and readily available sustainable biomass (Miscanthus grass). Nitrogen and phosphorous were successfully doped into the MCD frameworks, as was revealed by FTIR spectroscopy. All the synthesized MCDs and doped MCDs exhibited excitation-dependent PL emission behavior, as well as stable and strong fluorescence, without any surface modification or passivation. N-doped MCDs demonstrated a high QY (11.65%) and excellent excitation-dependent and pH-dependent PL emission. The synthesized N-doped MCDs showed superb selectivity and sensitivity to detect low concentrations of Fe3+ ions. In addition, the N-doped MCDs demonstrated a wide range of detection abilities (0.02 to 2000 μM) with a low limit of detection (20 nM), which could be valuable for the selective detection of Fe3+ ions from biological systems as well as in industrial waste. We believe that the proposed MCD sensors will be more beneficial for further advances.
Conflicts of interest
There are no conflicts to declare.
Acknowledgements
The authors would like to thank the following for their financial support: Ontario Ministry of Agriculture, Food and Rural Affairs (OMAFRA)/University of Guelph – Bioeconomy for Industrial Uses Research Program (Project # 030332); Natural Sciences and Engineering Research Council (NSERC), Canada Discovery Grants Project # 400320 and 401111; and the Ontario Research Fund, Research Excellence Program; Round-7 (ORF-RE07) from the Ontario Ministry of Research, Innovation and Science (MRIS) (Project # 052644 and 052665).
Notes and references
- X. M. Li, M. C. Rui, J. Z. Song, Z. H. Shen and H. B. Zeng, Adv. Funct. Mater., 2015, 25, 4929–4947 CrossRef CAS.
- A. R. Chowdhuri, T. Singh, S. K. Ghosh and S. K. Sahu, ACS Appl. Mater. Interfaces, 2016, 8, 16573–16583 CrossRef CAS.
- J. Liao, Z. H. Cheng and L. Zhou, ACS Sustainable Chem. Eng., 2016, 4, 3053–3061 CrossRef CAS.
- P. Miao, K. Han, Y. G. Tang, B. D. Wang, T. Lin and W. B. Cheng, Nanoscale, 2015, 7, 1586–1595 RSC.
- Y. H. Liu, W. X. Duan, W. Song, J. J. Liu, C. L. Ren, J. Wu, D. Liu and H. L. Chen, ACS Appl. Mater. Interfaces, 2017, 9, 12663–12672 CrossRef CAS.
- S. Sarkar, K. Das and P. K. Das, ACS Sustainable Chem. Eng., 2017, 5, 8356–8369 CrossRef CAS.
- H. T. Li, Z. H. Kang, Y. Liu and S. T. Lee, J. Mater. Chem., 2012, 22, 24230–24253 RSC.
- S. Y. Park, H. U. Lee, E. S. Park, S. C. Lee, J. W. Lee, S. W. Jeong, C. H. Kim, Y. C. Lee, Y. S. Huh and J. Lee, ACS Appl. Mater. Interfaces, 2014, 6, 3365–3370 CrossRef CAS.
- P. Roy, P. C. Chen, A. P. Periasamy, Y. N. Chen and H. T. Chang, Mater. Today, 2015, 18, 447–458 CrossRef CAS.
- R. Atchudan, T. N. J. I. Edison, K. R. Aseer, S. Perumal, N. Karthik and Y. R. Lee, Biosens. Bioelectron., 2018, 99, 303–311 CrossRef CAS.
- Z. G. Wang, P. Long, Y. Y. Feng, C. Q. Qin and W. Feng, RSC Adv., 2017, 7, 2810–2816 RSC.
- H. F. Liu, Z. H. Li, Y. Q. Sun, X. Geng, Y. L. Hu, H. M. Meng, J. Ge and L. B. Qu, Sci. Rep., 2018, 8, 1086 CrossRef.
- K. Jiang, Y. Wang, X. Gao, C. Cai and H. Lin, Angew. Chem., 2018, 57, 6216–6220 CrossRef CAS.
- T. N. J. I. Edison, R. Atchudan, M. G. Sethuraman, J. J. Shim and Y. R. Lee, J. Photochem. Photobiol., B, 2016, 161, 154–161 CrossRef CAS.
- R. Atchudan, T. N. J. I. Edison and Y. R. Lee, J. Colloid Interface Sci., 2016, 482, 8–18 CrossRef CAS.
- R. Atchudan, T. N. J. I. Edison, S. Perumal, R. Vinodh and Y. R. Lee, J. Alloys Compd., 2018, 766, 12–24 CrossRef CAS.
- V. Arul, T. N. J. I. Edison, Y. R. Lee and M. G. Sethuraman, J. Photochem. Photobiol., B, 2017, 168, 142–148 CrossRef CAS.
- T. N. J. I. Edison, R. Atchudan, J. J. Shim, S. Kalimuthu, B. C. Ahn and Y. R. Lee, J. Photochem. Photobiol., B, 2016, 158, 235–242 CrossRef CAS PubMed.
- R. Atchudan, T. N. Jebakumar Immanuel Edison, S. Perumal and Y. R. Lee, ACS Omega, 2018, 3, 17590–17601 CrossRef CAS.
- A.-M. Alam, B.-Y. Park, Z. K. Ghouri, M. Park and H.-Y. Kim, Green Chem., 2015, 17, 3791–3797 RSC.
- J. Shi, G. Ni, J. Tu, X. Jin and J. Peng, J. Nanopart. Res., 2017, 19, 209 CrossRef.
- J. Xu, T. Lai, Z. Feng, X. Weng and C. Huang, Luminescence, 2015, 30, 420–424 CrossRef CAS.
- M. Das Purkayastha, A. K. Manhar, V. K. Das, A. Borah, M. Mandal, A. J. Thakur and C. L. Mahanta, J. Agric. Food Chem., 2014, 62, 4509–4520 CrossRef CAS PubMed.
- S. Y. Park, H. U. Lee, E. S. Park, S. C. Lee, J.-W. Lee, S. W. Jeong, C. H. Kim, Y.-C. Lee, Y. S. Huh and J. Lee, ACS Appl. Mater. Interfaces, 2014, 6, 3365–3370 CrossRef CAS.
- K. Bankoti, A. P. Rameshbabu, S. Datta, B. Das, A. Mitra and S. Dhara, J. Mater. Chem. B, 2017, 5, 6579–6592 RSC.
- S. A. A. Vandarkuzhali, V. Jeyalakshmi, G. Sivaraman, S. Singaravadivel, K. R. Krishnamurthy and B. Viswanathan, Sens. Actuators, B, 2017, 252, 894–900 CrossRef CAS.
- Y. Jeong, K. Moon, S. Jeong, W.-G. Koh and K. Lee, ACS Sustainable Chem. Eng., 2018, 6, 4510–4515 CrossRef CAS.
- I. Major, J.-M. Pin, E. Behazin, A. Rodriguez-Uribe, M. Misra and A. Mohanty, Green Chem., 2018, 20, 2269–2278 RSC.
- E. Behazin, M. Misra and A. K. Mohanty, Composites, Part B, 2017, 118, 116–124 CrossRef CAS.
- X. B. Cui, Y. L. Wang, J. Liu, Q. Y. Yang, B. Zhang, Y. Gao, Y. Wang and G. Y. Lu, Sens. Actuators, B, 2017, 242, 1272–1280 CrossRef CAS.
- R. Yang, X. F. Guo, L. H. Jia, Y. Zhang, Z. L. Zhao and F. Lonshakov, Appl. Surf. Sci., 2017, 423, 426–432 CrossRef CAS.
- S. H. Li, Y. C. Li, J. Cao, J. Zhu, L. Z. Fan and X. H. Li, Anal. Chem., 2014, 86, 10201–10207 CrossRef CAS.
- M. Zhou, Z. L. Zhou, A. H. Gong, Y. Zhang and Q. J. Li, Talanta, 2015, 143, 107–113 CrossRef CAS.
- C. Shen, Y. P. Sun, J. Wang and Y. Lu, Nanoscale, 2014, 6, 9139–9147 RSC.
- K. G. Qu, J. S. Wang, J. S. Ren and X. G. Qu, Chem.–Eur. J., 2013, 19, 7243–7249 CrossRef CAS PubMed.
- R. Atchudan, T. N. J. I. Edison, K. R. Aseer, S. Perumal and Y. R. Lee, Colloids Surf., B, 2018, 169, 321–328 CrossRef CAS.
- R. Atchudan, T. N. J. I. Edison, D. Chakradhar, S. Perumal, J. J. Shim and Y. R. Lee, Sens. Actuators, B, 2017, 246, 497–509 CrossRef CAS.
- Y. Liu, X. J. Gong, W. J. Dong, R. X. Zhou, S. M. Shuang and C. Dong, Talanta, 2018, 183, 61–69 CrossRef CAS.
- Y. A. Gismatulina and V. V. Budaeva, Ind. Crops Prod., 2017, 109, 227–232 CrossRef CAS.
- E. M. Hodgson, D. J. Nowakowski, I. Shield, A. Riche, A. V. Bridgwater, J. C. Clifton-Brown and I. S. Donnisona, Bioresour. Technol., 2011, 102, 3411–3418 CrossRef CAS.
- K. T. Wang, C. Y. Jing, C. Wood, A. Nagardeolekar, N. Kohan, P. Dongre, T. E. Amidon and B. M. Bujanovic, Energies, 2018, 11, 39 CrossRef.
- B. De and N. Karak, RSC Adv., 2013, 3, 8286–8290 RSC.
- Y. P. Hu, J. Yang, J. W. Tian and J. S. Yu, J. Mater. Chem. B, 2015, 3, 5608–5614 RSC.
- Y. H. Yuan, Z. X. Liu, R. S. Li, H. Y. Zou, M. Lin, H. Liu and C. Z. Huang, Nanoscale, 2016, 8, 6770–6776 RSC.
- Y. Q. Dong, H. C. Pang, H. B. Yang, C. X. Guo, J. W. Shao, Y. W. Chi, C. M. Li and T. Yu, Angew. Chem., Int. Ed., 2013, 52, 7800–7804 CrossRef CAS PubMed.
- S. D. Choudhury, J. M. Chethodil, P. M. Gharat, P. K. Praseetha and H. Pal, J. Phys. Chem. Lett., 2017, 8, 1389–1395 CrossRef PubMed.
- W. J. Liu, C. Li, X. B. Sun, W. Pan and J. P. Wang, Sens. Actuators, B, 2017, 244, 441–449 CrossRef CAS.
- H. Ding, J. S. Wei and H. M. Xiong, Nanoscale, 2014, 6, 13817–13823 RSC.
- S. J. Zhu, Y. B. Song, X. H. Zhao, J. R. Shao, J. H. Zhang and B. Yang, Nano Res., 2015, 8, 355–381 CrossRef CAS.
- M. Shamsipur, K. Molaei, F. Molaabasi, M. Alipour, N. Alizadeh, S. Hosseinkhani and M. Hosseini, Talanta, 2018, 183, 122–130 CrossRef CAS.
- L. K. Fraiji, D. M. Hayes and T. C. Werner, J. Chem. Educ., 1992, 69, 424–428 CrossRef CAS.
- Y. B. Song, S. J. Zhu, S. Y. Xiang, X. H. Zhao, J. H. Zhang, H. Zhang, Y. Fu and B. Yang, Nanoscale, 2014, 6, 4676–4682 RSC.
- F. W. J. Teale, Nature, 1984, 307, 486 CrossRef.
- J. F. Shangguan, J. Huang, D. G. He, X. X. He, K. M. Wang, R. Z. Ye, X. Yang, T. P. Qing and J. L. Tang, Anal. Chem., 2017, 89, 7477–7484 CrossRef CAS.
Footnote |
† Electronic supplementary information (ESI) available. See DOI: 10.1039/c8ra10051a |
|
This journal is © The Royal Society of Chemistry 2019 |
Click here to see how this site uses Cookies. View our privacy policy here.