DOI:
10.1039/C8RA09735F
(Paper)
RSC Adv., 2019,
9, 1029-1035
Structure, tunable luminescence and energy transfer in Tb3+ and Eu3+ codoped Ba3InB9O18 phosphors
Received
27th November 2018
, Accepted 12th December 2018
First published on 9th January 2019
Abstract
The borate Ba3InB9O18 (BIBO) is a promising host material for phosphors. A series of Tb3+ and Eu3+ codoped Ba3InB9O18 phosphors were synthesized. Based on the Rietveld method, structure refinement of the codoped BIBO phosphor was carried out. Then, the luminescence properties of BIBO:Tb3+, Eu3+ phosphors were extensively investigated under ultraviolet (UV) and vacuum ultraviolet (VUV) excitation. The measured PL spectra and decay times evidenced that energy transfer occurs between the Tb3+ and Eu3+ ions. The energy-transfer mechanism from Tb3+ to Eu3+ in Ba3InB9O18 is dominated by electric multipolar interactions, with the critical distance calculated to be 10.97 Å. The temperature sensitivity of the Tb3+ and Eu3+ codoped sample under VUV was also investigated at the low temperature range from 25 K to 298 K. The emission color could be tuned from green to the red region by adjusting the concentration of codoped ions. The results indicate that the BIBO-based phosphors are valuable candidates for applications in the display and lighting fields.
1. Introduction
Owing to their critical significance in modern lighting and display fields, such as white-light emitting diodes, vacuum fluorescent displays, cathode ray tubes, plasma display panels, X-ray imaging scintillators and field emission displays, rare earth ion-doped phosphors have attracted tremendous attention with the prevalence and boom of the electronics technology over the past few decades.1–3 Lacking a specific color phosphor in use has prompted more investigations to be focused on full color phosphors. Fortunately, great attention has been paid to multicolor tunable luminescence due to its potential application in the fields of plasma display panels, full color displays, light emitting diodes, and field emission displays.4,5
Borate is regarded as a dramatic host material for phosphors because of its various crystal structures, simple process of composition, and steady physicochemistry properties.6,7 In 2008, Cai et al. discovered a novel barium indium borate Ba3InB9O18 (BIBO), which could be used as an X-ray detector due to its superior scintillation character.8 The crystal structure of the BIBO compound was crystalline in the hexagonal centric structure of the P63/m space group, which can be viewed as a layered-type structure constructed by discrete planar hexagonal [B3O6]3− rings. The [B3O6]3− rings stack parallel or anti-parallel to each other along the c-axis. Also, layers connected with deformed BaO6 hexagons are interleaved with regular InO6 octahedra and BaO9 polyhedra.8 In the rare-earth family, Tb3+ is well-known as a green emitting activator due to its predominant 5D4–7F5 transition peak at around 545 nm.9 Meanwhile, the Eu3+ ion is considered one of the most frequently useful red emitters in rare-earth-ions-doped materials due to its 5D0–7F2 transition.10 Up to now, single Eu3+- or single Tb3+-doped layered-type BIBO polycrystalline structures have been investigated under UV excitation, and have shown potential applications in an illumination area due to their relatively simple preparation, intense luminescence, and large quenching concentration.11 On the other hand, it has been recognized that the luminescence intensities of rare-earth ions can be enhanced or quenched by the energy transfer from other codoped rare-earth ions.12,13 Moreover, Eu3+ and Tb3+ usually play the critical roles of energy transfer in displays and lightings, implying that Tb3+ can act as a good sensitizer to enhance the luminescence efficiency of Eu3+ in many host materials, such as LiSrBO3, Sr3LaNa(PO4)3F, and Y4Si2O7N2.14–16 However, to the best of our knowledge, there are no reports about the photoluminescence (PL) and photoluminescence excitation (PLE) properties and the energy transfer of Tb3+ and Eu3+ in the BIBO host.
In this work, single-phase tunable green-yellow-red emitting phosphors, Tb3+ and Eu3+ codoped BIBO, were synthesized for the first time. The structure parameters and rare-earth ions occupancy were confirmed by the Rietveld refinement method. Then the luminescence properties were extensively investigated, including the photoluminescence under UV and VUV excitation, low temperature VUV spectroscopy, decay curve, and chromaticity diagram. In addition, the energy-transfer mechanism between Tb3+ and Eu3+ ions is also discussed herein.
2. Experimental section
A series of phosphors, Ba3In0.9−xB9O18:xTb3+, 0.1Eu3+ (x = 0, 0.02, 0.05, 0.08, 0.12, 0.15, 0.18 mol) and Ba3In0.92−yB9O18:0.08Tb3+, yEu3+ (y = 0, 0.05, 0.10, 0.15, 0.20, 0.25, 0.28 mol), were synthesized via standard high-temperature solid-state reaction methods. Stoichiometric mixtures of BaCO3 (spectral reagent), In2O3 (analytical reagent), Eu2O3 (spectral reagent), Tb4O7 (spectral reagent), and H3BO3 (analytical reagent) were finely ground into powders in an agate mortar. The excess H3BO3 of 3% mol was used for compensation during the process of synthesis because of the volatilization of boron oxide at high temperature. Then, the mixtures were preheated in platinum crucibles at 650 °C for 12 h to obtain the precursors. Subsequently, the precursors underwent calcination at 980 °C for 48 h with an intermediate grinding. Finally, the obtained products were ground into homogeneous powders for the following analysis. X-ray powder diffraction data were recorded on an X-ray diffractometer (Rigaku D/Max-2500) with Cu Kα radiation and a diffracted-beam graphite monochromator operated at a power of 40 kV and 250 mA. Rietveld refinement of the structure was carried out by using the Full-Prof_suite program.17 UV-visible diffuse reflectance (UV-DR) spectra were measured on a UV-visible spectrophotometer (Shimadzu, UV-2600) equipped with an integration sphere using BaSO4 as a reference. The photoluminescence emission (PL) and excitation (PLE) spectra of Tb3+ and Eu3+ codoped BIBO under ultraviolet (UV) radiation were obtained on a Hitachi F-7000 florescence spectrophotometer equipped with a Xe lamp as the excitation source. The luminescence spectra under the vacuum ultraviolet (VUV) region were measured at Beam line 3B1B at the Beijing Synchrotron Radiation Facilities (BSRF) under normal operating conditions (220 mA). The VUV excitation spectrum was corrected by sodium salicylate since its quantum efficiency was almost constant in the region. Photoluminescence decay curves were obtained using a fluorescence spectrometer (Edinburgh, FLS-920) with a μF900 flash lamp as the excitation source. The CIE coordinates were calculated by using the PL data based on the CIE 1931 standard colorimetric system.
3. Results and discussion
3.1 Phase identification and crystal structure
The XRD patterns of the as-synthesized BIBO:xTb3+, 0.1Eu3+ and BIBO:0.08Tb3+, yEu3+ phosphors are shown in Fig. 1, respectively. As seen in Fig. 1a, all the patterns of the single-doped and codoped BIBO samples are consistent with the standard pattern of Ba3InB9O18 (ICSD #245820) and no impurities were observed. For BIBO:xTb3+, 0.1Eu3+, a pure phase of Ba3InB9O18 (ICSD #245820) could be identified when Tb3+ varies in the range of 0–0.18 mol. Moreover, when the x value was increased, the main diffraction peaks (−120) and (014) at around 25–26° are shifted markedly toward a lower angle, indicating a lattice expansion. This should be ascribed to the larger Tb3+ (r = 0.92 Å) substituting the smaller In3+ (r = 0.80 Å) in the Ba3InB9O18 host lattice.18 As illustrated in Fig. 1b, the measured lattice parameters, a, c, and V, increase linearly and obey Vegard's law. In addition, as shown in Fig. 1c, for BIBO:0.08Tb3+, yEu3+, no impurity phase was yet detected when the Eu3+ concentration was raised from 0 to 0.28 mol. As presented in Fig. 1d, by increasing the Eu3+ concentration, the lattice parameters of BIBO:0.08Tb3+, yEu3+ also exhibit a near-linear expansion due to the substitution of smaller In3+ (r = 0.80 Å) by larger Eu3+ (r = 0.95 Å) ions.18
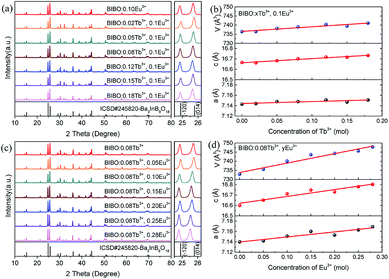 |
| Fig. 1 (a) XRD patterns and (b) lattice parameters of BIBO:xTb3+, 0.1Eu3+, (c) XRD patterns, and (d) lattice parameters of BIBO:0.08Tb3+, yEu3+ samples. | |
Rietveld refinement is a powerful method to investigate the crystal structure and the distribution of ions in crystal lattices. In order to understand the occupancies of the RE ions in the codoped BIBO phosphors, the structure refinement of BIBO:0.08Tb3+, 0.25Eu3+ was performed using the Full-Prof_suite program. The crystal structure of nondoped BIBO was considered as the initial structure, with the corresponding refined pattern given in Fig. 2a. In the figure, the experimental pattern, the calculated pattern, and the difference plot are expressed by small red dots, a solid black line, and a solid blue line at the bottom, respectively. Also, the positions of the calculated Bragg reflections are marked by the vertical bars. During the refinement, a total of 43 parameters were refined, including 26 structural parameters and 17 profile parameters. The refined crystallographic parameters and reliability factors are listed in Table 1. The primary reliability factors in the structural refinement converged to RB = 7.38%, RP = 5.79%, RWP = 7.76%, and S = 2.60, indicating that the determined structure should be reasonably accepted. The codoped BIBO:0.08Tb3+, 0.25Eu3+ was crystallized in the space group P63/m and in a hexagonal symmetry. The lattice parameter (a, c) and lattice volume (V) of the codoped sample were 7.1600(3) Å, 16.8123(4) Å, and 746.43(3) Å3, respectively. The structural parameters including the atomic coordinates, atomic occupancies, and isotropic displacement are shown in Table 2. The crystal structure of Ba3InB9O18:0.08Tb3+, 0.25Eu3+ was formed by the stable stacking arrangement of B3O6, BaO6, InO6, and BaO9 modules, with B3O6 layers connected by barium and indium atoms, as shown in Fig. 2b and c. The codoped Tb3+ and Eu3+ ions were demonstrated to substitute the In3+ sites in the host of BIBO. In addition, the Tb3+ and Eu3+ ions sites were verified by the actual experiments. We synthesized many samples with the codoped rare-earth ions occupying different cations. Using the XRD analysis and the index calculations, it was eventually found that Tb3+ and Eu3+ ions could not enter into the lattice of Ba2+ (r = 1.34 Å), whereas they could substitute the In3+ ions. This may attributed to the closed ionic radius and coordination of Eu3+ (r = 0.95 Å), Tb3+ (r = 0.92 Å), and In3+ (r = 0.80 Å) in the BIBO host.18
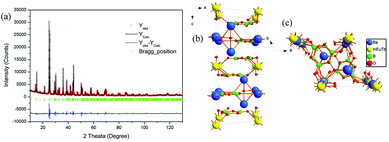 |
| Fig. 2 (a) Rietveld refinement patterns, (b) and (c) crystal structures of the BIBO:0.08Tb3+, 0.25Eu3+ sample. | |
Table 1 Experimental parameters of the powder XRD analysis and refined crystallographic data of the BIBO:0.08Tb3+, 0.25Eu3+ samplea
Rp = ∑|yio − yic|/∑|yio|, Rwp = [∑wi(yio − yic)2/∑wiyio2]1/2, Rexp = [(N − P)/∑wiyio2]1/2. |
Formula |
Ba3In0.67Tb0.08Eu0.25B9O18 |
Diffractometer |
Rigaku D/Max-2500 |
Radiation type |
Cu Kα |
Monochromator |
Graphite |
Wavelength (Å) |
1.5405 |
Refined profile range 2θ (°) |
10–130 |
Step size 2θ (°) |
0.017 |
Step scan time per step (s) |
2 |
Number of structure parameters |
26 |
Number of profile parameters |
17 |
RB |
7.38% |
RP |
5.79% |
RWP |
7.76% |
S |
2.60 |
Symmetry |
Hexagonal |
Space group |
P63/m |
a (Å) |
7.1600(3) |
c (Å) |
16.8123(4) |
Volume (Å3) |
746.43(3) |
Z |
2 |
Calculated density (g cm−3) |
4.11 |
Table 2 Atomic coordinates and isotropic displacement parameters for the BIBO:0.08Tb3+, 0.25Eu3+ sample
Atom |
Site |
x |
y |
z |
Biso (Å2) |
Occ |
Ba1 |
4f |
1/3 |
2/3 |
0.13063(5) |
0.247(4) |
0.333(0) |
In1 |
2b |
0 |
0 |
0 |
0.376(8) |
0.117(0) |
Eu1 |
2b |
0 |
0 |
0 |
0.376(8) |
0.042(0) |
Tb1 |
2b |
0 |
0 |
0 |
0.376(8) |
0.008(0) |
Ba2 |
2a |
0 |
0 |
1/4 |
2.41(1) |
0.167(0) |
O1 |
6h |
0.454(1) |
0.295(1) |
1/4 |
−1.52(3) |
1/2 |
O2 |
12i |
−0.1160(9) |
0.3856(9) |
0.0794(3) |
0.44(3) |
1 |
O3 |
6h |
0.596(1) |
0.672(1) |
1/4 |
0.81(4) |
1/2 |
O4 |
12i |
−0.0056(7) |
0.7487(7) |
0.0819(3) |
0.55(3) |
1 |
B1 |
6h |
0.604(3) |
0.483(3) |
1/4 |
1.39(9) |
1/2 |
B2 |
12i |
−0.172(2) |
0.554(2) |
0.0783(6) |
−0.10(4) |
1 |
3.2 Photoluminescence properties
The BIBO, as a potential host for phosphors, exhibits a broad and intense emission band in the range of 360–500 nm with weak violet-blue emission on account of the recombination of an electron on a donor formed by oxygen vacancies with a hole on an acceptor consisting of indium or barium vacancies.8,9,19 As presented in Fig. 3, the photoluminescence of single Tb3+- or Eu3+-doped BIBO demonstrated excellent performance for emitting intense green and red lighting when studied under UV excitation. Fig. 3a shows the excitation and emission spectra of the BIBO:0.08Tb3+ sample. Intense green emission at 550 nm based on the 5D4–7FJ (J = 6, 5, 4, 3) transitions of Tb3+ ions in BIBO host can be observed under 232 nm excitation. Under 232 nm excitation, the main excitation band is located at 232 nm due to the spin-allowed 4f8–4f75d1 transitions of Tb3+ ions.20,21 Moreover, as seen in Fig. 3b, upon 221 nm excitation, the BIBO:Eu3+ phosphor exhibits a red emission, attributed to the absorption band magnetic dipole transition 5D0–7F1.22–25 The excitation spectrum was obtained by monitoring the emission of Eu3+ at 590 nm, and showed an absorption band ranging from 200 to 250 nm containing the excitation spectrum of Eu3+. Furthermore, the broad excitation band centered at 211 nm should be assigned to the charge transfer band of O2− → Eu3+. Fig. 3c illustrates the excitation and emission spectrum of the typical BIBO:0.08Tb3+, 0.25Eu3+ phosphor. Once Eu3+ is doped into BIBO:0.08Tb3+, both the Tb3+ green emission peak at 550 nm and the Eu3+ red emission at 590 nm were found in the PL spectrum of Tb3+, Eu3+ codoped BIBO phosphor at 233 nm excitation. Monitoring the 550 nm emission, the PLE spectrum of BIBO:0.08Tb3+, 0.25Eu3+ had a similar profile with that of BIBO:0.08Tb3+ and showed a peak wavelength at 233 nm, indicating effective energy transfer from Tb3+ ions to Eu3+ ions. Moreover, monitoring 590 nm emission, an obvious split in the excited band below 250 nm was found at 219 and 233 nm in the Eu3+ and Tb3+ ions codoped BIBO, corresponding to the CTB bands of Eu3+ and Tb3+ in Fig. 3a and b, implying the energy transfer from Tb3+ to Eu3+.
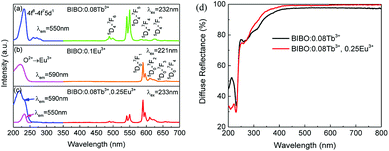 |
| Fig. 3 UV excitation and emission spectra of (a) BIBO:0.08Tb3+, (b) BIBO:0.1Eu3+, and (c) BIBO:0.08Tb3+, 0.25Eu3+, and (d) diffuse reflectance spectra of the BIBO:0.08Tb3+ and BIBO:0.08Tb3+, 0.25Eu3+ samples. | |
In addition, the diffuse reflectance spectra of BIBO:0.08Tb3+ and BIBO:0.08Tb3+, 0.25Eu3+ phosphors are given in Fig. 3d, and are consistent with the above PLE spectra. For BIBO:0.08Tb3+, there were two absorption bands located in the range of 210–250 nm and 250–400 nm, respectively. The first one is attributed to both the transitions from Tb3+ and from the valence to conduction bands of the host lattice, meanwhile the second one is the absorption band of Tb3+ ions due to the transitions from the 4f to 5d states. Then for the Tb3+, Eu3+ codoped BIBO sample, the diffuse reflectance between 210–250 nm is obviously reduced, indicating the higher absorption caused by the energy transfer of Eu3+ and Tb3+. By the intercept method, the optical band gap was estimated as 5.34 and 5.41 eV for BIBO:0.08Tb3+ and BIBO:0.08Tb3+, 0.25Eu3+, respectively. This means that the band gap of BIBO is slightly widened by the codoped Tb3+ and Eu3+ ions.
The concentration-dependent emission spectra of BIBO:xTb3+, 0.1Eu3+ with varying Tb3+ concentrations under 233 nm excitation are given in Fig. 4a and b. With increasing the Tb3+ concentration, the emission intensity of Eu3+ at 590 nm is linearly increased, whereas the red emission intensity of Tb3+ at 550 nm reaches the maximum at x = 0.12, and then generally decreases due to the concentration quenching of Eu3+ ions. The shapes of all PL spectra remain unchanged with varying the Tb3+ concentration. In addition, considering the results of single Tb3+-doped BIBO phosphors, the concentration Tb3+ is fixed at 0.08 mol in the codoped samples.11 Fig. 4c and d illustrate the emission spectra and luminescence intensity of Tb3+ and Eu3+ for BIBO:0.08Tb3+, yEu3+. With increasing the concentration of Eu3+, the luminescence of Tb3+ decreases gradually, whereas that of Eu3+ first reaches a maximum at y = 0.25 and then begins to decline. This further implies the energy transfer from Tb3+ to Eu3+ in the BIBO host. Usually, the excitation of the isomorphic compounds of Ba3REB9O18 is primarily determined by the B3O6 borate groups.26–28 When ions with a smaller radius are replaced by larger ones with little electronegativity, they will attract the electrons from O2− more weakly based on the bond structure of RE3+–O2−–B3+. On this account, the electron-cloud density of the O2− ion increased and it needed less energy for the electron transfer from the O2− 2p6 valence bands to B3+ 2s and 2p conduction.28 Therefore, the optimal excitation wavelength slightly increases from 232 to 233 nm, ascribed to the increasing concentration of Eu3+ with a larger ionic radius.
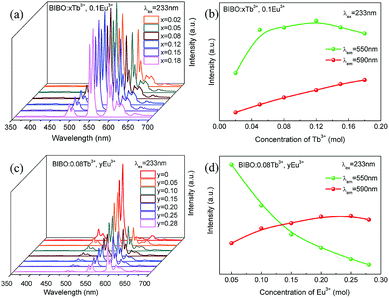 |
| Fig. 4 (a) UV emission spectra and (b) luminescence intensity of Tb3+ and Eu3+ for BIBO:xTb3+, 0.1Eu3+, (c) UV emission spectra and (d) luminescence intensity of Tb3+ and Eu3+ for BIBO:0.08Tb3+, yEu3+. | |
With the development of plasma display panels (PDPs) and large flat panel displays (FPDs), an economic phosphor that can be excited efficiently in the vacuum ultraviolet (VUV) range was needed to convert the VUV photons, particularly the Xe resonance emission to red, green, and blue (RGB) tricolor lights.29,30 The synchrotron radiation light source makes investigations in the VUV region possible. Then the energy level of rare-earths in the VUV region can be acquired and assumed. The emission spectra and luminescence intensity of BIBO:0.08Tb3+, yEu3+ under VUV excitation are presented in Fig. 5a and b. The spectra are similar to those obtained under UV conditions. Note that the red emission band of Eu3+ is slightly moved from 590 to 589 nm, probably due to the different excitation source compared with the Xe lamp. The intensity of emission spectra for Tb3+ and Eu3+ under the VUV region was modified as a result of Tb3+ and Eu3+ codoping into BIBO, similar to the case for the UV measurements in Fig. 4.
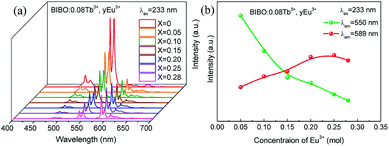 |
| Fig. 5 (a) VUV emission spectra, and (b) luminescence intensity of Tb3+ and Eu3+ for BIBO:0.08Tb3+, yEu3+. | |
3.3 Energy-transfer mechanism
In order to further investigate the energy transfer from Tb3+ to Eu3+ in BIBO:Tb3+, Eu3+ phosphors, the decay curves of the Tb3+ emission were measured and are illustrated in Fig. 6. Generally, a double-exponential decay behavior of an activator is frequently observed when the excitation energy is transferred from the sensitizer to activator. Hence, all decay curves of BIBO:0.08Tb3+, yEu3+ could be well fitted using a biexponential function:31–33 |
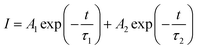 | (1) |
where I is the luminescence intensity at time t, τ1 and τ2 refer to two components of the luminescence lifetime, A1 and A2 are constants, and t stands for the average decay time. According to these parameters, the average decay times (τ) can be determined by the following formula:31–33 |
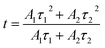 | (2) |
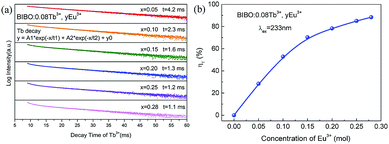 |
| Fig. 6 (a) Decay curves for the luminescence of Tb3+ monitored at 550 nm, and (b) the energy-transfer efficiency for BIBO:0.08Tb3+, yEu3+ phosphors. | |
The decay lifetimes of Tb3+ and Eu3+ in a series of BIBO:0.08Tb3+, yEu3+ samples were obtained under the same conditions. As shown in Fig. 6a, the effective lifetime values of Tb3+ monitored at 550 nm were calculated as 4.2, 2.3, 1.6, 1.3, 1.2, and 1.1 ms for x = 0.05, 0.10, 0.15, 0.20, 0.25, and 0.28, respectively. In the case of energy transfer, the luminescent lifetime of a sensitizer will be shortened, because of the presence of additional decay channels that shorten the lifetime of the excited state. In this work, the decay lifetimes of Tb3+ descended with increasing the Eu3+ concentration, which demonstrated the occurrence of efficient energy transfer from Tb3+ to the neighboring Eu3+ in the BIBO host.
The energy-transfer efficiency ηT from Tb3+ to Eu3+ ions in BIBO:0.08Tb3+, yEu3+ can be expressed by the formula:34
|
 | (3) |
where
IS0 and
IS are the luminescence intensity of Tb
3+ in the absence and presence of Eu
3+, respectively. As given in
Fig. 6b,
ηT was found to increase gradually with increasing the Eu
3+ concentration, reaching a maximum of 88.4% when the concentration of Eu
3+ was up to 0.28.
The resonant energy transfer mechanism consists of two types: exchange interaction and multipolar interaction. The critical distance between the sensitizer and activator should be shorter than 3–4 Å when the energy transfer occurs via the exchange interaction.35,36 Furthermore, the critical distance of the Tb3+ → Eu3+ energy transfer Rc can be calculated via using the concentration quenching method, and the critical distance between Tb3+ and Eu3+ could be estimated by the following formula:35,36
|
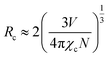 | (4) |
where
V stands for the volume of the unit cell,
N is the number of host cations in the unit cell, and
χc is the quenching concentration of the summation of the sensitizer of Tb
3+ and activator of Eu
3+. For the BIBO host, based on
V = 746.43 Å
3,
N = 6, and
χc = 0.18, the critical distance of energy transfer for Tb
3+ and Eu
3+ in BIBO materials was calculated to be 10.97 Å, indicating that the electric multipolar interaction dominates the energy transfer from Tb
3+ to Eu
3+ ions in BIBO.
According to the above analysis, a schematic energy-level diagram and the energy transfer between Tb3+ and Eu3+ in BIBO is presented in Fig. 7. It is known that the exchange and electrostatic interaction are generated from physical interaction between the sensitizer and activator ions during the energy-transfer process. After the multistep relaxations, the excited electrons of Tb3+ are excited from the ground state 7F6 to the excitation state 5D4, and then jump to the 7F5 state accompanied by energy release under 233 nm excitation. Simultaneously, the rest of the Tb3+ ions with the 5D4 excited state transfer their energy to the 5D1 excited state of Eu3+ ions, leading to red emission from Eu3+ ions through 5D0–7FJ (J = 0–6) transitions.37–39
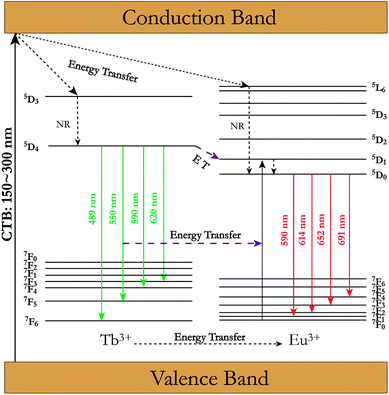 |
| Fig. 7 Schematic energy level diagram and energy-transfer process from Tb3+ to Eu3+ in BIBO. (NR stands for nonradiative transition). | |
3.4 Low-temperature dependence under VUV
The luminescence properties of a typical BIBO:0.08Tb3+, 0.1Eu3+ phosphor under VUV excitation were investigated in the temperature range of 25 K and 298 K, and exhibited temperature dependence properties. As seen in Fig. 8, besides the 209 and 234 nm excitation bands, which were observed to the same to the above UV spectra, another band centered at 164 nm was demonstrated, attributed to defects in the host. Under 164 nm excitation, the wide emission band ranged from 340 to 400 nm, assigned to the intrinsic emission of the BIBO host (Fig. 8a). As shown in Fig. 8b and c, the emission spectra were also excited with 209 nm and 234 nm, which showed intensified emission at 589 and 549 nm due to the two luminescence centers of Eu3+ and Tb3+ and the energy transfer between them. The broad absorption bands of the BIBO:0.08Tb3+, 0.1Eu3+ phosphor indicated good potential for applications in the display or lighting field. Furthermore, note that the luminescence intensity of Tb3+ and Eu3+ in the codoped BIBO phosphor was sensitive to temperature and exhibited a feature of temperature dependence (Fig. 8d). The highest emission was observed at the lowest temperature (25 K). In addition, at room temperature, the emission maximum intensity of Tb3+ centered at 549 nm and Eu3+ centered at 589 nm, respectively, declined by 34.5% and 24.6% for BIBO:0.08Tb3+, 0.1Eu3+ as compared to the initial intensity measured at 25 K. Usually, the host lattice extension is caused by thermal expansion with the increased temperature. Then the increased bond length between the activator and the ligand leads to a decrease in the crystal field, thus reducing the transition energy.40–42 Furthermore, the emission intensity can be fitted by using the Arrhenius equation,43,44 |
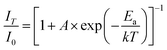 | (5) |
where I0 is the initial intensity, IT is the intensity at a given temperature T, A is a constant, k is the Boltzman constant, and Ea is the energy barrier for thermal quenching. Then the values of activation energy for thermal quenching (Ea) were calculated as 0.381 eV for Tb3+ and 0.469 eV for Eu3+, respectively. The relatively high activation energy indicated that the emission of Eu3+ had a higher thermal barrier for luminescence quenching.
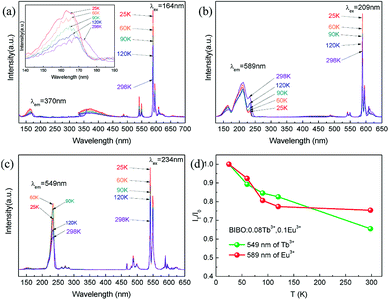 |
| Fig. 8 The VUV excitation and emission spectra under (a) 164 nm, (b) 209 nm, (c) 234 nm excitation with different temperatures, and (d) low-temperature-dependent emission intensity of BIBO:0.08Tb3+, 0.1Eu3+. | |
3.5 Tunable emissions
Under the excitation of 233 nm, the CIE chromaticity coordinates of BIBO:0.08Tb3+, yEu3+ were calculated and the results are listed in Table 3. With the increasing concentration of codoped Eu3+ in BIBO:0.08Tb3+, the emission color varied from yellowish green to orange, with the corresponding CIE chromaticity coordinates from A0 (y = 0) to A6 (y = 0.28) illustrated in Fig. 9. The results indicate that the as-obtained phosphors showed merits of multicolor emissions in the visible region when excited by a single wavelength light. The inset figures are the digital photographs of BIBO:0.08Tb3+, yEu3+ upon 254 nm UV-lamp excitation. As also given in the photographs of the emitting phosphors, the variation of the as-observed emitting color is obvious, which means that tunable luminescence could be realized in the novel Tb3+ and Eu3+ codoped BIBO phosphors based on effective energy transfer.
Table 3 CIE Chromaticity Coordinate for BIBO:0.08Tb3+, yEu3+ phosphors under 233 nm excitation
No. of points |
BIBO:0.08Tb3+, yEu3+ |
CIE (x, y) |
A0 |
y = 0 |
0.3119, 0.6127 |
A1 |
y = 0.05 |
0.3748, 0.5595 |
A2 |
y = 0.10 |
0.4203, 0.5189 |
A3 |
y = 0.15 |
0.4583, 0.4838 |
A4 |
y = 0.20 |
0.4869, 0.4586 |
A5 |
y = 0.25 |
0.5102, 0.4380 |
A6 |
y = 0.28 |
0.5194, 0.4277 |
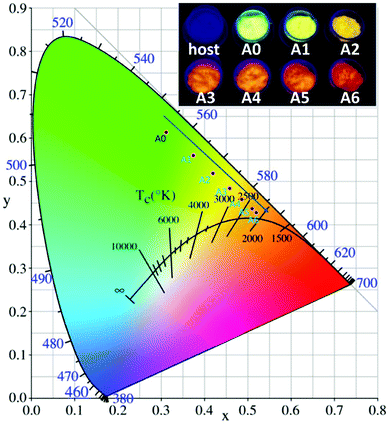 |
| Fig. 9 CIE chromaticity diagram excited at 233 nm and the emitting color under 254 nm UV lamp excitation of BIBO:0.08Tb3+, yEu3+ phosphors. | |
4. Conclusions
In conclusion, a series of Tb3+ and Eu3+ codoped BIBO phosphors were synthesized by high-temperature solid-state reaction. The crystal structure and the sites of Tb3+ and Eu3+ ions of BIBO:Tb3+, Eu3+ phosphors were investigated using the Rietveld refinement method. Then the luminescence properties of BIBO:Tb3+, Eu3+ phosphors were investigated under ultraviolet (UV) and vacuum ultraviolet (VUV) excitation. Energy transfer between Tb3+ and Eu3+ ions was demonstrated by the PL spectra and decay time. The energy-transfer mechanism from Tb3+ to Eu3+ in Ba3InB9O18 was dominated by electric multipolar interactions, with the critical distance calculated to be 10.97 Å. Moreover, the temperature sensitivity of the sample under VUV was investigated at low temperature ranging from 25 K to 298 K. The emission colors of BIBO:Tb3+, Eu3+ could be adjusted from yellowish green to orange by tuning the content of Eu3+ ions under UV radiation, thus showing a great potential for their use in display and lighting fields applications.
Conflicts of interest
There are no conflicts to declare.
Acknowledgements
This work was supported by the National Natural Science Foundation of China (Grant numbers 51472273 and 51772330), and Beijing Synchrotron Radiation Facilities (BSRF). The Aid program for the Natural Science Foundation of Hunan Province (2017JJ2403), the Key Research Foundation of Education Bureau of Hunan Province (No. 16A220), and Science and Technology Innovative Research Team in Higher Educational Institutions of Hunan Province (No. 2014207) are gratefully acknowledged.
Notes and references
- J. Zhang, G. M. Cai, L. W. Yang, Z. Y. Ma and Z. P. Jin, Inorg. Chem., 2017, 56, 12902 CrossRef CAS PubMed.
- S. P. Tiwari, A. Kumar, S. Singh and K. Kumar, Vacuum, 2017, 146, 537 CrossRef CAS.
- V. V. Shinde, S. V. Shinde, N. S. Dhoble and S. J. Dhoble, J. Inorg. Organomet. Polym., 2015, 25, 593 CrossRef CAS.
- C. C. Lin, W. T. Chen, C. I. Chu, K. W. Huang, C. W. Yeh, B. M. Cheng and R. S. Liu, Light: Sci. Appl., 2016, 5, e16066 CrossRef CAS PubMed.
- C. He, Z. Xia and Q. Liu, Opt. Mater., 2015, 42, 11 CrossRef CAS.
- R. Velchuri, B. Vijaya Kumar, V. Rama Devi, G. Prasad, D. Jaya Prakash and M. Vithal, Mater. Res. Bull., 2011, 46, 1219 CrossRef CAS.
- D. Chikte, S. K. Omanwar and S. V. Moharil, J. Lumin., 2013, 142, 180 CrossRef CAS.
- G. Cai, X. L. Chen, W. Y. Wang, Y. F. Lou, J. Liu, J. T. Zhao and H. H. Chen, J. Solid State Chem., 2008, 181, 646 CrossRef CAS.
- J. S. Zhang, Z. D. Hao, X. Zhang, Y. S. Luo, X. G. Ren, X. J. Wang and J. H. Zhang, J. Appl. Phys., 2009, 106, 34915 CrossRef.
- Z. J. Liang, F. W. Mo, X. G. Zhang, L. Y. Zhou, P. C. Chen and C. Y. Xu, Ceram. Int., 2014, 40, 7501 CrossRef CAS.
- G. M. Cai, F. Zheng, D. Q. Yi, Z. P. Jin and X. L. Chen, J. Lumin., 2010, 130, 910 CrossRef CAS.
- L. H. Tian, B. Y. Yu, C. H. Pyun, H. L. Park and S. I. Mho, Solid State Commun., 2004, 129, 43 CrossRef CAS.
- R. S. Kumar, V. Ponnusamy and M. S. Jose, Eur. Phys. J.: Appl. Phys., 2014, 68, 30702 CrossRef.
- J. Cao, X. Li, Z. Wang, Y. Wei, L. Chen and H. Guo, Sens. Actuators, B, 2016, 224, 507 CrossRef CAS.
- J. Cao, X. Wang, X. Li, Y. Wei, L. Chen and H. Guo, J. Lumin., 2016, 170, 207 CrossRef CAS.
- G. J. Wang, D. J. Pan, T. Xu, G. X. Xiang, Z. J. Zhang, H. T. Hintzen, J. T. Zhao and Y. Huang, J. Alloys Compd., 2017, 708, 154 CrossRef CAS.
- J. Rodriguez-Carvajal, Phys. Rev. B: Condens. Matter Mater. Phys., 1993, 192, 55 CrossRef CAS.
- R. D. Shannon, Acta Crystallogr., Sect. A: Cryst. Phys., Diffr., Theor. Gen. Crystallogr., 1976, 32, 751 CrossRef.
- Z. X. Wang, H. Pei, X. M. Tao, G. M. Cai, R. H. Mao and Z. P. Jin, J. Solid State Chem., 2018, 258, 351 CrossRef CAS.
- M. Zawadzki, D. Hreniak, J. Wrzyszcz, W. Mista, H. Grabowska, O. L. Malta and W. Strek, Chem. Phys., 2003, 291, 275 CrossRef CAS.
- Z. Zhang, S. Zhang, W. Zhang and W. Yang, Solid State Sci., 2017, 64, 69 CrossRef CAS.
- G. Blasse and B. C. Grabmaier, Luminescent Materials, Springer-Verlag, Berlin, 1994 Search PubMed.
- S. Shionoya and W. M. Yen, Phosphor Handbook, CRC Press, Boca Raton, 1999 Search PubMed.
- S. K. Sharma and M. M. Malik, J. Lumin., 2016, 173, 231 CrossRef CAS.
- S. Jayakiruba, G. Kumar and N. Lakshminarasimhan, Solid State Sci., 2016, 55, 121 CrossRef CAS.
- G. M. Cai, M. Li, J. Liu, S. F. Jin, W. Y. Wang, F. Zheng and X. L. Chen, Mater. Res. Bull., 2009, 44, 2211 CrossRef CAS.
- G. M. Cai, M. He, X. L. Chen, W. Y. Wang, Y. F. Lou, H. H. Chen and J. T. Zhao, Powder Diffr., 2007, 22, 328 CrossRef CAS.
- C. Duan, J. Yuan, X. Yang, J. Zhao, Y. Fu, G. Zhang, Z. Qi and Z. Shi, J. Phys. D: Appl. Phys., 2005, 38, 3576 CrossRef CAS.
- B. Han, H. B. Liang, H. Y. Ni, Q. Su, G. T. Yang, J. Y. Shi and G. B. Zhang, Opt. Express, 2009, 17, 7138 CrossRef CAS PubMed.
- M. B. Xie, Y. Tao, Y. Huang, H. B. Liang and Q. Su, Inorg. Chem., 2010, 49, 11317 CrossRef CAS PubMed.
- G. M. Cai, J. J. Fan, H. K. Li, Z. Zhao, L. M. Su and Z. P. Jin, J. Alloys Compd., 2013, 562, 182 CrossRef CAS.
- M. Shang, D. Geng, X. Kang, D. Yang, Y. Zhang and J. Lin, Inorg. Chem., 2012, 51, 11106 CrossRef CAS PubMed.
- T. Jiang, X. Yu, X. Xu, H. Yu, D. Zhou and J. Qiu, Mater. Res. Bull., 2014, 51, 80 CrossRef CAS.
- P. I. Paulose, G. Jose, V. Thomas, N. V. Unnikrishnan and M. K. R. Warrier, J. Phys. Chem. Solids, 2003, 64, 841 CrossRef CAS.
- G. Blasse, Philips Res. Rep., 1969, 24, 131 CAS.
- J. T. Zhang, C. Y. Ma, Z. C. Wen, M. M. Du, J. Q. Long, R. Ma, X. Y. Yuan, J. T. Li and Y. G. Cao, Opt. Mater., 2016, 58, 290 CrossRef CAS.
- Y. T. Lin, Z. R. Niu, Y. Han, C. Z. Li, W. L. Zhou, J. L. Zhang, L. P. Yu and S. X. Lian, J. Alloys Compd., 2017, 690, 267 CrossRef CAS.
- S. Loos, F. Steudel, B. Ahrens and S. Schweizer, J. Lumin., 2017, 181, 31 CrossRef CAS.
- J. Y. Si, N. Liu, S. Y. Song, G. M. Cai, N. Yang and Z. Li, J. Alloys Compd., 2017, 719, 171 CrossRef CAS.
- L. M. Su, X. Fan, G. M. Cai and Z. P. Jin, RSC Adv., 2017, 7, 22156 RSC.
- J. S. Kim, Y. H. Park, S. M. Kim, J. C. Choi and H. L. Park, Solid State Commun., 2005, 133, 445 CrossRef CAS.
- Z. Xia, X. Wang, Y. Wang, L. Liao and X. Jing, Inorg. Chem., 2011, 50, 10134 CrossRef CAS PubMed.
- S. Bhushan and M. V. Chukichev, J. Mater. Sci. Lett., 1988, 7, 319 CrossRef CAS.
- P. Dorenbos, J. Phys.: Condens. Matter, 2005, 17, 8103 CrossRef CAS.
|
This journal is © The Royal Society of Chemistry 2019 |
Click here to see how this site uses Cookies. View our privacy policy here.