DOI:
10.1039/C8RA08984A
(Paper)
RSC Adv., 2019,
9, 4539-4544
Multifunctional property exploration: Bi4O5I2 with high visible light photocatalytic performance and a large nonlinear optical effect†
Received
30th October 2018
, Accepted 20th January 2019
First published on 5th February 2019
Abstract
Exploration of the versatility of materials is very important for increasing the utilization of materials. Herein, we successfully prepared Bi4O5I2 powders via a facile solvothermal method. The Bi4O5I2 photocatalyst exhibited significantly higher photocatalytic activity as compared to the common BiOI photocatalyst in the degradation of methyl orange, methylene blue and rhodamine B under visible light irradiation. Especially, for the degradation of methyl orange, the photocatalytic activity of Bi4O5I2 is about 10 times that of BiOI. Moreover, Bi4O5I2 exhibits an extremely high second harmonic generation response of about 20 × KDP (the benchmark) estimated by the unbiased ab initio calculations. The coexisting multifunction of Bi4O5I2 is mainly because of the increased dipole moment due to the stereochemical activity of lone pairs that promotes separation and transfer of photogenerated carriers, then enhances the photocatalytic activity and results in a high second harmonic generation response. This indicates that Bi4O5I2 may have good potential applications in photocatalytic and nonlinear optical fields.
1. Introduction
Green, efficient, harmonious and civilized society is the development trend of future life. Ever since Fujishima and Honda have reported the photocatalytic water splitting on the TiO2 surface,1 TiO2 has been extensively studied for the degradation of pollutants or splitting of water.2 However, the wide bandgap (3.0–3.2 eV) of TiO2 limits its application range. Therefore, it is essential to find visible light-driven photocatalysts. Methylene blue (MB), methyl orange (MO) and rhodamine B (RhB) are the main components in common water pollutants. Scientists have invented many methods for the degradation of organic pollutants.3–8 Bismuth-containing materials are attracting significant attention of researchers due to their unique electronic, magnetic, optical, and catalytic properties;9–13 however, the existence of high photogenerated carrier recombination rate restricts the development of Bi-based photocatalysts.
To enhance the photocatalytic activity, scientists have carried out a lot of research and exploration.14–21 To the best of our knowledge, in 2002, Bi4O5I2 was successfully synthesized by changing the stoichiometry of bismuth oxyhalide.22 After this, non-stoichiometric ratio halogenated bismuth compounds, for example, Bi4O5I2, Bi4O5Br2, and Bi5O7Br, have been studied constantly.23,24 Ye et al. have committed to the research and development of non-stoichiometric Bi-based photocatalysts. They made a series of innovative achievements such as photocatalytic water splitting for hydrogen (H2) evolution,25 selective photoreduction of CO2 into CO,26 molecular oxygen activation and RhB degradation.27,28 Xiao et al.29,30 have successfully synthesized Bi4O5I2 nanoflakes, which had better photocatalytic activities in the degradation of 4-tert-butylphenol, and predicted that they could be applied to other fields as well.
Non-stoichiometric ratio halogenated bismuth compounds have become the most promising second-order nonlinear optical (NLO) and photovoltaic (PV) candidate materials owing to their special structural features, rich chemical compositions, and suitable optical band gaps. In fact, most of these noncentrosymmetric structures could be applied in more than one of the aforementioned fields. It is known that nonlinear optical (NLO) materials have played an important role in laser science and technology field and are rapidly developing.31–34 The NLO crystals are laser frequency conversion materials, and their SHG coefficients are the focus of attention, which may reflect the ability of the NLO crystal to generate harmonic laser emission.35,36 Among them, fluoroborate is responsive to ultraviolet light and is widely used in military, medical, aviation and other fields.37 It is known that lower SHG coefficient limits their applications. Therefore, the search for new NLO materials with SHG response larger than 10 × KDP has attracted significant attention.
In general, noncentrosymmetric crystal structure materials may possess very good NLO properties.28 In 2014, Fan et al.38–40 found a series of materials, such as K3B6O10Br, Na3VO2B6O11, and M2B5O9Cl (M = Ca, Sr, Ba, Pb), with noncentrosymmetric structures. Due to intrinsic large polarization effects, they not only have good nonlinear optical properties, but have also been found to exhibit significantly enhanced photocatalytic activities.
To the best of our knowledge, the noncentrosymmetric polarized Bi4O5I2 belongs to the space group P21.22 Then, does it have the multifunctional property? To research the potential properties of Bi4O5I2, we carried out photodegradation experiments and theoretical calculations of the optical properties. The results showed that Bi4O5I2 had high visible light photocatalytic performance. Moreover, the theoretical calculation showed that Bi4O5I2 exhibited large second harmonic generation (SHG) response. At present, the versatility of materials is a hot research topic that scientists have been exploring. Based on the abovementioned findings, herein, we successfully prepared BiOI and Bi4O5I2 materials, and some characterizations and calculations were performed to verify the versatility of these materials.
2. Experimental
2.1. Materials and methods
Ethylene glycol (EG), bismuth nitrate pentahydrate (Bi(NO3)3·5H2O), and potassium iodide (KI) were purchased commercially and used without further purification. Moreover, deionized water was used throughout this study.
Bi4O5I2 powders were synthesized via a facile solvothermal method. A mixture of 2 mmol Bi(NO3)3·5H2O, 4 mmol KI and 35 mL EG was prepared and transferred to a 45 mL Teflon-lined stainless steel autoclave, and the pH of the suspension was adjusted to 10 using 2 mol L−1 NaOH. Subsequently, it was maintained at 150 °C for 12 h followed by naturally cooling to room temperature. The precipitate was centrifugally washed with distilled water and absolute ethanol for several times and then dried at 80 °C for 5 h under an air atmosphere. BiOI was obtained through the abovementioned procedure without the addition of NaOH.
2.2. Characterization
Phase identification was performed using the Bruker D8 ADVANCE X-ray diffractometer (XRD) equipped with a diffracted-beam monochromator set for Cu and Kα (λ = 0.154059 nm) as the radiation sources. The acceleration voltage and acceleration current are 40 kV and 40 mA, respectively. The results were obtained by scanning the sample through a range of 2 theta values (10–85°). UV-visible diffuse reflectance spectra (DRS) were obtained via the Shimadzu UV-3600 UV-vis spectrophotometer using BaSO4 as a reference. The morphologies of the samples were observed via a scanning electron microscope (SEM) using a Zeiss SUPRA 55VP apparatus, and energy dispersive X-ray (EDX) spectroscopic analysis was conducted using EDX-8000. The photocurrents (PC) of UV-vis light on and off studies were measured via the CHI660E electrochemical system (Shanghai, China) using a standard three-electrode cell containing a working electrode (1.0 cm × 1.0 cm), a platinum plate as the counter electrode, and a standard calomel electrode (SCE) as the reference electrode. Electrochemical impedance spectroscopy (EIS) measurements were carried out using an electrochemical workstation (Zahner Im6ex) with the working electrode in the frequency range from 100 kHz to 0.01 Hz at open circuit potential in a 0.1 M KCl solution.
2.3. Photocatalytic reaction
The photocatalytic activities of BiOI and Bi4O5I2 samples were tested using a 300 W Xe lamp as the light source and by the degradation of MO, MB, RhB and norfloxacin (NOR) under the visible-light irradiation. Optical filters were used to eliminate the UV light (λ ≤ 420 nm). The power density at the position of the reactor is about 7.4 mW cm−2. In each of the experiment, 50 mg catalyst powder was dissolved in an aqueous solution of MO (100 mL, 10 mg L−1) in a beaker. Prior to irradiation, the suspensions were magnetically stirred in the dark for 30 min to achieve an adsorption–desorption equilibrium. Reduction in the concentrations of dyes was analyzed by the Cary 500 UV-vis spectrophotometer at the wavelength of maximal absorption (464 nm, 664 nm, and 554 nm). The samples of the reaction solution were taken out and then centrifuged and filtered at regular intervals. Finally, the filtrates obtained were analyzed.
2.4. Numerical calculation details and methods
The electronic structures and NLO properties of BiOI and Bi4O5I2 were simulated by the CASTEP package.41 The experimental crystal data were used in density functional theory (DFT) calculation via a plane wave pseudo-potential method. During the calculation, geometry optimization was performed using the BFGS minimization technique. The local density approximation (LDA) with the Ceperley–Alder–Perdew–Zunger functional and the ultrasoft pseudopotentials (USPs) were chosen as the exchange correlation functional and pseudopotential, respectively.42 In the electronic structure and optical property calculations, the ultra-fine pseudopotentials were set up for BiOI and Bi4O5I2. Bi 5d106s26p3, I 5s25p5, and O 2s22p4 were chosen as the valence electrons. The k-point separation of each material was set to 0.04 Å−1 in the Brillouin zone.
3. Results and discussion
3.1. XRD analysis
BiOI and Bi4O5I2 belong to the tetragonal space group P4/nmm with cell dimensions a = 3.995(2) Å, b = 3.995(2) Å, and c = 9.151(5) Å and noncentrosymmetric monoclinic space group P21 with cell dimensions a = 14.944(1) Å, b = 5.6983(3) Å, and c = 11.263(1) Å, respectively. Powder XRD was used to characterize the crystal structure of the products. Fig. 1 shows the XRD patterns of the BiOI and Bi4O5I2 samples. As shown in Fig. 1a, all the observed peaks of the pattern can be indexed to the tetragonal phase of BiOI (ICSD 391354), and the observed major diffraction peaks at 29.65°, 31.66°, 45.38°, 51.35° and 55.15° belong to (102), (110), (200), (114) and (212) planes of the tetragonal phase BiOI, respectively. Fig. 1b clearly shows that the diffraction data match well with the phase of Bi4O5I2 (ICSD 412590). Moreover, it can be clearly seen that the characteristic peaks located at 28.8, 31.5, 45.1, 49.3, and 54.4 can be indexed to (−4−11), (402), (422), (006), and (811) planes of Bi4O5I2, respectively (Fig. 1b). These findings are consistent with the previously reported data. Therefore, it can be initially determined that BiOI and Bi4O5I2 samples have been successfully synthesized.
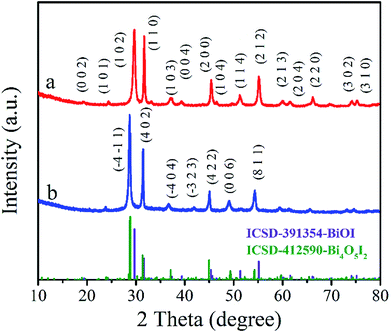 |
| Fig. 1 XRD patterns of the BiOI (a) and Bi4O5I2 (b) samples. | |
3.2. SEM and EDS analyses
To identify the morphological information and atomic compositions of samples, BiOI and Bi4O5I2 samples were investigated by SEM and EDS. The results are shown in Fig. 2. As clearly observed from Fig. 2a–d, the architecture and morphology of Bi4O5I2 nanosheets are distinctly different from those of BiOI. The flaked structure of the Bi4O5I2 nanosheet has been successfully prepared. The EDS results are shown in Fig. S1a and b,† and the Bi
:
I ratios of Bi4O5I2 and BiOI are determined to be 2.2
:
1 and 1.08
:
1, respectively. The EDS results further demonstrate the conclusion drawn based on the XRD results.
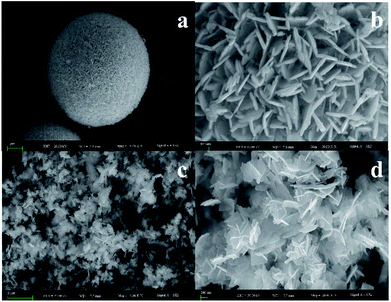 |
| Fig. 2 SEM images of different magnifications of BiOI (a and b) and Bi4O5I2 (c and d) samples. | |
3.3. UV-vis diffuse reflectance spectral analysis
As shown in Fig. 3a, the absorption edges of BiOI and Bi4O5I2 samples are about 663 nm and 536 nm, respectively. The band gap energies of BiOI and Bi4O5I2 may be determined by the following equation:43–47
where α, h, hν, A, and Eg are the absorption coefficient, Planck's constant, photon energy, a constant value, and bandgap energy, respectively. In addition, n is determined by the transition type of a semiconductor (n = 1 for direct transition, whereas n = 4 for indirect transition). According to the previously reported studies,48 the n value of BiOI and Bi4O5I2 is 4, which is consistent with the band structure results. According to the formula, the band gap energies for BiOI and Bi4O5I2 samples are found to be 1.83 and 2.39 eV, respectively.
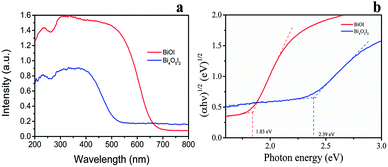 |
| Fig. 3 (a) UV-vis diffuse reflectance spectra of Bi4O5I2 and BiOI. (b) Plots of (αhv)1/2 of Bi4O5I2 and BiOI versus photon energy (hv). | |
3.4. Photocatalytic degradation analysis
As shown in Fig. S2a†, an adsorption–desorption equilibrium has been achieved in dark for 30 min. To better understand the photocatalytic effect of the samples, MO solutions at different initial concentrations were used for comparison. It is observed from Fig. S2b† that lower concentrations of MO degrade more efficiently. As the initial concentration of MO increases, additional organic molecules are able to adsorb onto the photocatalysts surface, thereby reducing the photogeneration of the reactive oxygen species. In addition, it can be clearly seen that Bi4O5I2 displays relatively better degradation efficiency than TiO2 (P25) (Fig. S2a†).
Herein, MO, RhB and MB dyes were selected as model pollutants to evaluate the photocatalytic activities of BOI and Bi4O5I2 (Fig. S2c†). It is clearly seen that Bi4O5I2 displays relatively better degradation efficiency than BiOI. Moreover, as can be seen, under the light irradiation for 100 min, about 29%, 50%, and 90% of RhB, MO and MB were degraded over the BOI samples, respectively. However, under the light irradiation for 60 min, 94% of MO and MB were degraded over the Bi4O5I2 samples. Note that under the light irradiation for 40 min, 99% of RhB was degraded over the Bi4O5I2 samples. In Table ESI-1,† the results show that the photocatalytic activity of the Bi4O5I2 photocatalyst is about 10 times that of BiOI for the degradation of MO. In addition, to minimize the effect of dye sensitization, degradation of colorless solution has been performed (Fig. S2d†). As can be seen, under light irradiation for 100 min, about 43% of NORs were degraded over the Bi4O5I2 samples.
3.5. Free-radical trapping experiment analysis
Trapping experiments may confirm the main active species during the photodegradation process.4,30 Ar, IPA, and KI purging were employed to quench the active species such as ·O2−, ·OH and h+, respectively. At first, trapping agents were dissolved in a Bi4O5I2 solution before the reaction. The free-radical trapping experiment results are shown in Fig. S3a.† It can be seen that the photoinduced h+ and ·O2− radicals are the primary reactive species. To evaluate the repeatability of the Bi4O5I2 sample, recycling experiments for the photocatalytic degradation of MO over the Bi4O5I2 sample were performed (Fig. S3b†). Note that even after 4 cycles, the photocatalytic performance of Bi4O5I2 was retained. These results imply the high stability of the Bi4O5I2 sample, which is significant for its practical application.
3.6. Band structure analysis
The electronic structures of BiOI and Bi4O5I2 were calculated. As shown in Fig. 4a, the highest valence band (VB) is located between the R and Z points and the lowest conduction band (CB) is located between Γ and Z points for BiOI. As shown in Fig. 4b, the highest valence band (VB) is located between D and E points and the lowest conduction band (CB) is located at Γ point for Bi4O5I2. Therefore, the calculated indirect band gaps of BiOI and Bi4O5I2 are about 1.65 and 2.34 eV, which are close to the experimental values of 1.83 and 2.39 eV, respectively.
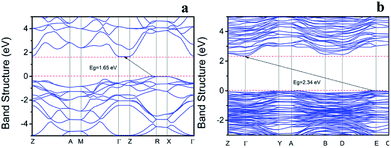 |
| Fig. 4 Band structures of BiOI (a) and Bi4O5I2 (b). | |
3.7. Dipole moment analysis
To identify what promotes the effective separation of photogenerated electron–hole pairs of Bi4O5I2, the dipole moment calculation was carried out.39 The calculations of the Bi–O and Bi–I bond valences and dipole moments of the BiOmIn (m = 4, n = 4; m = 5, n = 3; m = 6, n = 1; m = 3, n = 4; m = 3, n = 5) polyhedra in Bi4O5I2 were performed. The details of the calculations are also provided in ESI.† The results and details of the calculations are provided in Table ESI-2.† It proves that the direction of the dipole moment of Bi4O5I2 powder is along [0 −1 0]. Due to the increased dipole moments, Bi4O5I2 has better separation efficiency of electron–hole pairs. The polarization promotes the separation of photogenerated electron–hole pairs and mitigates the charge recombination, thus resulting in high photocatalytic activity. The calculated results are in accordance with those obtained via the photocatalytic degradation experiments.
3.8. PC and EIS analyses
The separation of photoinduced electron–hole pairs and the charge transfer processes in Bi4O5I2 were investigated by transient photocurrent (TPC) response and electrochemical impedance spectra (EIS), respectively. Fig. 5a shows the instantaneous photocurrent response of BiOI and Bi4O5I2 catalysts. As can be seen from Fig. 5a, due to the action of dipole moments, the photocurrent of Bi4O5I2 is significantly higher than that of BiOI, which promotes the effective separation of photogenerated carriers, thereby resulting in an increase in photocurrent. To better understand the PC measurement results, the EIS spectrum in the form of a Nyquist plot are shown in Fig. 5b. It reflects the recombination rate of electrons and holes during the photoreaction. The smaller arc radius reflects the lower resistance of the interface charge transfer.49 It is clearly shown that Bi4O5I2 has a smaller arc radius, lower chemical impedance and faster charge transport rate. This is consistent with the abovementioned characterization results.
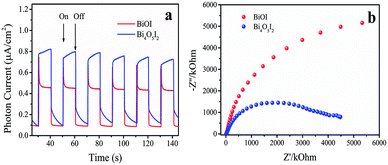 |
| Fig. 5 (a) Transient photocurrent spectra and (b) electrochemical impedance spectra of BiOI and Bi4O5I2. | |
3.9. PL analysis
Photoluminescence (PL) technique was employed herein to further confirm the transfer and recombination of photogenerated electrons and holes for BiOI and Bi4O5I2 during the process of photocatalysis. Fig. S3c† shows that Bi4O5I2 sample exhibits much lower intensity than BiOI sample, which suggests that Bi4O5I2 sample possesses lower recombination rate of the photogenerated charge carriers. It is consistent with the results of PC and EIS analyses.
3.10. Mott–Schottky plot analysis
Mott–Schottky plots (vs. SCE) for Bi4O5I2 are shown in Fig. S3d.† It is known that the conduction bands of the n-type semiconductors are 0–0.1 eV higher than that of the flat potentials, which could be estimated with Mott–Schottky plots. The flat potential is calculated to be −0.75 V versus the saturated calomel electrode (SCE), which is equivalent to −0.51 V versus the normal hydrogen electrode (NHE). Therefore, the ECB of Bi4O5I2 was estimated to be −0.61 eV vs. NHE. EVB vs. NHE can further be calculated from the equation EVB = Eg + ECB, which is estimated to be 1.78 eV vs. NHE.
3.11. Mechanism analysis
Fig. 6 shows a schematic diagram of the band structure and charge migration and separation caused by visible light irradiation. Due to the action of dipole moments, Bi4O5I2 noncentrosymmetric polarized structure promotes the separation of photogenerated electron–hole pairs, which leads to efficient photocatalytic activity. On the other hand, based on the trapping and Mott–Schottky experiment, h+ and ·O2− play the role of major active radicals during photodegradation. The possible reason is that a part of ·O2− is converted into ·OH. In addition, ECB of Bi4O5I2 is estimated to be −0.61 eV vs. NHE, which is lower than that of E0 (O2/O2˙− = −0.046 eV vs. NHE). Therefore, the photogenerated electrons on the CB of Bi4O5I2 could be trapped by molecular oxygen to generate ·O2− under visible light.
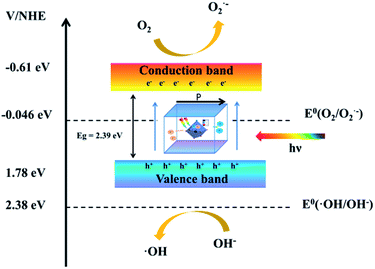 |
| Fig. 6 Schematic of the energy band of Bi4O5I2 as well as charge migration and separation caused by visible light irradiation. | |
However, its valence band is more negative than E0 (OH−/·OH = 2.38 eV vs. NHE). The result is corresponding to the trapping experiments. The mechanism of the active radical production is proposed as follows:
·O2− + H2O2 →·OH + OH− + O2 |
3.12. PDOS and band-resolved analysis
To clearly understand the essence of photocatalysis and NLO properties, the partial density of states (PDOSs) of Bi4O5I2 were calculated. For Bi4O5I2, both the O 2p and I 5p states contributed to the top of the VB and Bi 6p and O 5p states dominated most of the bottom of the CB. The SHG efficiencies are strongly influenced by the direction and the magnitude of the dipole moments of polar structure units.50 The calculated SHG coefficients of Bi4O5I2 are d22 = −7.82 pm V−1 and ∼20 KDP (d22) (where KDP, KH2PO4, d36 ≈ 0.39 pm V−1, which means it should have large SHG responses). Herein, we considered the SHG efficiencies of Bi4O5I2. However, because of the very small size of the Bi4O5I2 powder particles, the SHG efficiencies could not be directly accommodated in the experiment. We could not test the results of theoretical predictions experimentally. Therefore, we planned to grow bigger Bi4O5I2 crystals and further study and explore the NLO properties of Bi4O5I2. In addition, we used the band-resolved method to further explain the contributions of orbitals to the SHG response. The virtual-electron (VE) and virtual-hole (VH) contributions to the total SHG coefficients were obtained. Based on the band-resolved results shown in Fig. 7, these VE and VH contributions are 61.6% and 38.4%, respectively. It indicates that the SHG effect may be due to the VE and VH processes.
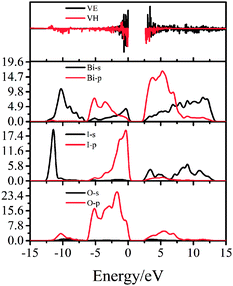 |
| Fig. 7 The PDOS and band-resolved VE and VH processes. | |
4. Conclusions
In summary, exploration of the versatility of materials to increase the utilization of materials is a hot research topic nowadays. Herein, Bi4O5I2 powders have been fabricated via a facile solvothermal method. Under the light irradiation for 60 min, 94% of MO and MB were degraded over Bi4O5I2 samples. It is noteworthy that for the degradation of methyl orange, the photocatalytic activity of Bi4O5I2 is about 10 times that of BiOI. Due to the action of dipole moments, charge separation of the photogenerated electron–hole pairs occurs and thus enhances the photocatalytic activity. In addition, increased dipole moment due to the stereochemical activity of lone pairs induces a high second harmonic generation response. Moreover, theoretical calculation and experiment results reveal that Bi4O5I2 would have potential applications in photocatalytic and NLO fields.
Conflicts of interest
There are no conflicts of interest to declare.
Acknowledgements
This work is financially supported by the National Natural Science Foundation (21463002).
References
- A. Fujishima and K. Honda, Nature, 1972, 238, 238–245 CrossRef.
- S. N. Frank and A. J. Bard, J. Am. Chem. Soc., 1977, 99, 303–304 CrossRef CAS.
- X. Bai, R. Zong, C. Li, D. Liu, Y. Liu and Y. Zhu, Appl. Catal., B, 2014, 147, 82–91 CrossRef CAS.
- C. Liu and X. Wang, Dalton Trans., 2016, 45, 7720–7727 RSC.
- L. Shan, Y. Liu, H. Chen, Z. Wu and Z. Han, Dalton Trans., 2017, 46, 2310–2321 RSC.
- Y. Huang, B. Long, H. Li, M. Balogun, Z. Rui, Y. Tong and H. Ji, Adv. Mater. Interfaces, 2015, 2, 1500249 CrossRef.
- Y. Huang, H. Xu, H. Yang, Y. Lin, H. Liu and Y. Tong, ACS Sustainable Chem. Eng., 2018, 6, 2751–2757 CrossRef CAS.
- W. Zhong, S. Shen, S. Feng, Z. Lin, Z. Wang and B. Fang, CrystEngComm, 2018, 20, 7851–7856 RSC.
- X. Xiao, C. Zheng, M. Lu, L. Zhang, F. Liu, X. Zuo and J. Nan, Appl. Catal., B, 2018, 228, 142–151 CrossRef CAS.
- Y. Huang, H. Li, M. Balogun, W. Liu, Y. Tong, X. Lu and H. Ji, ACS Appl. Mater. Interfaces, 2014, 6, 22920–22927 CrossRef CAS PubMed.
- W. Fan, H. Li, F. Zhao, X. Xiao, Y. Huang, H. Ji and Y. Tong, Chem. Commun., 2016, 52, 5316–5319 RSC.
- Y. Huang, H. Li, W. Fan, F. Zhao, W. Qiu, H. Ji and Y. Tong, ACS Appl. Mater. Interfaces, 2016, 8, 27859–27867 CrossRef CAS PubMed.
- Y. Huang, H. Hu, S. Wang, M. Balogun, H. Ji and Y. Tong, Appl. Catal., B, 2017, 218, 700–708 CrossRef CAS.
- C. Wang, X. Zhang, X. Song, W. Wang and H. Yu, ACS Appl. Mater. Interfaces, 2016, 8, 5320–5326 CrossRef CAS PubMed.
- Z. Li, M. Wang, J. Shen, Z. Zhu and Y. Liu, RSC Adv., 2016, 6, 30037–30047 RSC.
- B. B. Adormaa, W. K. Darkwah and Y. Ao, RSC Adv., 2018, 8, 33551–33563 RSC.
- X. Jing, X. Peng, Y. Cao, W. Wang and S. Wang, RSC Adv., 2018, 8, 33993–33999 RSC.
- F. Ma, Q. Yang, Z. Wang, Y. Liu, J. Xin, J. Zhang, Y. Hao and L. Li, RSC Adv., 2018, 8, 15853–15862 RSC.
- S. Garg, M. Yadav, A. Chandra, S. Sapra, S. Gahlawat, P. P. Ingole, Z. Pap and K. Hernadi, RSC Adv., 2018, 8, 29022–29030 RSC.
- W. Zhong, W. Tu, S. Feng and A. Xu, J. Alloys Compd., 2019, 772, 669–674 CrossRef CAS.
- Z. Lin, C. Wang, Z. Wang, Q. Liu, C. Le, B. Lin and S. Chen, Electrochim. Acta, 2019, 294, 142–147 CrossRef CAS.
- E. Keller, V. Krämer, M. Schmidt and H. Oppermann, Z. Kristallogr. - Cryst. Mater., 2002, 217, 256–264 CAS.
- X. Xiao and W. Zhang, RSC Adv., 2011, 1, 1099 RSC.
- W. W. Lee, C.-S. Lu, C.-W. Chuang, Y.-J. Chen, J.-Y. Fu, C.-W. Siao and C.-C. Chen, RSC Adv., 2015, 5, 23450–23463 RSC.
- Y. Bai, T. Chen, P. Wang, L. Wang and L. Ye, Chem. Eng. J., 2016, 304, 454–460 CrossRef CAS.
- C. Ding, L. Ye, Q. Zhao, Z. Zhong, K. Liu, H. Xie, K. Bao, X. Zhang and Z. Huang, J. CO2 Util., 2016, 14, 135–142 CrossRef CAS.
- Y. Su, C. Ding, Y. Dang, H. Wang, L. Ye, X. Jin, H. Xie and C. Liu, Appl. Surf. Sci., 2015, 346, 311–316 CrossRef CAS.
- X. Wang, Y. Wang, B. Zhang, F. Zhang, Z. Yang and S. Pan, Angew. Chem., 2017, 129, 14307–14311 CrossRef.
- X. Xiao, C. Liu, R. Hu, X. Zuo, J. Nan, L. Li and L. Wang, J. Mater. Chem., 2012, 22, 22840 RSC.
- X. Xiao, C. Xing, G. He, X. Zuo, J. Nan and L. Wang, Appl. Catal., B, 2014, 148–149, 154–163 CrossRef CAS.
- H. Wu, S. Pan, K. R. Poeppelmeier, H. Li, D. Jia, Z. Chen, X. Fan, Y. Yang, J. M. Rondinelli and H. Luo, J. Am. Chem. Soc., 2011, 133, 7786–7790 CrossRef CAS PubMed.
- H. Yu, H. Wu, S. Pan, Z. Yang, X. Su and F. Zhang, J. Mater. Chem., 2012, 22, 9665–9670 RSC.
- H. Wu, H. Yu, Z. Yang, X. Hou, X. Su, S. Pan, K. R. Poeppelmeier and J. M. Rondinelli, J. Am. Chem. Soc., 2013, 135, 4215–4218 CrossRef CAS PubMed.
- X. Dong, Q. Jing, Y. Shi, Z. Yang, S. Pan, K. R. Poeppelmeier, J. Young and J. M. Rondinelli, J. Am. Chem. Soc., 2015, 137, 9417–9422 CrossRef CAS PubMed.
- G. Shi, Y. Wang, F. Zhang, B. Zhang, Z. Yang, X. Hou, S. Pan and K. R. Poeppelmeier, J. Am. Chem. Soc., 2017, 139, 10645–10648 CrossRef CAS PubMed.
- M. Mutailipu, M. Zhang, B. Zhang, L. Wang, Z. Yang, X. Zhou and S. Pan, Angew. Chem., Int. Ed., 2018, 57, 6095–6099 CrossRef CAS PubMed.
- B. Zhang, G. Shi, Z. Yang, F. Zhang and S. Pan, Angew. Chem., Int. Ed., 2017, 56, 3916–3919 CrossRef CAS PubMed.
- X. Fan, L. Zang, M. Zhang, H. Qiu, Z. Wang, J. Yin, H. Jia, S. Pan and C. Wang, Chem. Mater., 2014, 26, 3169–3174 CrossRef CAS.
- X. Fan, K. Lai, L. Wang, H. Qiu, J. Yin, P. Zhao, S. Pan, J. Xu and C. Wang, J. Mater. Chem., 2015, 3, 12179–12187 RSC.
- X. Fan, Z. Wu, L. Wang and C. Wang, Chem. Mater., 2016, 29, 639–647 CrossRef.
- S. J. Clark, M. D. Segall, C. J. Pickard, P. J. Hasnip, M. I. J. Probert, K. Refson and M. C. Payne, Z. Kristallogr. - Cryst. Mater., 2005, 220, 567–570 CAS.
- J. P. Perdew and A. Zunger, Phys. Rev. B, 1981, 23, 5048–5079 CrossRef CAS.
- X. Zhang, Z. Ai, F. Jia and L. Zhang, J. Phys. Chem. C, 2008, 112, 747–753 CrossRef CAS.
- W. Huang, J. Comput. Chem., 2009, 30, 1882–1891 CrossRef CAS PubMed.
- S. Qu, Y. Xiong and J. Zhang, Sep. Purif. Technol., 2019, 210, 382–389 CrossRef CAS.
- L. Li, L. Xu, W. Shi and J. Guan, Int. J. Hydrogen Energy, 2013, 38, 816–822 CrossRef CAS.
- J. Xia, J. Di, S. Yin, H. Li, L. Xu, Y. Xu, C. Zhang and H. Shu, Ceram. Int., 2014, 40, 4607–4616 CrossRef CAS.
- J. Cao, B. Xu, B. Luo, H. Lin and S. Chen, Catal. Commun., 2011, 13, 63–68 CrossRef CAS.
- X. Bai, L. Wang and Y. Zhu, ACS Catal., 2012, 2, 2769–2778 CrossRef CAS.
- Z. Yang, C. Hu, M. Mutailipu, Y. Sun, K. Wu, M. Zhang and S. Pan, J. Mater. Chem. C, 2018, 6, 2435–2442 RSC.
Footnote |
† Electronic supplementary information (ESI) available: EDS patterns of BiOI (a) and Bi4O5I2 (b) samples. Photocatalytic activity of black (without light), Bi4O5I2, and TiO2 for degradation of MO under visible light irradiation (a), effect of MO initial concentration on the photocatalytic performances of Bi4O5I2 (b), photocatalytic degradation of MO, RhB, MB over BiOI and Bi4O5I2 samples, respectively (c), photocatalytic activity of Bi4O5I2 for degradation of norfloxacin under visible light irradiation (d). Free radicals trapping experiment of Bi4O5I2 photocatalysts under visible light irradiation (a), recycling property of Bi4O5I2 photocatalyst (b), PL spectra of BiOI and Bi4O5I2 (c), Mott–Schottky plots of the Bi4O5I2 samples (d). Pseudo-first-order kinetics parameter of MO. The dipole moment calculation and which of BiOmIn (m = 4, n = 4; m = 5, n = 3; m = 6, n = 1; m = 3, n = 4; m = 3, n = 5) polyhedra in Bi4O5I2. Dipole moments of BiO4I4, BiO5I3, BiO6I, Bi(1)O3I4, BiO3I5 polyhedra in Bi4O5I2 crystal. See DOI: 10.1039/c8ra08984a |
|
This journal is © The Royal Society of Chemistry 2019 |