DOI:
10.1039/C8RA07832G
(Paper)
RSC Adv., 2019,
9, 1882-1888
Absorption and thermodynamic properties of CO2 by amido-containing anion-functionalized ionic liquids†
Received
20th September 2018
, Accepted 7th January 2019
First published on 14th January 2019
Abstract
In this contribution, two kinds of amido-containing anion-functionalized ionic liquids (ILs) were designed and synthesized, where the anions of these ILs were selected from deprotonated succinimide (H-Suc) and o-phthalimide (Ph-Suc). Then, these functionalized ILs were used to capture CO2. Towards to this end, solubility of CO2 in the ILs was determined at different temperatures and different CO2 partial pressures. Based on these data, chemical equilibrium constants of CO2 with the ILs were derived at different temperatures from the “deactivated IL” model. The other thermodynamic properties such as reaction Gibbs energy, reaction enthalpy, and reaction entropy in the absorption were also calculated from the corresponding equilibrium constant data at different temperatures. It was shown that these anion-functionalized ILs exhibited high CO2 solubility (up to 0.95 mol CO2 mol−1 IL) and low energy desorption, and enthalpy change was the main driving force for CO2 capture by using such ILs as absorbents. In addition, the interactions of CO2 with the ILs were also investigated by 1H NMR, 13C NMR, and FT-IR spectroscopy.
1. Introduction
In recent decades, the excessive accumulation of carbon dioxide (CO2) in the atmosphere has attracted widespread concern due to the greenhouse effect.1 In order to solve this problem, carbon capture and storage (CCS) through sustainable and green methods has become a hot topic in recent decades.2 Although aqueous alkanolamine solutions have been used in industry for nearly a century as a kind of chemical absorbent for CO2 in flue gas from power plants through the formation of ammonium carbamate, the main disadvantage of this process is the high energy consumption for the regeneration and recycling of the absorbent, which accounts for 30% of the energy of the power plant.3,4 Thus, alternative CCS methods for highly efficient and reversible capture of CO2 are desired.
In recent years, considerable attention has been focused on using ionic liquids (ILs), especially functionalized ILs, as a kind of competitive alternative green absorbent for CO2 capture. These liquid materials provide some unique advantages such as reduced volatilization and improved regeneration.5–9 In light of the reaction mechanism of CO2 in alkanolamine solutions and the outstanding properties of ILs, Davis et al.10 first reported imidazolium ILs with amino-grafted cation for the chemical absorption of CO2. Since the anion plays a key role in carbon capture, worldwide researchers have developed many kinds of task-specified ILs with the functionalized anions such as amino acids,11–14 azolates,15–20 phenolates,7,21–23 and acetate.24 Compared with conventional ILs, these anion-functionalized ILs typically have high CO2 absorption capacity and selectivity. It is highly encouraged that novel kinds of other anion-functionalized ILs should be developed and used in CCS process.
Thermodynamic properties of CO2 – functionalized IL systems are essential to guide the design of new functionalized ILs for CO2 capture. Up to now, great efforts have been devoted to the studies on the thermodynamic properties of CO2 chemical absorption by functionalized ILs. For instance, Brennecke et al.25,26 proposed “deactivated IL” model and “two-reaction” model to analyze the thermodynamic properties of CO2 in amine-functionalized ILs. Wang et al.27 reported that anion-functionalized [P66614][p-AA] and [P66614][p-ANA] had higher absorption capacity and lower reaction enthalpy because of entropic effects. Hu et al.28 systematically studied the thermodynamic properties of CO2 capture in low-viscous fluorine-substituted phenolic ILs through the “deactivated IL” model. Thus, it is very important to expand the thermodynamic studies of different types of functionalized ILs.
In this work, we designed and synthesized two kinds of amido-containing anion-functionalized ILs (see Fig. 1) for CO2 capture. Solubility of CO2 in these functionalized ILs was determined at different temperatures and different CO2 partial pressures. From these data, the equilibrium constants for the reaction of CO2 with these ILs were calculated from a modified “deactivated IL” model as a function of temperature. Then, the Gibbs energy, enthalpy and entropy change were reported for the process of CO2 capture. It was shown that high CO2 absorption capacity and low energy consumption regeneration of the ILs could be achieved by these anion-functionalized ILs. From the viewpoint of chemical thermodynamic, enthalpy change is the main driving force for CO2 capture by using such ILs as absorbents.
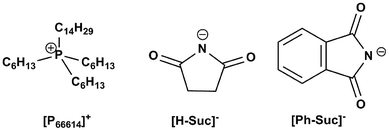 |
| Fig. 1 Chemical structures of ILs used in this work for CO2 capture. | |
2. Experimental section
2.1. Materials
CO2 and N2 were purchased from Beijing Oxygen Plant Specialty Gases Institute Co., Ltd. with a purity of 99.999% and 99.9993%, respectively. Trihexyl(tetradecyl)phosphonium bromide ([P66614][Br], 97%) and o-phthalimide (Ph-Suc, 98%) were obtained from J&K Scientific, while succinimide (H-Suc) was supplied by Sigma-Aldrich. An anion-exchange resin (Amersep 900 OH) was purchased from Alfa Aesar. All these substances were used as received.
2.2. Preparation of the ionic liquids
Anion-functionalized ILs were simply produced through the neutralization of different kinds of acylamides and an ethanol solution of trihexyl (trtradecyl)phosphonium hydroxide ([P66614][OH]) at room temperature according to the procedures described in literature,29,30 where [P66614][OH] was prepared from [P66614][Br] by anion-exchange method using ethanol as the solvent.31 In a typical synthesis of [P66614][H-Suc], equimolar H-Suc was added to the ethanol solution of [P66614][OH], and the mixture was then stirred at room temperature for 24 h. Then, ethanol and water were evaporated at 333 K under reduced pressure. The as-prepared [P66614][H-Suc] was dried with P2O5 under vacuum at 333 K for 24 h to remove possible residual moisture before use. The chemical structures of these ILs were confirmed by 1H NMR, 13C NMR and FT-IR spectra, and the data were listed in the ESI.†
2.3. Determination of CO2 solubility
Solubility data of CO2 in these ILs were measured by gravimetric method.32 In a typical measurement, CO2 was bubbled through about 1.0 g IL loaded in a glass container with an inside diameter of 12 mm, and the gas flow rate monitored by gas rotameter was about 60 ml min−1. The glass container was partly immersed in a water bath which was maintained at the given temperature with temperature uncertainty of ± 0.1 K. During gas absorption, weight of the sample was determined at regular intervals by an electronic balance with an accuracy of ± 0.1 mg until it became constant. At this stage, the adsorption equilibrium of CO2 in the IL was reached, and the solubility of CO2 in the IL could be calculated. For the absorption of CO2 under different partial pressure, CO2 was diluted by N2 gas and the given partial pressure was produced by controlling the flow rate ratio of CO2 and N2.
2.4. Characterization of the ionic liquids
1H NMR and 13C NMR spectra were determined on a Bruker spectrometer (400 MHz) in DMSO-d6 with tetramethylsilane (TMS) as the standard. FT-IR spectra were recorded using a Nicolet 470 FT-IR spectrometer. The structures of ([P66614][H-Suc] and [P66614][Ph-Suc]) before and after CO2 absorption were confirmed by NMR and FT-IR spectroscopy. The water contents in these ILs after drying, determined with Karl Fischer Titration (Mettler Toledo DL32, Switzerland), was lower than 0.1 wt%. The residual halide content, as determined by combining a Br− selective electrode (Shanghai Precision & Scientific Instrument Co. Ltd.) with a saturated calomel electrode (Shanghai Precision & Scientific Instrument Co. Ltd.), was less than 0.0005 mol per kilogram.
3. Results and discussion
3.1. Solubilities of CO2 in the ionic liquids
At the beginning, solubility of CO2 in these ionic liquids was measured at 308.15 K under atmospheric pressure. The results showed that up to 0.95 mol CO2 per mol of IL could be achieved by these functionalized ILs, indicating good absorption performance of the absorbents. Thus, the solubility of CO2 in these ILs was determined at 308.15 K, 313.15 K, 318.15 K, and 323.15 K under different CO2 partial pressures. Table S1 and S2 (see ESI†) listed the solubility data of CO2 in [P66614][H-Suc] and [P66614][Ph-Suc] at various temperatures and CO2 partial pressures. For the sake of easy understanding, these data were shown in Fig. 2 and 3, respectively. It can be seen that the solubility of CO2 in the ILs increased with increasing partial pressure in the range of low pressure. For instance, the solubility of CO2 in [P66614][H-Suc] at 308.15 K was 0.61 mol CO2 mol−1 IL under 10 kPa of CO2 partial pressure, while it increased to 0.95 mol CO2 mol−1 IL under 100 kPa of CO2 partial pressure. However, Fig. 2 and 3 exhibited a nonlinear absorption trend with the increase of CO2 partial pressure. This indicates that the absorption of CO2 in these ILs was mainly carried out through chemical absorption. On the other hand, when the temperature increased from 308.15 K to 323.15 K, the molar ratio of CO2 to [P66614][H-Suc] reduced from 0.61 to 0.14 under 10 kPa of CO2 (Fig. 2). This result suggests that the captured CO2 could be facilely released by mild heating. Thus, the CO2 absorption process by these ILs was characterized by high CO2 absorption capacity and low energy desorption.
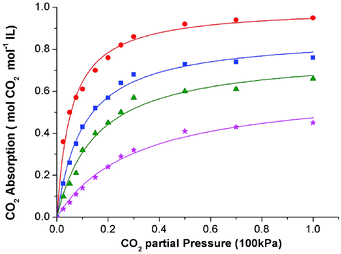 |
| Fig. 2 The absorption isotherms of the CO2-[P66614][H-Suc] system at different temperatures: 308.15 K, (●) 313.15 K, (■) 318.15 K, (▲) 323.15 K, (★) the curves were fittings from eqn (4). | |
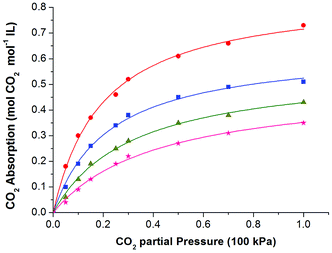 |
| Fig. 3 The absorption isotherms of the CO2-[P66614][Ph-Suc] system at different temperatures: 308.15 K, (●) 313.15 K, (■) 318.15 K, (▲) 323.15 K, (★) the curves were fittings from eqn (4). | |
3.2. Chemical absorption mechanism of CO2 in the ionic liquids
The interaction between CO2 and [P66614][H-Suc] was studied by 1H NMR, 13C NMR, and FT-IR spectra, and the results were illustrated in Fig. 4. Comparing the 1H NMR spectrum of CO2-IL with that of neat IL, it was found that the peak of 2× CH2 in [H-Suc]− at δ = 2.05 ppm was shifted downfield to δ = 2.24 ppm after saturation of CO2 (Fig. 4a), indicating the strong interaction between CO2 and the anion of [P66614][H-Suc]. In the 13C NMR spectrum of CO2-IL, a new peak appeared at 158.1 ppm after CO2 uptake, this could be attributed to the formation of carbamate carbonyl carbon in N–CO2 interaction (Fig. 4b).15,33 On the other hand, when 0.2 to 0.8 mol CO2 was absorbed by [P66614][H-Suc], a new characteristic peak at 1628 cm−1 could be observed in the FT-IR spectrum of [P66614][H-Suc] (Fig. 4c), which belongs to the asymmetrical stretching vibration of N–CO2 due to the chemical interaction between CO2 and the electronegative N atom in [H-Suc]− of the [P66614][H-Suc].34
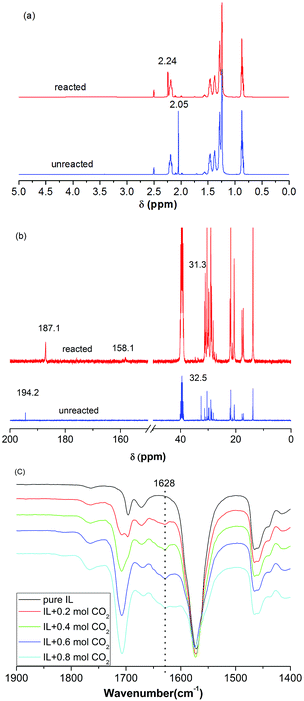 |
| Fig. 4 1H NMR (a), 13C NMR (b) and FT-IR (c) spectra of [P66614][H-Suc] before and after the absorption of CO2. | |
The NMR and FT-IR spectra of [P66614][Ph-Suc] before and after the absorption of CO2 were showed in Fig. S1.† It can be seen that chemical shift of the protons in [Ph-Suc]− from δ = 7.30–7.46 to 7.43–7.47 ppm was observed after CO2 absorption, while typical carbon peak of C
O in [Ph-Suc]− moved from 185.0 ppm to 178.3 ppm, indicating the existence of chemical interaction between [Ph-Suc]− and CO2. Moreover, the new characteristic peak at δ = 157.9 ppm after the absorption was attributed to the CO2 through the interaction of the N⋯CO2. No peak at about 1620 cm−1 could be seen from FT-IR spectrum after CO2 capture, which is possibly covered by other peaks. However, the peaks at 1619 and 1588 cm−1, which were assigned to the five-membered cyclic imide anion of [P66614][Ph-Suc], was blue shifted to 1769 and 1720 cm−1, respectively, suggesting the interaction between CO2 and N atom in the anion.34
Based on the above solubility data and the spectroscopic investigation of the ILs before and after CO2 capture, the possible mechanisms of CO2 capture by [P66614][H-Suc] and [P66614][Ph-Suc] were proposed and shown in Schemes 1 and S1,† respectively, where the negatively charged N atom in the anions interacted with CO2 and formed the complexes.
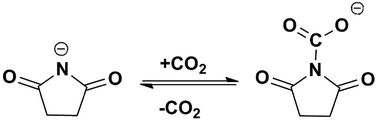 |
| Scheme 1 Possible mechanism of CO2 absorption by [P66614][H-Suc]. | |
3.3. The recycling of ILs for CO2 absorption
Considering the fact that recycling performance of CO2 capture directly influences the application of ILs, we investigated CO2 absorption–desorption cycles by using [P66614][H-Suc] as an example (Fig. 5). It can be seen that the high CO2 absorption capacity and rapid absorption rate were remained during the 6 cycles, indicating that these amido-containing anion-functionalized ILs are highly recyclable.
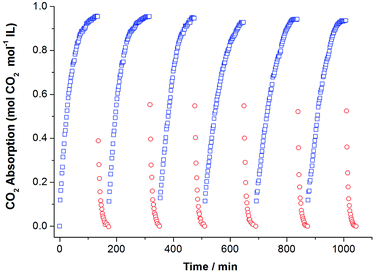 |
| Fig. 5 Six consecutive CO2 absorption–desorption cycles of [P66614][H-Suc]. The absorption of CO2 was performed at 308.15 K and 1.0 bar under CO2 (60 ml min−1). CO2 desorption was carried out at 333.15 K and 1.0 bar under N2 (60 ml min−1). Absorption, (□) desorption, (○). | |
3.4. Thermodynamic properties of CO2 absorbed in the ionic liquids
Thermodynamic properties such as the absorption Gibbs energy, enthalpy and entropy change are of great importance for the evaluation of new absorbents in practical application35 as well as for the understanding of thermodynamic driving force for the absorption.27 For example, absorption enthalpy can reflect the binding strength between the gas and the active site on the absorbent, absorption entropy can be used to probe the change in microstructure of the absorption systems. Thus, the thermodynamic properties of CO2 absorbed in these amido-containing anion-functionalized ILs were derived and analyzed. Usually, the absorption of CO2 in ILs consists of two parts, the physical absorption and the chemical absorption. Thus, the equations for physical CO2 capture and chemical CO2 capture can be expressed as follows: |
CO2 (g) + IL (l) → CO2-IL (l)
| (2) |
where CO2 (g) and CO2 (l) in eqn (1) represent the CO2 in gaseous and liquid states, respectively, while IL (l) and IL-CO2 (l) in eqn (2) stand for the IL before and after CO2 capture. Since our functionalized ILs are basic and they can interact chemically with acid CO2 to form complex, IL-CO2 (l) in eqn (2) may represent such a complex.
Inspired by the previous reports,25,26,28 the CO2 absorption isotherm data in Fig. 2 and 3 were fitted with the “deactivated IL” model developed by Brennecke and co-workers.25 This model assumes that only 1
:
1 reaction takes place and less than 100% of the ionic liquids is allowed to react with CO2. The “deactivated IL” model can be expressed as follows:
|
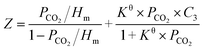 | (3) |
where
Z is the solubility expressed by molar ratio of CO
2 to ionic liquid.
Kθ,
PCO2, and
Hm represent the equilibrium constant (dimensionless parameter), the CO
2 partial pressure in kPa, and the Henry's constant in kPa, respectively.
C3 stands for the molar ratio of active ionic liquids to the total ionic liquids. The first term denotes the contribution from physical absorption, while the second term is the contribution from chemical absorption.
It is known that when the pressure of CO2 is not higher than 100 kPa, the effect of CO2 physical absorption by IL on the absorption isotherms can be ignored.25,26 Considering the fact that our absorption isotherms of CO2 in the amido-containing anion-functionalized ILs were determined under the pressure up to 100 kPa and the CO2 absorption was mainly chemical, it is reasonable to ignore the CO2 physical absorption term in the fitting of absorption isotherms. Therefore, the CO2 absorption system by using these ILs as absorbents can be treated as an ideal chemical reaction system in the studied experimental temperature range, and the following equation of correlation between the solubility of CO2 in these ILs and partial pressure of CO2 could be obtained after simple derivation:
|
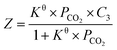 | (4) |
First, the values of the experimental solubility of CO2 in [P66614][H-Suc] and [P66614][Ph-Suc] were fitted to eqn (4) to obtain the values of chemical equilibrium constants at different temperatures, which were shown in the Table 1. It can be seen from Fig. 2 and 3 that the experimental solubility values of CO2 in the studied ILs could be well correlated with the CO2 partial pressure using eqn (4).
Table 1 Equilibrium constants for CO2 absorption in [P66614][H-Suc] and [P66614][Ph-Suc] at different temperatures
IL |
Property |
T (K) |
308.15 |
313.15 |
318.15 |
323.15 |
[P66614][H-Suc] |
Kθ |
18.3 ± 1.4 |
9.7 ± 0.7 |
6.6 ± 0.8 |
3.2 ± 0.3 |
C3 |
1.00 ± 0.02 |
0.87 ± 0.02 |
0.78 ± 0.03 |
0.62 ± 0.03 |
r2 |
0.992 |
0.994 |
0.984 |
0.990 |
[P66614][Ph-Suc] |
Kθ |
5.1 ± 0.3 |
4.5 ± 0.3 |
3.2 ± 0.2 |
2.4 ± 0.2 |
C3 |
0.86 ± 0.02 |
0.64 ± 0.02 |
0.57 ± 0.02 |
0.50 ± 0.02 |
r2 |
0.998 |
0.996 |
0.997 |
0.996 |
Since the temperature range studied in this work is quite narrow, the reaction enthalpy and reaction entropy for the CO2 absorption by the ILs could be determined by using the van't Hoff equation:
|
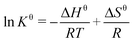 | (5) |
where Δ
Hθ and Δ
Sθ represent the enthalpy and entropy change for the chemical capture of CO
2 in kJ mol
−1 and kJ mol
−1 K
−1, respectively.
T is the thermodynamic temperature in
K,
R denotes the universal gas constant (
R = 8.314 J mol
−1 K
−1). The relationship between the logarithms of the equilibrium constant (
Kθ) and the reciprocal of temperature (1/
T) was shown in
Fig. 6. It can be seen that the linear relationship is reasonable for the CO
2-[P
66614][H-Suc] and CO
2-[P
66614][Ph-Suc] systems. From the slope and intercept of the straight lines, the Δ
Hθ and Δ
Sθ values could be obtained, and the results were given in
Table 2.
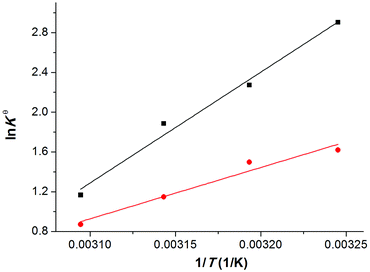 |
| Fig. 6 Linear correlation between ln Kθ and 1/T. [P66614][H-Suc], (■) [P66614][Ph-Suc], (●). | |
Table 2 Molar reaction enthalpy, molar reaction Gibbs energy, and molar reaction entropy for the CO2 absorption by the amido-containing anion-functionalized ILs
IL |
Property |
T (K) |
308.15 |
313.15 |
318.15 |
323.15 |
[P66614][H-Suc] |
ΔGθ (kJ mol−1) |
−7.44 |
−5.92 |
−4.99 |
−3.13 |
ΔHθ (kJ mol−1) |
−92.7 |
103ΔSθ (kJ mol−1 K−1) |
−276.8 |
[P66614][Ph-Suc] |
ΔGθ (kJ mol−1) |
−4.15 |
−3.90 |
−3.03 |
−2.34 |
ΔHθ (kJ mol−1) |
−42.9 |
103ΔSθ (kJ mol−1 K−1) |
−125.2 |
Then, the reaction Gibbs energy (ΔGθ) in kJ mol−1 could be calculated by the following equations:
|
ΔGθ = −RT ln Kθ
| (6) |
The resultant values of ΔGθ were also collected in Table 2. It is clearly noted that ΔHθ values for CO2-[P66614][H-Suc] and CO2-[P66614][Ph-Suc] systems are −92.7 kJ mol−1 and −42.9 kJ mol−1, respectively. The negative values indicate that the capture of CO2 is an exothermic process in [P66614][H-Suc] and [P66614][Ph-Suc] absorbents. Compared with the CO2-[P66614][Ph-Suc] system, ΔHθ value of the CO2-[P66614][H-Suc] system is much lower, suggesting that the interaction between [P66614][H-Suc] and CO2 is much stronger than that between [P66614][Ph-Suc] and CO2. It is also implies that CO2 is more likely difficult to desorb from CO2-[P66614][H-Suc] than CO2-[P66614][Ph-Suc], and more energy consumption is needed in the regeneration of [P66614][H-Suc]. We also calculated the gas-phase reaction enthalpies of [H-Suc]-CO2 and [Ph-Suc]-CO2 complexes using Gaussian 09 program36 by DFT-D3(BJ) at the B3LYP/6-31++G(p,d) level. Although the calculated enthalpies were found to be only −38.4 and −24.3 kJ mol−1 for [H-Suc]-CO2 and [Ph-Suc]-CO2 complexes, respectively, the order was in agreement with the experimental result.
Furthermore, it can be seen from Table 2 that ΔSθ has a negative value in the temperature range investigated, which indicates that the degree of disorder of the system becomes smaller due to the strong interaction of the IL with CO2 molecules and the formation of CO2-anion complexes mentioned above. The values of ΔGθ are negative under the experimental conditions (Table 2), this is a strong indication that CO2 molecules are favourable to dissolve in the amido-containing anion-functionalized ILs. Moreover, considering the fact that ΔGθ, ΔHθ and ΔSθ are all negative, and absolute value of ΔHθ is greater than TΔSθ, the sign of ΔGθ is determined by that of ΔHθ. Therefore, the enthalpy term is predominant for the favourable absorption of CO2.
4. Conclusion
Two kinds of amido-containing anion-functionalized ILs were synthesized, and evaluated for CO2 capture by CO2 solubility measurements at different temperatures and different CO2 partial pressures. It was found that high CO2 absorption capacity (up to 0.95 mol CO2 mol−1 IL) and low energy consumption regeneration of the ILs could be achieved by these anion-functionalized ILs. The interaction of negatively charged N atom in the anions with CO2 and the formation of CO2–anion complexes were responsible for the high absorption capacity. Thermodynamically, reaction enthalpy was the main driving force for CO2 capture. These results are useful for the design of new ionic liquid absorbents for capture of CO2 from flue gas.
Conflicts of interest
There are no conflicts to declare.
Acknowledgements
This work was supported by the National Natural Science Foundation of China (No. U1704251, 21733011), the National Key Research and Development Program of China (No. 2017YFA0403101), the Science Foundation for Excellent Young Scholars of Henan Normal University (No. 15YQ002), and the 111 project (No. D17007). The DFT calculations were supported by the High Performance Computing Center of Henan Normal University.
References
- J. E. Bara, D. E. Camper, D. L. Gin and R. D. Noble, Acc. Chem. Res., 2010, 43, 152–159 CrossRef CAS PubMed.
- G. Cui, J. Wang and S. Zhang, Chem. Soc. Rev., 2016, 45, 4307–4339 RSC.
- J. F. Brennecke and B. E. Gurkan, J. Phys. Chem. Lett., 2010, 1, 3459–3464 CrossRef CAS.
- P. Mores, N. Scenna and S. Mussati, Energy, 2012, 45, 1042–1058 CrossRef CAS.
- V. I. Pârvulescu and C. Hardacre, Chem. Rev., 2007, 107, 2615–2665 CrossRef PubMed.
- X. Li, M. Hou, Z. Zhang, B. Han, G. Yang, X. Wang and L. Zou, Green Chem., 2008, 10, 879–884 RSC.
- X. Luo, Y. Guo, F. Ding, H. Zhao, G. Cui, H. Li and C. Wang, Angew. Chem., Int. Ed., 2014, 126, 7173–7177 CrossRef.
- F. Ding, X. He, X. Luo, W. Lin, K. Chen, H. Li and C. Wang, Chem. Commun., 2014, 50, 15041–15044 RSC.
- M. Tao, J. Gao, W. Zhang, Y. Li, Y. He and Y. Shi, Ind. Eng. Chem. Res., 2018, 57, 9305–9312 CrossRef CAS.
- E. D. Bates, R. D. Mayton, I. Ntai and J. H. Davis, J. Am. Chem. Soc., 2002, 124, 926–927 CrossRef CAS PubMed.
- B. E. Gurkan, J. C. de la Fuente, E. M. Mindrup, L. E. Ficke, B. F. Goodrich, E. A. Price, W. F. Schneider and J. F. Brennecke, J. Am. Chem. Soc., 2010, 132, 2116–2117 CrossRef CAS PubMed.
- S. Saravanamurugan, A. J. Kunov-Kruse, R. Fehrmann and A. Riisager, ChemSusChem, 2014, 7, 897–902 CrossRef CAS PubMed.
- S. Bhattacharyya and F. U. Shah, ACS Sustainable Chem. Eng., 2016, 4, 5441–5449 CrossRef CAS.
- M. Pan, R. Vijayaraghavan, F. Zhou, M. Kar, H. Li, C. Wang and D. R. MacFarlane, Chem. Commun., 2017, 53, 5950–5953 RSC.
- C. Wang, X. Luo, H. Luo, D.-e. Jiang, H. Li and S. Dai, Angew. Chem., Int. Ed., 2011, 123, 5020–5024 CrossRef.
- C. Wang, H. Luo, D.-e. Jiang, H. Li and S. Dai, Angew. Chem., Int. Ed., 2010, 122, 6114–6117 CrossRef.
- X. Zhu, M. Song and Y. Xu, ACS Sustainable Chem. Eng., 2017, 5, 8192–8198 CrossRef CAS.
- B. Gurkan, B. F. Goodrich, E. M. Mindrup, L. E. Ficke, M. Massel, S. Seo, T. P. Senftle, H. Wu, M. F. Glaser, J. K. Shah, E. J. Maginn, J. F. Brennecke and W. F. Schneider, J. Phys. Chem. Lett., 2010, 1, 3494–3499 CrossRef CAS.
- S. Seo, M. Quiroz-Guzman, M. A. DeSilva, T. B. Lee, Y. Huang, B. F. Goodrich, W. F. Schneider and J. F. Brennecke, J. Phys. Chem. B, 2014, 118, 5740–5751 CrossRef CAS PubMed.
- H. Tang and C. Wu, ChemSusChem, 2013, 6, 1050–1056 CrossRef CAS PubMed.
- C. Wang, H. Luo, H. Li, X. Zhu, B. Yu and S. Dai, Chem.–Eur. J., 2012, 18, 2153–2160 CrossRef CAS PubMed.
- M. Vafaeezadeh, J. Aboudi and M. M. Hashemi, RSC Adv., 2015, 5, 58005–58009 RSC.
- M. Pan, N. Cao, W. Lin, X. Luo, K. Chen, S. Che, H. Li and C. Wang, ChemSusChem, 2016, 9, 2351–2357 CrossRef CAS PubMed.
- F.-F. Chen, K. Huang, Y. Zhou, Z.-Q. Tian, X. Zhu, D.-J. Tao, D.-e. Jiang and S. Dai, Angew. Chem., Int. Ed., 2016, 55, 7166–7170 CrossRef CAS PubMed.
- B. F. Goodrich, J. C. de la Fuente, B. E. Gurkan, D. J. Zadigian, E. A. Price, Y. Huang and J. F. Brennecke, Ind. Eng. Chem. Res., 2011, 50, 111–118 CrossRef CAS.
- B. F. Goodrich, J. C. de la Fuente, B. E. Gurkan, Z. K. Lopez, E. A. Price, Y. Huang and J. F. Brennecke, J. Phys. Chem. B, 2011, 115, 9140–9150 CrossRef CAS PubMed.
- X. Y. Luo, F. Ding, W. J. Lin, Y. Q. Qi, H. R. Li and C. M. Wang, J. Phys. Chem. Lett., 2014, 5, 381–386 CrossRef CAS PubMed.
- X.-M. Zhang, K. Huang, S. Xia, Y.-L. Chen, Y.-T. Wu and X.-B. Hu, Chem. Eng. J., 2015, 274, 30–38 CrossRef CAS.
- K. Fukumoto, M. Yoshizawa and H. Ohno, J. Am. Chem. Soc., 2005, 127, 2398–2399 CrossRef CAS PubMed.
- Y. Zhang, S. Zhang, X. Lu, Q. Zhou, W. Fan and X. Zhang, Chem.–Eur. J., 2009, 15, 3003–3011 CrossRef CAS PubMed.
- G. Cui, C. Wang, J. Zheng, Y. Guo, X. Luo and H. Li, Chem. Commun., 2012, 48, 2633–2635 RSC.
- G. Cui, Y. Huang, R. Zhang, F. Zhang and J. Wang, RSC Adv., 2015, 5, 60975–60982 RSC.
- D. Xiong, G. Cui, J. Wang, H. Wang, Z. Li, K. Yao and S. Zhang, Angew. Chem., Int. Ed., 2015, 54, 7265–7269 CrossRef CAS PubMed.
- S. Zhang, Y.-N. Li, Y.-W. Zhang, L.-N. He, B. Yu, Q.-W. Song and X.-D. Lang, ChemSusChem, 2014, 7, 1484–1489 CrossRef CAS PubMed.
- Y. Bian, S. Shen, Y. Zhao and Y.-n. Yang, J. Chem. Eng. Data, 2016, 61, 2391–2398 CrossRef CAS.
- M. J. Frisch, G. W. Trucks, H. B. Schlegel, G. E. Scuseria, M. A. Robb, J. R. Cheeseman, G. Scalmani, V. Barone, B. Mennucci, G. A. Petersson, H. Nakatsuji, M. Caricato, X. Li, H. P. Hratchian, A. F. Izmaylov, J. Bloino, G. Zheng, J. L. Sonnenberg, M. Hada, M. Ehara, K. Toyota, R. Fukuda, J. Hasegawa, M. Ishida, T. Nakajima, Y. Honda, O. Kitao, H. Nakai, T. Vreven, J. A. J. Montgomery, J. E. Peralta, F. Ogliaro, M. Bearpark, J. J. Heyd, E. Brothers, K. N. Kudin, V. N. Staroverov, T. Keith, R. Kobayashi, J. Normand, K. Raghavachari, A. Rendell, J. C. Burant, S. S. Iyengar, J. Tomasi, M. Cossi, N. Rega, J. M. Millam, M. Klene, J. E. Knox, J. B. Cross, V. Bakken, C. Adamo, J. Jaramillo, R. Gomperts, R. E. Stratmann, O. Yazyev, A. J. Austin, R. Cammi, C. Pomelli, J. W. Ochterski, R. L. Martin, K. Morokuma, V. G. Zakrzewski, G. A. Voth, P. Salvador, J. J. Dannenberg, S. Dapprich, A. D. Daniels, O. Farkas, J. B. Foresman, J. V. Ortiz, J. Cioslowski and D. J. Fox, Gaussian 09 (Revision D.01), Gaussian, Inc., Wallingford CT, 2013 Search PubMed.
Footnote |
† Electronic supplementary information (ESI) available: NMR and IR data of the acylamido-based ILs, Tables S1 and S2, Fig. S1 and Scheme S1. See DOI: 10.1039/c8ra07832g |
|
This journal is © The Royal Society of Chemistry 2019 |