DOI:
10.1039/C8RA07645F
(Paper)
RSC Adv., 2019,
9, 993-1003
In situ phytoremediation of copper and cadmium in a co-contaminated soil and its biological and physical effects
Received
14th September 2018
, Accepted 17th December 2018
First published on 9th January 2019
Abstract
Phytoremediation is a potential cost-effective technology for remediating heavy metal-contaminated soils. This method was used to evaluate the biomass and accumulation of copper (Cu) and cadmium (Cd) of plant species grown in contaminated soil and their biological and physical effects on the soil. In co-contaminated soils with copper (Cu) and cadmium (Cd), a three-year field experiment was conducted by planting four plant species in the co-contaminated acidic soil treated with hydroxyapatite. The four species produced different amounts of biomass in this order: Pennisetum sp. > Elsholtzia splendens > Setaria lutescens > Sedum plumbizincicola. Over three growing seasons, the best accumulators of Cu and Cd were Elsholtzia splendens and Sedum plumbizincicola, respectively. Overall, Pennisetum sp. was the best species for Cu and Cd removal when biomass was considered. The four plant treatments could improve the content of >0.25 mm mechanically stable (DR0.25) and water-stable (WR0.25) aggregates and significantly improve the aggregate mean mass diameter (MWD) and the geometric mean diameter (GMD). The largest increase was with the treatment of Pennisetum sinese, while the fractal dimension (FD) of mechanically stable aggregates could be significantly reduced by the treatment of Pennisetum sp. Hydroxyapatite and phytoremediation could improve the soil enzyme activity, and Elsholtzia splendens had the best effect in this respect. This study will provide a better understanding of the remediation of heavy metal contaminated soil.
1. Introduction
Soil contamination, particularly, agricultural soil contamination, has become a severe environmental problem as it poses a grave threat to human health by entering food chains and to environmental safety by leaching into groundwater.1 Cadmium is a non-essential element that can cause great harm to plants and animals at very low concentrations. Excessive cadmium can not only cause serious pollution to soil, reducing crop yield and quality but can also be taken up by humans through the way of soil/crop/food migration, thus endangering human health.2 In fact, some pollutants are nowadays never isolated in the soil system. Cd can be brought into the soil when other heavy metal elements enter the soil. For example, in the process of copper smelting, not only copper but cadmium will also enter the soil together, thus leading to some contaminated soils.3
Various remediation methods, such as land filling, fixation, and leaching, may be beneficial to the remediation of Cu and Cd polluted soils.4 However, these methods are usually expensive, and some of them can impose adverse effects on the biological activity, structure, and fertility of soils.5 Phytoremediation is considered an environmentally friendly, gentle method of managing polluted sites as it uses biological processes to treat the pollutant.6 In contrast to most other remediation technologies, phytoremediation has significant environmental advantages.7 The application of native plants for phytoremediation is particularly important, because they can better adapt to the soil properties, toxicity levels and climatic conditions of the contaminated site.8 Gramineae species usually adapt faster to these conditions than other plant species because their shorter life cycles allow them to produce various genotypes in a shorter time.9 Some experiments have been carried out to investigate the phytoremediation potential of various plants in greenhouse experiments. At the same time, hyperaccumulators are often used, which can grow normally in soils contaminated with heavy metals and accumulate these metals in the harvested parts over the course of phytoremediation.10 In fact, in addition to hyperaccumulators, some plants with large biomass, good adaptability and fast growth rates also show good remediation potential for heavy metals, such as Giantreed and Pennisetum sp.11 However, in the process of remediation, because of the low soil pH, high metal toxicity may impede the growth of plants.12 Results have shown that some materials have good adsorption and stability to Cu and Cd such as ligno-cellulosic materials, Saccharomyces cerevisiae, biosorbents, limestone and hydroxyapatite.13–15 For example, the addition of hydroxyapatite can lead to an increase of soil pH; at the same time, it can reduce the toxicity of heavy metals through ion exchange reactions, surface complexation reactions, co-precipitation and precipitation.10,16 All these processes will promote the growth of plants in polluted areas. For example, Xu et al. found that Pennisetum sp. could grow normally only when lime was applied in the copper and cadmium contaminated soils.17
The aim of phytoremediation should not only be to remove heavy metals but also to improve soil quality.18 Therefore, the comprehensive evaluation of soil quality needs to be considered when evaluating the phytoremediation effect. Soil enzyme activity is the most active constituent; a large number of studies have shown that soil enzyme activity is sensitive to heavy metal pollution because of its significance in nutrient cycles, organic matter turnover, soil characteristics, microbial activity and biomass.19,20 The most frequently measured activities include b-1,4-glucosidase (BG, which catalyzes the terminal reaction in cellulose degradation), b-1,4-N-acetylglucosaminidase (NAG, which catalyzes the terminal reaction in chitin degradation), and acid or alkaline phosphatase (AP, hydrolyze phosphate esters including phosphomonoesters, phosphodiesters and, in some cases, phosphosaccharides that release phosphate), which are frequently linked to the rates of microbial metabolism and biogeochemical processes.21 At the same time, as a basic unit of soil structure, aggregate stability and its influencing factors are important for maintaining good soil structure and soil fertility.22 During phytoremediation, plants increase soil organic matter content through the decomposition of root exudates and litter, thus promoting the formation and stability of aggregates, improving soil physical and chemical properties.23 Therefore, it is necessary to evaluate the composition and structure of soil aggregates which can reflect the changes in soil physical properties during phytoremediation.
The goal of this study was to evaluate the metal removal efficiency of Pennisetum sp. compared with three other kinds of plants in combination with hydroxyapatite application in Cu and Cd contaminated soil. The objectives of this study include: (1) evaluating changes in soil chemical properties and heavy metal availability before and after the combined remediation; (2) determining removal efficiency and removal amount of heavy metals by different plants; (3) measuring the composition and stability of soil aggregates; and (4) measuring the soil enzyme activity.
2. Materials and methods
2.1. Study site
The study site is located in Guixi City, Jiangxi Province, China (117°12′E, 28°19′N). The area is located near a large copper smelter and fertilizer plant. There are about 130 hectares of soil in the area that are contaminated by heavy metals (mainly Cu and Cd) due to 30 years of sewage irrigation.24 At present, most of the soil in the area has been abandoned due to severe pollution, and desertification has appeared in some areas. The soil texture in this area is of sandy loam; the primary pollutants in the soil are Cu and Cd, with concentrations of 632 and 0.41 mg kg−1, respectively. Moreover, the site soil is very acidic (pH = 4.35), having soil organic carbon (SOC) content and cation exchange capacity (CEC) of 28.5 mg kg−1 and 8.31 cmol kg−1, respectively. Total N and total P in the soil were 1.11 g kg−1 and 0.190 g kg−1, respectively.
2.2. Reagent and plants
Hydroxyapatite (particle size = 0.25 mm, pH = 8.40, Cu and Cd concentrations were 9.54 mg kg−1 and 1.18 mg kg−1) was purchased from the Nanzhang Lihua mineral powder factory (Hubei, China). In this experiment, three species of plants were selected based on the high concentrations of Cu and Cd in the soil. We chose a copper tolerant plant (Elsholtzia splendens), a cadmium hyperaccumulator plant (Sedum plumbizincicola), and a kind of energy plant (Pennisetum sp.) that had a good tolerance to Cu and Cd in our previous study. All of the plants were derived from indoor-grown seedlings.
2.3. Experimental design
The field experiment consisted of 5 m (long) × 4 m (wide) plots arranged in a completely random plot design with three replicates per treatment. The treatments had four plants: native plant Setaria lutescens (MW), Elsholtzia splendens (ME), Sedum plumbizincicola (MS), and Pennisetum sp. (MP). A control treatment without plants or added hydroxyapatite (CK) was conducted in parallel. Prior to planting, 1% hydroxyapatite (based on the 0–17 cm soil weight) was applied and fully mixed into the soil by plowing on 23 December 2012. After a week of equilibration, a compound fertilizer (N
:
P2O5
:
K2O = 15
:
15
:
15, 834 kg h m−2) was applied to each plot. The indigenous plants – Setaria lutescens could grow normally after the application of hydroxyapatite. For the other three treatments (ME, MS, and MP), E. splendens, S. plumbizincicola, and Pennisetum sp. were planted on 26 April each year (2013, 2014, and 2015). Weeds (mainly S. lutescens) were cleared from all plots before planting every year, and no weeding was carried out thereafter. The planting density was 20 cm × 20 cm for E. splendens and S. plumbizincicola plants, and 50 cm × 50 cm for Pennisetum sp. plants. All plots were managed using the same field management.
2.4. Sample collection
S. plumbizincicola was harvested in mid-July every year, while the upper part of the other plants were cut out of the ground by a sickle in mid-December every year. All the plant samples were taken to the laboratory and washed with tap water and then rinsed with ultrapure water. They were then put into an oven at 80 °C and dried until the weight no longer changed. Then, the sample was smashed with a grinder and put into a plastic bag. Soil samples were collected from each plot from an area of 20 cm × 20 cm × 17 cm, with three samples taken from each plot and then mixed together to form a mixed sample. These samples were air dried and sieved using a 5 mm sieve, and the resulting samples were used for the analysis of soil aggregates. Soil samples were collected from the top 17 cm at five representative locations per plot and then mixed together to form a composited sample after the plant harvest. The soil samples were divided into two parts: one part was dried and sifted for the analysis of soil physical and chemical properties, and the other part was kept at −80 °C for soil enzyme activity analysis.
2.5. Soil physicochemical and heavy metal analysis
Soil pH was determined based on the method of Xu and was measured using a pH meter (PHS-3CW-CN, Bante instrument, Shanghai, China).10 Soil organic carbon (SOC) was determined by the Walkley–Black procedure.25 Soil available nitrogen (N) and phosphate (P) were determined in the same way as Bingham, and soil available potassium (K) was measured in accordance with Olsen.26,27
Total soil Cu and Cd were measured in accordance with the method used by Cui, and a standard soil sample (GBW07405, National Research Center for Certified Reference Materials, China) was used to ensure the reliability of the experimental data.28 The available Cu and Cd in soils were extracted with 0.01 mol L−1 CaCl2 and determined in accordance with the method of Cui.19
2.6. Aggregates analysis
Mechanically stable aggregates. The mechanically stable aggregates was measured by the dry sieve method.29 The air-dried soil was put on the sieve with apertures of 5 mm, 2 mm, 1 mm, 0.5 mm and 0.25 mm. Then the aggregates were divided into six grades: >5 mm, 2–5 mm, 1–2 mm, 0.5–1 mm, 0.25–0.5 mm, and <0.25 mm. Then the proportion of each grade aggregate to soil weight was calculated based on the results.
Water stable aggregates. The water stable aggregates were measured by the method of Bearden.30 100 g of soil samples were prepared according to the dry sieve ratio, placed in a 5 mm soil sieve and soaked in distilled water for 10 min. The soil samples were then passed through 2 mm, 1 mm, 0.5 mm, and 0.25 mm soil sieves, respectively. The aggregates were separated by moving the sieve 3 cm upward and downward 50 times (2 min); and then, the soil particles on the sieves were rinsed into the aluminum box, dried at 50 °C and weighed.The contents of >0.25 mm mechanically stable aggregates (DR0.25) and >0.25 mm water-stable aggregates (WR0.25) were calculated based on the results. The mean weight diameter (MWD, mm), geometric mean diameter (GMD, mm) and fractal dimension (FD) of aggregates were calculated by Zhao's method.31,32
|
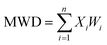 | (1) |
|
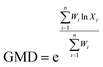 | (2) |
|
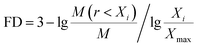 | (3) |
Xi is the average soil aggregate diameter at any level, equal to the average value of the adjacent two sieve hole stages. The upper limit of the diameter of >5 mm aggregates is 10 mm. Xmax is the average particle size of the maximum particle size, mm, M(r < Xi) is the weight of aggregates smaller than Xi, and M is the aggregate weight. With
as abscissa and
as ordinate, the slope is calculated by linear fitting with the least squares method. Finally, the fractal dimension (FD) of mass is calculated from the slope.
2.7. Soil enzyme activity
The activities of b-glucosidase (BG), N-acetylglucosaminidase (NAG), and acid phosphatase (AP) were measured by the method of Saiya-Cork.33 Soils were assayed at ambient pH by suspending approximately 1 g of soil in 100 mL of 50 mm sodium acetate buffer. The microplates were incubated in the dark at 20 °C for 4 h. During the incubation, the incubation plates were shaken every hour to ensure the homogeneity of the reaction mixtures. To stop the reaction, a 1 mL aliquot of 1 M NaOH was added to each well.
2.8. Statistical analysis
All the data were presented as mean ± standard error and were estimated using one-way ANOVA at a significance level of 0.05 using SPSS 20.0 (IBM SPSS, Somers, NY, USA) when necessary. All the graphics in this article were made with Sigmaplot 12.5.
3. Results and discussion
3.1. Soil chemical characteristics and heavy metal availability
In the untreated soil (CK), the soil pH decreased from 4.24 to 4.20 during the three years (Table 1). The soil pH increased significantly from 4.24 to 5.17 when hydroxyapatite was added. This might be due to the high pH (8.40) of hydroxyapatite. This was consistent with Cui et al., who found that the soil pH could be improved from of the addition of hydroxyapatite.28 At the same time, we found that plant growth did not significantly affect the soil pH (Table 1), although the plants might have secreted some weak organic acid ions, amino acids, vitamins, and inorganic ions (HCO3−, OH−, and H+) by the roots, which could change the soil pH.34 In the same way as the CK treatment, we found that the soil pH treated with hydroxyapatite also decreased slightly over time. This may be due to the fact that our experimental area was located in a typical acid rain area and that H+ in the atmosphere entered the soil, thus the reducing of the soil pH. The SOC concentration of the combination treatments (ME, MS, MP) increased significantly compared with CK after three years of remediation (Table 1). However, applying hydroxyapatite alone (MW) had no significant effect on SOC. This might be due to how the growing of plants could increase the amount of litter and fine roots and change the structure of soil aggregates, leading to an increase in SOC content.35 The phosphorus content in each treatment was significantly higher than that in the untreated soil because of the addition of hydroxyapatite. In southern China, phosphorus is generally deficient in soil, and the addition of hydroxyapatite is beneficial to mitigate the adverse effects of phosphorus deficiency in plants.
Table 1 Soil chemical characteristics after the harvest of four plant species during the three years. CK = untreated soil, MW = hydroxyapatite + Setaria lutescens, ME = hydroxyapatite + Elsholtzia splendens, MS = hydroxyapatite + Sedum plumbizincicola, MP = hydroxyapatite + Pennisetum sp. SOC, soil organic carbon; T-N, total nitrogen; T-P total phosphorus; CEC, cation exchange capacity; T-Cu, total Cu concentration; T-Cd, total Cd concentration. Different lowercase letters indicate significant differences between treatments at the same time (n = 3, P < 0.05)
Time |
Treatment |
pH |
SOC g kg−1 |
T-N g kg−1 |
T-P g kg−1 |
CEC cmol kg−1 |
T-Cu mg kg−1 |
T-Cd mg kg−1 |
CaCl2–Cu mg kg−1 |
CaCl2–Cd mg kg−1 |
2013 |
CK |
4.24 ± 0.207b |
16.2 ± 0.191a |
1.11 ± 0.0379a |
0.190 ± 0.0100b |
8.32 ± 0.0153a |
666 ± 16.3a |
0.412 ± 0.0244a |
81.6 ± 25.9a |
0.125 ± 0.0185a |
MW |
5.17 ± 0.118a |
16.3 ± 0.0458a |
1.36 ± 0.0666a |
0.620 ± 0.0872a |
8.41 ± 0.149a |
660 ± 28.1a |
0.400 ± 0.0181a |
28.4 ± 8.88b |
0.081 ± 0.0123b |
ME |
5.33 ± 0.0503a |
17.8 ± 0.398a |
1.32 ± 0.0900a |
0.650 ± 0.0306a |
8.63 ± 1.15a |
618 ± 13.4a |
0.390 ± 0.00913a |
23.2 ± 5.44b |
0.076 ± 0.0133b |
MS |
5.19 ± 0.102a |
18.0 ± 0.508a |
1.26 ± 0.104a |
0.780 ± 0.0723a |
7.84 ± 0.974a |
633 ± 19.3a |
0.377 ± 0.0153a |
22.8 ± 4.28b |
0.066 ± 0.0162b |
MP |
5.15 ± 0.135a |
18.0 ± 1.39a |
1.27 ± 0.173a |
0.710 ± 0.202a |
8.65 ± 0.575a |
657 ± 15.3a |
0.380 ± 0.0169a |
23.4 ± 5.11b |
0.073 ± 0.00932b |
2014 |
CK |
4.23 ± 0.110b |
16.1 ± 0.172b |
1.05 ± 0.0306a |
0.190 ± 0.0379b |
8.39 ± 0.0404a |
674 ± 12.5a |
0.394 ± 0.0142a |
94.2 ± 36.3a |
0.132 ± 0.0283a |
MW |
5.16 ± 0.130a |
16.2 ± 0.118b |
1.40 ± 0.0305a |
0.620 ± 0.0764a |
8.53 ± 0.285a |
664 ± 23.7a |
0.391 ± 0.0155a |
31.1 ± 6.95b |
0.086 ± 0.00768ab |
ME |
5.24 ± 0.261a |
18.4 ± 0.725a |
1.33 ± 0.112a |
0.520 ± 0.0404a |
8.64 ± 0.170a |
621 ± 16.9a |
0.372 ± 0.00811a |
34.5 ± 13.2b |
0.073 ± 1.73 × 10−2b |
MS |
5.13 ± 0.195a |
18.4 ± 0.239a |
1.25 ± 0.123a |
0.680 ± 0.0608a |
8.47 ± 0.180a |
639 ± 29.0a |
0.359 ± 0.0256a |
26.0 ± 13.5b |
0.068 ± 0.0215b |
MP |
5.14 ± 0.253a |
18.8 ± 0.546a |
1.27 ± 0.251a |
0.630 ± 0.242a |
8.56 ± 0.206a |
650 ± 25.2a |
0.352 ± 0.0346a |
25.0 ± 3.30b |
0.075 ± 0.0113b |
2015 |
CK |
4.20 ± 0.280b |
16.5 ± 0.451b |
1.08 ± 0.0600b |
0.190 ± 0.0153b |
8.36 ± 0.140b |
668 ± 11.7a |
0.406 ± 0.0154a |
100.0 ± 21.1a |
0.148 ± 0.0175a |
MW |
5.03 ± 0.137a |
16.6 ± 0.358b |
1.45 ± 0.0379a |
0.510 ± 0.136a |
8.51 ± 0.0929b |
658 ± 24.5a |
0.389 ± 0.0124ab |
49.8 ± 7.48b |
0.119 ± 0.0123ab |
ME |
5.26 ± 0.172a |
19.0 ± 0.593a |
1.46 ± 0.0436a |
0.460 ± 0.0252ab |
8.84 ± 0.0710a |
616 ± 8.67ab |
0.364 ± 0.00526b |
45.9 ± 6.66b |
0.118 ± 0.00747ab |
MS |
5.13 ± 0.161a |
18.6 ± 1.09a |
1.28 ± 0.0751ab |
0.620 ± 0.102a |
8.53 ± 0.0200b |
630 ± 8.53ab |
0.350 ± 0.0120b |
43.5 ± 3.50b |
0.090 ± 0.0211b |
MP |
5.07 ± 0.0971a |
19.5 ± 0.478a |
1.35 ± 0.276ab |
0.550 ± 0.140a |
8.56 ± 0.0819b |
649 ± 13.8b |
0.350 ± 0.0227b |
44.3 ± 9.81b |
0.089 ± 0.0279b |
It is known that the harmfulness of heavy metals in soils is mainly determined by their availability and mobility, CaCl2-extractable heavy metals can be used as an index to measure the availability of heavy metals in soil.36 Our experimental results indicated that untreated soil had the highest CaCl2 extractability (Cu 81.6 mg kg−1, Cd 0.125 mg kg−1 in 2013). The addition of hydroxyapatite significantly reduced the available Cu and Cd in the soil; the lowest extractable Cu by CaCl2 (Cu 43.5 mg kg−1) was in Sedum plumbizincicola plots and Cd by CaCl2 (Cd 0.089 mg kg−1) was in Pennisetum sp. plots (Table 1). The results showed that potential mobility of Cu and Cd in the control was higher than that in hydroxyapatite treated soils, which might be mainly due to the lower pH in the CK.
3.2. Biomass and metal accumulation
In our study, the native S. lutescens and the three phytoextractors were able to grow normally only after hydroxyapatite application. Among the four plants grown, the biomass of Pennisetum sp. was the largest followed by Elsholtzia splendens, Setaria lutescens and Sedum plumbizincicola (Table 2). As a hyperaccumulator of cadmium, Sedum plumbizincicola exhibited a high absorptive capacity for Cu (451.5 mg kg−1) and Cd (13.7 mg kg−1) in our study, which were 13.8 and 11.8 times that of Setaria lutescens, respectively. E. splendens (a Cutolerant plant) also showed a high absorption capacity for Cu and Cd, which reached 202 mg kg−1 and 2.59 mg kg−1, respectively (Table 2, Fig. 1).
Table 2 Shoot biomass and Cu and Cd accumulation in each plant during phytoextraction. CK = untreated soil, MW = hydroxyapatite + Setaria lutescens, ME = hydroxyapatite + Elsholtzia splendens, MS = hydroxyapatite + Sedum plumbizincicola, MP = hydroxyapatite + Pennisetum sp. Different lowercase letters indicate significant differences between treatments in the same year (n = 3, P < 0.05). — indicates no plant growth
Treatment |
Shoot biomass (t dry weight h per m2 per year) |
Metal accumulation (g h per m2 per year) |
|
Cu |
Cd |
2013 |
2014 |
2015 |
2013 |
2014 |
2015 |
2013 |
2014 |
2015 |
CK |
— |
— |
— |
— |
— |
— |
— |
— |
— |
MW |
10.1 ± 4.91bc |
8.55 ± 1.52bc |
5.20 ± 0.560c |
236 ± 148c |
285 ± 20.5b |
224 ± 93.2b |
10.4 ± 3.96b |
10.3 ± 4.27b |
6.50 ± 2.23c |
ME |
15.1 ± 4.17ab |
12.6 ± 1.38b |
14.4 ± 4.22b |
2.74 × 103 ± 437a |
2.54 × 103 ± 759a |
2.93 × 103 ± 1.28 × 103a |
39.2 ± 15.0a |
32.1 ± 8.59a |
37.6 ± 8.49b |
MS |
2.25 ± 0.365c |
2.10 ± 0.210c |
2.70 ± 0.468c |
1.03 × 103 ± 266c |
910 ± 92.8b |
1.28 × 103 ± 395ab |
29.8 ± 3.94ab |
29.5 ± 1.10a |
38.1 ± 5.15ab |
MP |
22.3 ± 3.36a |
29.2 ± 6.10a |
37.7 ± 4.14a |
1.88 × 103 ± 353b |
2.98 × 103 ± 949a |
3.81 × 103 ± 1.40 × 103a |
29.1 ± 4.46ab |
39.8 ± 8.97a |
52.0 ± 3.94a |
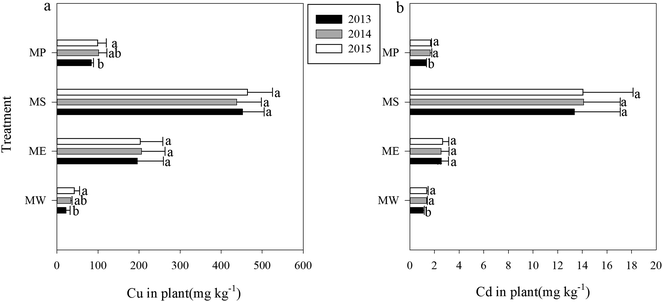 |
| Fig. 1 Concentrations of (a) Cu and (b) Cd in the shoots of each plant. MW = Setaria lutescens, ME = Elsholtzia splendens, MS = Sedum plumbizincicola, MP = Pennisetum sp. Different lowercase letters indicate significant differences in the same treatment during the three years (n = 3, P < 0.05). | |
According to Pedron, phytoremediation can be considered as a successful treatment for soil contaminated with heavy metals if plants are able to reduce soil metal concentration over time through the uptake processes.37 Thus, “removal efficiency” should be calculated using tissue concentration and biomass produced to illustrate the treatment effectiveness of phytoextraction.38 With regard to the total accumulation of Cu and Cd, Pennisetum sp. showed the greatest advantage, with the three-year cumulative amounts of 8.67 × 103 g h m−2 and 121 g h m−2, respectively (Table 2). The integrated results of plant biomass and accumulation capability showed that E. splendens and S. plumbizincicola had similar absolute accumulations of Cu and Cd. The poor biomass and accumulation ability of S. lutescens showed that this native plant species had the lowest absolute accumulation. In terms of absolute accumulation concentration, the remediation efficiency of different plants ranked Pennisetum sp. > E. splendens > S. plumbizincicola > S. lutescens. Based on our data, we would suggest that intercropping S. plumbizincicola with Pennisetum sp. or E. splendens might be a useful approach to removing Cu and Cd from soils. Nevertheless, more experiments are still needed to verify this hypothesis.
3.3. Soil aggregates structure and stability
As a basic component of soil, aggregates play an important role in the transportation of water, nutrients and air in soil. The improvement of soil aggregate stability is conducive to the progress of these processes.39 In the process of evaluating the stability of soil aggregates, the average mass diameter, geometric mean diameter and aggregate stability rate are commonly used indicators. Using these indicators, we can objectively evaluate the stability of soil aggregates.40 The content of the soil with mechanically stable aggregates was 69.4–76.6% before the harvest of vegetation in 2015 (Table 3), which was less than a lot of previous studies.41,42 This indicated that the physical structure of the soil in this area was poor, which might be related to serious soil pollution and difficult growth of plants in the area, resulting in the slight desertification of soil and the deterioration of soil structure.43
Table 3 Effects of phytoremediation on the composition of soil aggregates. CK = untreated soil, MW = hydroxyapatite + Setaria lutescens, ME = hydroxyapatite + Elsholtzia splendens, MS = hydroxyapatite + Sedum plumbizincicola, MP = hydroxyapatite + Pennisetum sp. Different lowercase letters indicate significant differences between treatments in the same year (n = 3, P < 0.05)
Treatment |
The size of soil mechanically stable aggregates |
>5 mm |
5–2 mm |
2–1 mm |
1–0.5 mm |
0.5–0.25 mm |
<0.25 mm |
DR0.25 |
CK |
10.2 ± 0.405b |
26.0 ± 1.19b |
8.34 ± 0.95a |
11.0 ± 1.10b |
13.9 ± 0.493a |
30.2 ± 1.46a |
69.4 ± 1.34b |
MW |
11.2 ± 0.674ab |
27.1 ± 0.322ab |
9.23 ± 0.277a |
13.7 ± 1.23a |
12.0 ± 0.607b |
25.7 ± 1.33b |
73.2 ± 1.09ab |
ME |
10.4 ± 0.356b |
27.6 ± 1.21ab |
9.35 ± 0.815a |
14.1 ± 0.813a |
12.5 ± 0.569b |
25.3 ± 2.35b |
74.0 ± 2.64a |
MS |
11.5 ± 0.654ab |
28.0 ± 1.05ab |
8.83 ± 0.464a |
14.1 ± 0.201a |
12.3 ± 0.222b |
24.6 ± 0.933b |
74.6 ± 0.90a |
MP |
13.1 ± 1.30a |
28.6 ± 0.167a |
8.96 ± 1.27a |
13.2 ± 0.745ab |
12.6 ± 0.628ab |
22.8 ± 1.00b |
76.6 ± 0.73a |
Treatment |
The size of soil water-stable aggregates |
>5 mm |
5–2 mm |
2–1 mm |
1–0.5 mm |
0.5–0.25 mm |
<0.25 mm |
WR0.25 |
CK |
5.18 ± 0.630b |
7.26 ± 1.60a |
6.00 ± 0.265b |
8.29 ± 0.66ab |
18.0 ± 3.39a |
55.3 ± 2.97a |
44.7 ± 2.97b |
MW |
5.25 ± 0.606b |
8.95 ± 0.514a |
6.43 ± 0.594b |
8.21 ± 0.374ab |
19.8 ± 0.974ab |
50.7 ± 1.35ab |
48.7 ± 1.33ab |
ME |
6.41 ± 0.704ab |
8.83 ± 1.55a |
6.68 ± 0.182b |
7.59 ± 1.13b |
22.8 ± 1.39a |
47.7 ± 2.32b |
52.3 ± 2.32a |
MS |
5.32 ± 0.768b |
7.98 ± 1.33a |
6.70 ± 0.101b |
7.00 ± 0.248b |
23.7 ± 2.33a |
49.3 ± 2.27b |
50.7 ± 2.27a |
MP |
7.70 ± 0.887a |
7.49 ± 0.599a |
8.39 ± 0.445a |
9.64 ± 0.130a |
19.3 ± 0.610a |
47.4 ± 1.16b |
52.6 ± 1.16a |
The content of DR0.25 in soil significantly increased after 3 years of remediation for all three kinds of plants; moreover, the 3 plant treatments mainly increased the mechanically stable aggregate content of >5 mm, 2–5 mm and 0.5–1 mm, while especially increasing the content of >2 mm aggregates. This showed that the remediation of the three plants had a significant promoting effect on the formation of soil with >0.25 mm mechanically stable aggregates, which was mainly achieved by increasing the content of the >2 mm mechanically stable aggregates. Among the 3 plants, the maximum increase of >0.25 mm aggregates was Pennisetum sp., especially for the >5 mm and 2–5 mm aggregates, which increased by 29.0% and 10.1% compared with the CK treatment, respectively. The results showed that Pennisetum sp. had the largest increase in mechanically stable aggregates and had the best effect on improving soil physical structure. The percent content of water-stable aggregates for different treatments were 44.7–52.6% (Table 3). Similar to mechanically stable aggregates, all three kinds of plants could significantly increase the content of water-stable aggregates. The increase was mainly concentrated in the >5 mm aggregates, and it was not significant in other particle sizes. This was the same as that of Zheng, who found that the vegetation restoration process mainly increased the content of >5 mm aggregates.44
The mean weight diameter (MWD) and geometric mean diameter (GMD) are important indexes for evaluating the stability of soil aggregates. The increase of MWD and GMD values can represent the increase in soil aggregate stability.45 The MWD and GMD of mechanically stable and water-stable aggregates increased significantly after the 3 years of remediation in our study (Table 4). The MWD and GMD values of both mechanically stable and water-stable aggregates increased to the greatest extent. In terms of MWD, MP treatment increased the amount of mechanically stable and water-stable aggregates by 16.2% and 24.2% compared with CK treatment. In terms of GMD, the GMD of mechanically stable and water-stable aggregates, compared to CK, increased 29.1% and 25.0% by MP treatment, respectively. The results proved again that the application of hydroxyapatite and Pennisetum sp. in the phytoremediation of degraded heavy metal contaminated soil could improve the stability of soil aggregates and the physical structure of the soil. Moreover, the MWD and GMD of the mechanically stable aggregate were greater than those of water-stable aggregates in all the treatments, which indicated that the mechanically stable aggregates were the main aggregate type in the soil. These results were consistent with those of Zhang et al.46
Table 4 Effects of vegetation restoration on the mean weight diameter and geometric mean diameter of mechanically stable and water-stable aggregates in heavy metal contaminated soil. CK = untreated soil, MW = hydroxyapatite + Setaria lutescens, ME = hydroxyapatite + Elsholtzia splendens, MS = hydroxyapatite + Sedum plumbizincicola, MP = hydroxyapatite + Pennisetum sp. Different lowercase letters indicate significant differences between treatments in the same year (n = 3, P < 0.05)
Treatment |
Mean weight diameter (MWD) (mm) |
Geometry weight diameter (GMD) (mm) |
Fractal dimension (FD) |
Mechanical-stable aggregates |
Water-stable aggregates |
Mechanical-stable aggregates |
Water-stable soil aggregates |
Mechanical-stable aggregates |
Water-stable soil aggregates |
CK |
1.98 ± 0.0374c |
0.946 ± 0.0944b |
0.794 ± 0.0264c |
0.324 ± 0.0205b |
3.15 ± 0.0964a |
3.54 ± 0.0563a |
MW |
2.11 ± 0.0382bc |
1.02 ± 0.0593ab |
0.915 ± 0.0303b |
0.358 ± 0.0150ab |
3.10 ± 0.0163b |
3.53 ± 0.0431a |
ME |
2.08 ± 0.0614bc |
1.10 ± 0.0733ab |
0.909 ± 0.0532b |
0.379 ± 0.0220a |
3.09 ± 0.0205b |
3.47 ± 0.0357a |
MS |
2.16 ± 0.0350b |
1.00 ± 0.0917ab |
0.947 ± 0.0237ab |
0.353 ± 0.0216ab |
3.07 ± 0.0139b |
3.52 ± 0.0480a |
MP |
2.30 ± 0.0731a |
1.18 ± 0.0648a |
1.02 ± 0.0287a |
0.398 ± 0.170a |
3.03 ± 0.0148c |
3.44 ± 0.0260a |
Fractal dimension (FD) is a new index to used evaluate the comprehensive soil structure. It can reflect the stability of soil aggregates while reflecting the uniformity of soil texture. The lower the fractal dimension, the more beneficial to the improvement of soil nutrient circulation and structure.47 The study found that the fractal dimension (FD) of mechanically stable aggregates could be significantly reduced by the restoration of 4 planting. The range of the reduction range was 1.59–3.81% (Table 4). This showed that after 3 years of vegetation restoration, the particle size composition of the soil aggregates was more homogeneous and the physical structure of soil had been improved.
Soil organic carbon is an important index for evaluating soil quality, which has an important influence on the formation and cementation of aggregates. As an existing place for soil organic carbon, aggregates play an important role in the storage of organic matter and the transport of water vapor. Therefore, soil organic carbon and aggregates are inseparable.48 After 3 years of vegetation restoration on this heavy metal contaminated soil, the content of soil organic matter and >0.25 mm aggregates increased significantly, while the improvement of Pennisetum sp. treatment which had the highest biomass per unit area was the most significant. Regression analysis showed that soil organic matter content was positively correlated with >0.25 mm mechanically stable aggregates (DR0.25) and water-stable aggregates (WR0.25) (R2 = 0.550*, 0.504*). This result was in accordance with the results of Zhu et al., who found that the main reason for the formation and increase of large aggregates was the increase in organic matter content.49 The restoration of vegetation improved the content of organic matter and organic residues in the soil, and the smaller aggregates in the soil formed a larger aggregate by cementing organic carbon, mycelium nuclei and plant residues in the soil.50
3.4. Soil enzyme activities
Soil enzyme activity is a direct indicator of soil microbial activity in response to metabolic requirements and available nutrients; it is especially useful for evaluating the impact of heavy metal pollution in soil.51 The untreated soil showed very low BG and NAG activities, indicating a poor functional ability to catalyze the decomposition and transformation of soil carbon and nitrogen. The activities of BG, NAG and AP increased significantly by 205%, 114% and 17.4% in E. splendens soil, respectively, as compared with the control (Fig. 2). But the activities of these three enzymes were all at low levels in the Setaria lutescens plot. In contrast to BG and NAG, AP activity was only slightly affected by the treatments except for the E. splendens plots. This might be due to the fact that acid conditions were favorable to acid phosphatase activity.52 Previous studies have reported that heavy metals in soils can inhibit enzyme activities (1) by their toxic effects on soil microflora, (2) by combining with the active groups of the enzymes (3) through the complexation of the substrate, and (4) by reacting with the enzyme–substrate complex.53,54 O. N. Belyaeva et al. found that soil BG and NAG activities increased with decreasing soil bioavailable Cu.55
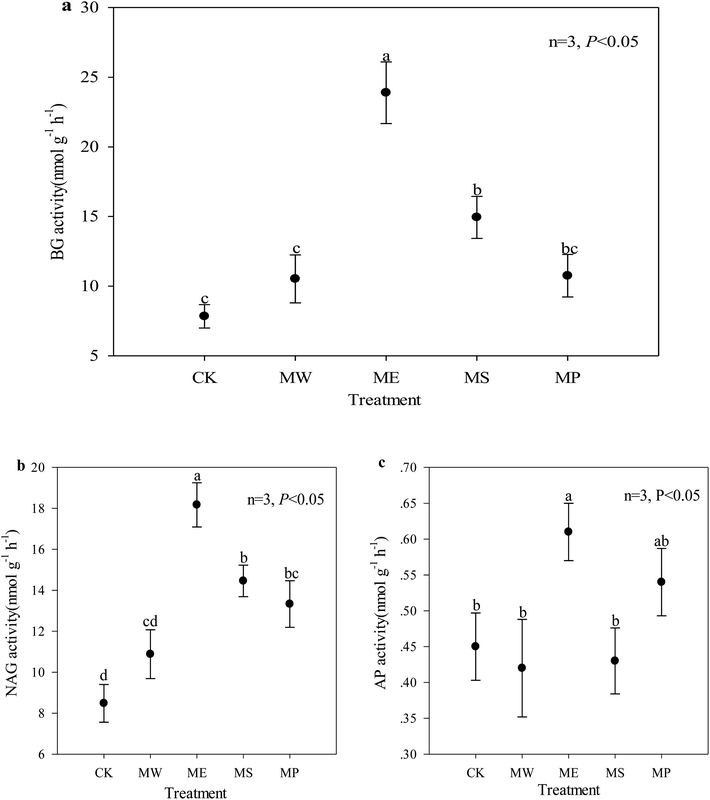 |
| Fig. 2 Soil enzyme activities after the harvest of the four plant species in 2015. (a) BG activity, (b) NAG activity, (c) AP activity. CK = untreated soil, MW = hydroxyapatite + Setaria lutescens, ME = hydroxyapatite + Elsholtzia splendens, MS = hydroxyapatite + Sedum plumbizincicola, MP = hydroxyapatite + Pennisetum sp. BG, b-1,4-glucosidase; NAG, b-1,4-N-acetylglucosaminidase; AP, acid phosphatase. Different lowercase letters indicate significant differences between treatments obtained at the same time. | |
Interestingly, in addition to the significant correlation between NAG activity and CaCl2-extractable copper, there was no significant correlation among the other two enzyme activities and CaCl2-extractable copper and cadmium (Table 5). BG and NAG activity was mainly significantly positively correlated with soil pH and CEC but negatively correlated with total Cu in the soil. The soil pH and cation exchange affect the immobilization of enzymes in the soil.56 The potential for biological activity as indicated by the enzyme activity may be attributed to root residues and root exudates (such as amino acids, sugars, phenolics, polysaccharides and proteins). Such processes could improve soil physical and chemical properties and cause changes in the composition and function of soil microbial communities.57 In our study, soil pH was an important factor that restricted plant growth; the increase of soil pH could promote the activity of soil BG and NAG activity. AP contributes to the transformation of organic phosphorus to inorganic phosphorus, thereby enhancing the absorption of inorganic phosphorus by plants.58 The synthesis of new phosphatase or the phosphatase activity in the soil can be inhibited by inorganic phosphate.59 In this study, the addition of hydroxyapatite led to the increase of phosphate, which could lead to the decrease of AP activity. On the other hand, the addition of hydroxyapatite led to the increase of soil pH and to the reduced toxicity of heavy metals in the soil, which could improve the activity AP. The combined effect resulted in a small increase in the activity of AP (Fig. 2c).
Table 5 Correlation coefficients among soil biological properties, soil chemical properties, and CaCl2-extractable Cu and Cd. (BG, b-1,4-glucosidase; NAG, b-1,4-N-acetylglucosaminidase; AP, acid phosphatase; CEC, cation exchange capacity; T-Cu, total Cu; T-Cd, total Cd; C-Cu, CaCl2-extractable Cu; C-Cd, CaCl2-extractable Cd; SOC, soil organic carbon; T-N, soil total nitrogen; T-P soil total phosphorus. All samples of all plots included in these correlation analyses (n = 18). ** Correlation is significant at the 0.01 level; * correlation is significant at the 0.05 level)
|
BG |
NAG |
AP |
pH |
CEC |
T-Cu |
T-Cd |
C-Cu |
C-Cd |
SOC |
T-N |
T-P |
BG |
1.00 |
|
|
|
|
|
|
|
|
|
|
|
NAG |
0.861* |
1.00 |
|
|
|
|
|
|
|
|
|
|
AP |
0.564* |
0.600* |
1.00 |
|
|
|
|
|
|
|
|
|
pH |
0.607* |
0.793** |
0.271 |
1.00 |
|
|
|
|
|
|
|
|
CEC |
0.848** |
0.836** |
0.537** |
0.698** |
1.00 |
|
|
|
|
|
|
|
T-Cu |
−0.783** |
−0.797** |
−0.296 |
−0.559* |
−0.675** |
1.00 |
|
|
|
|
|
|
T-Cd |
−0.382 |
−0.651** |
−0.428 |
−0.618* |
−0.401 |
0.470 |
1.00 |
|
|
|
|
|
C-Cu |
−0.474 |
−0.645** |
−0.240 |
−0.733** |
−0.464 |
0.599* |
0.742** |
1.00 |
|
|
|
|
C-Cd |
−0.180 |
−0.465 |
−0.135 |
−0.514* |
−0.247 |
0.268 |
0.793** |
0.757** |
1.00 |
|
|
|
SOC |
0.505 |
0.647** |
0.622* |
0.523* |
0.488 |
−0.515* |
−0.702** |
−0.573* |
−0.489 |
1.00 |
|
|
T-N |
0.487 |
0.446 |
0.250 |
0.677** |
0.506 |
−0.310 |
−0.120 |
−0.525* |
−0.0396 |
0.371 |
1.00 |
|
T-P |
0.272 |
0.465 |
−0.0142 |
0.689** |
0.235 |
−0.460 |
−0.565* |
−0.779** |
−0.520* |
0.518* |
0.609* |
1.00 |
4. Conclusions
This study demonstrates the benefits of combining hydroxyapatite application and phytoextraction for the improvement of soil quality when remediating heavy metals. Four plant species were successfully established in the hydroxyapatite-amended soil and produced different amounts of aboveground biomass in the order of Pennisetum sp. > Elsholtzia splendens > Setaria lutescens > Sedum plumbizincicola. Results indicated that Pennisetum sp. was the best species for Cu and Cd removal from the contaminated soils. The application of hydroxyapatite and four plant treatments could improve the content of >0.25 mm mechanically stable (DR0.25) and water-stable (WR0.25) aggregates and the stability of soil aggregates, the largest increase being with the treatment of Pennisetum sinese. In addition, hydroxyapatite and phytoremediation could improve soil enzyme activity, and Elsholtzia splendens had the best effect in this respect. In conclusion, Elsholtzia splendens and Pennisetum sp. may be the best choices for the remediation of this type of heavy metal contaminated soil.
Conflicts of interest
There are no conflicts to declare.
Acknowledgements
The authors acknowledge the PhD Special Project of Nanyang Normal University (2018ZX018), the National Science and Technology Support Plan (2015BAD05B01), the National Natural Science Foundation of China (41571461, 41601340), the Scientific Research and Service Platform Fund of Henan Province (2016151) and the fund for the Scientific and Technological Innovation Team of Water Ecological Security for the water source region of the midline of the South-to-North Diversion Project of Henan Province (17454).
References
- N. Hechmi, A. N. Ben, H. Abdennaceur and N. Jedidi, Phytoremediation potential of maize (Zea mays L.) in co-contaminated soils with pentachlorophenol and cadmium, Int. J. Phytorem., 2013, 15, 703–713 CrossRef CAS PubMed.
- X. Yin, Y. Xu, R. Huang, Q. Huang, Z. Xie, Y. Cai and X. Liang, Remediation mechanisms for Cd-contaminated soil using natural sepiolite at the field scale, Environ. Sci.: Processes Impacts, 2017, 19, 1563–1570 RSC.
- S. K. Gautam, C. Maharana, D. Sharma, A. K. Singh, J. K. Tripathi and S. K. Singh, Evaluation of groundwater quality in the Chotanagpur plateau region of the Subarnarekha river basin, Jharkhand State, India, Sustainability of Water Quality and Ecology, 2015, 6, 57–74 CrossRef.
- K. Lu, X. Yang, G. Gielen, N. Bolan, Y. S. Ok, N. K. Niazi, S. Xu, G. Yuan, X. Chen and X. Zhang, Effect of bamboo and rice straw biochars on the mobility and redistribution of heavy metals (Cd, Cu, Pb and Zn) in contaminated soil, J. Environ. Manage., 2017, 186, 285–292 CrossRef CAS PubMed.
- H. Ali, E. Khan and M. A. Sajad, Phytoremediation of heavy metals--concepts and applications, Chemosphere, 2013, 91, 869–881 CrossRef CAS PubMed.
- S. Greipsson, Phytoremediation, Nature Education Knowledge, 2011, 3, 643–668 Search PubMed.
- M. Vithanage, B. B. Dabrowska, A. B. Mukherjee, A. Sandhi and P. Bhattacharya, Arsenic uptake by plants and possible phytoremediation applications: a brief overview, Environ. Chem. Lett., 2012, 10, 217–224 CrossRef CAS.
- E. Pilonsmits, Phytoremediation, Annu. Rev. Plant Biol., 2005, 56, 15–21 CrossRef CAS PubMed.
- H. M. Conesa, A. B. Moradi, B. H. Robinson, G. Kühne, E. Lehmann and R. Schulin, Response of native grasses and Cicer arietinum to soil polluted with mining wastes: implications for the management of land adjacent to mine sites, Environ. Exp. Bot., 2009, 65, 198–204 CrossRef CAS.
- L. Xu, H. Cui, X. Zheng, Z. Zhu, J. Liang and J. Zhou, Immobilization of copper and cadmium by hydroxyapatite combined with phytoextraction and changes in microbial community structure in a smelter-impacted soil, RSC Adv., 2016, 6, 103955–103965 RSC.
- L. Marchiol, S. Assolari, P. Sacco and G. Zerbi, Phytoextraction of heavy metals by canola (Brassica napus) and radish (Raphanus sativus) grown on multicontaminated soil, Environ. Pollut., 2004, 132, 21–27 CrossRef CAS PubMed.
- T. Pardo, R. Clemente and M. P. Bernal, Effects of compost, pig slurry and lime on trace element solubility and toxicity in two soils differently affected by mining activities, Chemosphere, 2011, 84, 642–650 CrossRef CAS PubMed.
- Y. Huang, C. Yang, Z. Sun, Z. Zeng and H. He, Removal of cadmium and lead from aqueous solutions using nitrilotriacetic acid anhydride modified ligno-cellulosic material, RSC Adv., 2015, 5, 11475–11484 RSC.
- J. Wang and C. Chen, Biosorption of heavy metals by Saccharomyces cerevisiae: a review, Biotechnol. Adv., 2006, 24, 247–451 CrossRef PubMed.
- J. Wang and C. Chen, Biosorbents for heavy metals removal and their future, Biotechnol. Adv., 2009, 27, 195–226 CrossRef CAS PubMed.
- N. Karami, R. Clemente, E. Morenojiménez, N. W. Lepp and L. Beesley, Efficiency of green waste compost and biochar soil amendments for reducing lead and copper mobility and uptake to ryegrass, J. Hazard. Mater., 2011, 191, 41–48 CrossRef CAS PubMed.
- L. Xu, J. Zhou, J. Liang, H. Cui, M. Tao, Z. Tao, Z. Zhu and L. Huang, The remediation potential of Pennisetum sp. On Cu, Cd contaminated soil, Acta Ecol. Sin., 2014, 34, 5342–5348 Search PubMed.
- M. D. Jusselme, E. Miambi, P. Mora, M. Diouf and C. Rouland-Lefèvre, Increased lead availability and enzyme activities in root-adhering soil of Lantana camara during phytoextraction in the presence of earthworms, Sci. Total Environ., 2013, 445, 101–109 CrossRef PubMed.
- H. B. Cui, Y. C. Fan, J. Zhou, Y. Shi, L. Xu, X. T. Guo, Y. B. Hu and L. M. Gao, Availability of soil Cu and Cd and microbial community structure as affected by applications of amendments, China Environ. Sci., 2016, 36, 197–205 Search PubMed.
- A. Pérez-De-Mora, P. Burgos, E. Madejón, F. Cabrera, P. Jaeckel and M. Schloter, Microbial community structure and function in a soil contaminated by heavy metals: effects of plant growth and different amendments, Soil Biol. Biochem., 2006, 38, 327–341 CrossRef.
- S. M. Shahzad, A. Khalid, M. S. Arif, M. Riaz, M. Ashraf and Z. Iqbal, Co-inoculation integrated with P-enriched compost improved nodulation and growth of Chickpea (Cicer arietinum L.) under irrigated and rainfed farming systems, Biol. Fertil. Soils, 2014, 50, 1–12 CrossRef CAS.
- S. Yoshinobu, K. Tomo'Omi, K. Atsushi, O. Kyoichi and O. Shigeru, Experimental analysis of moisture dynamics of litter layers – the effects of rainfall conditions and leaf shapes, Hydrol. Processes, 2004, 18, 3007–3018 CrossRef.
- Q. Y. Wang, J. S. Liu, Y. Wang and H. W. Yu, Accumulations of copper in apple orchard soils: distribution and availability in soil aggregate fractions, J. Soils Sediments, 2015, 15, 1075–1082 CrossRef CAS.
- L. I. Ping, X. Wang, T. Zhang, D. Zhou and H. E. Yuanqiu, Effects of several amendments on rice growth and uptake of copper and cadmium from a contaminated soil, J. Environ. Sci., 2008, 20, 449–455 CrossRef.
- M. K. N. Tiwari, V. Nigam and A. N. Pathak, Effect of potassium and zinc applications on dry-matter production and nutrient uptake by potato variety ‘Kufri chandramukhi’ (Solanum tuberosum L.) in an alluvial soil of Uttar Pradesh, Plant Soil, 1982, 65, 141–147 CrossRef.
- D. L. Sparks, A. L. Page, P. A. Helmke and R. H. Loeppert, Redox measurements of soils, Plast. Reconstr. Surg., 1996, 82, 328–335 Search PubMed.
- S. R. Olsen, Estimation of available phosphorus in soils by extraction with sodium bicarbonate, Institute for Agricultural Research Samaru, 1954 Search PubMed.
- H. B. Cui, J. Zhou, Y. B. Si, J. D. Mao, Q. G. Zhao, G. D. Fang and J. N. Liang, Immobilization of Cu and Cd in a contaminated soil: one- and four-year field effects, J. Soils Sediments, 2014, 14, 1397–1406 CrossRef CAS.
- X. Zhang, L. Chen, B. Fu, Q. Li, X. Qi and Y. Ma, Soil organic carbon changes as influenced by agricultural land use and management: a case study in Yanhuai Basin, Beijing, China, Acta Ecol. Sin., 2006, 26, 3198–3203 CrossRef CAS.
- B. N. Bearden and L. Petersen, Influence of arbuscular mycorrhizal fungi on soil structure and aggregate stability of a vertisol, Plant Soil, 2000, 218, 173–183 CrossRef CAS.
- S. J. Fonte, E. Yeboah, P. Ofori, G. W. Quansah, B. Vanlauwe and J. Six, Fertilizer and residue quality effects on organic matter stabilization in soil aggregates, Soil Sci. Soc. Am. J., 2009, 73, 961–966 CrossRef CAS.
- Y. S. Zhao, C. H. Han, H. G. Zhang, Q. Chen, M. Liu and X. C. Han, Soil Hydrologic Functions of Main Forest Types in Ashi River's Upstream Watershed, J. Soil Water Conserv., 2012, 26, 203–208 Search PubMed.
- K. R. Saiya-Cork, R. L. Sinsabaugh and D. R. Zakb, The effects of long term nitrogen deposition on extracellular enzyme activity in an Acer saccharum forest soil, Soil Biol. Biochem., 2002, 34, 1309–1315 CrossRef CAS.
- F. E. Z. Haichar, C. Santaella, T. Heulin and W. Achouak, Root exudates mediated interactions belowground, Soil Biol. Biochem., 2014, 77, 69–80 CrossRef CAS.
- G. L. Wu, Y. Liu, F. P. Tian and Z. H. Shi, Legumes Functional Group Promotes Soil Organic Carbon and Nitrogen Storage by Increasing Plant Diversity, Land Degradation & Development, 2017, 28, 1336–1344 CrossRef.
- J. H. Huang, S. H. Hsu and S. L. Wang, Effects of rice straw ash amendment on Cu solubility and distribution in flooded rice paddy soils, J. Hazard. Mater., 2011, 186, 1801–1807 CrossRef CAS PubMed.
- F. Pedron, G. Petruzzelli, M. Barbafieri and E. Tassi, Strategies to use phytoextraction in very acidic soil contaminated by heavy metals, Chemosphere, 2009, 75, 808–814 CrossRef CAS PubMed.
- W. M. Jarrell and R. B. Beverly, The dilution effect in plant nutrition studies, Adv. Agron., 1981, 34, 197–224 CAS.
- W. Li, Z. Zheng, T. Li, X. Zhang, Y. Wang, H. Yu, S. He and T. Liu, Effect of tea plantation age on the distribution of soil organic carbon fractions within water-stable aggregates in the hilly region of Western Sichuan, China, Catena, 2015, 133, 198–205 CrossRef CAS.
- K. Sieling, A. Herrmann, B. Wienforth, F. Taube, S. Ohl, E. Hartung and H. Kage, Biogas cropping systems: short term response of yield performance and N use efficiency to biogas residue application, Eur. J. Agron., 2013, 47, 44–54 CrossRef.
- L. I. Jie, X. Y. Yang, B. H. Sun, S. L. Zhang and N. Aamp, Effects of soil management practices on stability and distribution of aggregates in Lou soil, Journal of Plant Nutrition & Fertilizer, 2014, 20, 346–354 Search PubMed.
- Z. Liu, X. Chen, Y. Jing, Q. Li, J. Zhang and Q. Huang, Effects of biochar amendment on rapeseed and sweet potato yields and water stable aggregate in upland red soil, Catena, 2014, 123, 45–51 CrossRef CAS.
- J. S. Xie, Y. S. Yang, G. S. Chen, J. M. Zhu, H. D. Zeng and Z. J. Yang, Effects of vegetation restoration on water stability and organic carbon distribution in aggregates of degraded red soil in subtropics of China, Acta Ecol. Sin., 2008, 28, 702–709 CAS.
- X. B. Zheng, J. B. Fan and J. Zhou, Effects of Biogas Slurry on Soil Organic Matter and Characteristics of Soil Aggregates in Upland Red Earth, Sci. Agric. Sin., 2015, 48, 3201–3210 CAS.
- Z. Sharifi, N. Azadi and G. Certini, Fire and Tillage as Degrading Factors of Soil Structure in Northern Zagros Oak Forest, West Iran, Land Degradation & Development, 2017, 28, 1068–1077 CrossRef.
- P. Zhang, Z. K. Jia, W. Wang, L. U. Wen-Tao, F. Gao and N. Jun-Feng, Effects of Straw Returning on Characteristics of Soil Aggregates in Semi-arid Areas in Southern Ningxia of China, Sci. Agric. Sin., 2012, 45, 513–1520 Search PubMed.
- M. Y. Liu, Q. R. Chang and Y. B. Qi, Fractal characteristics of soil aggregates and micro aggregates in different land use types, Science of Soil and Water Conservation, 2006, 4, 47–51 Search PubMed.
- C. Li, Z. Cao, J. Chang, Y. Zhang, G. Zhu, N. Zong, Y. He, J. Zhang and N. He, Elevational gradient affect functional fractions of soil organic carbon and aggregates stability in a Tibetan alpine meadow, Catena, 2017, 156, 139–148 CrossRef CAS.
- Q. L. Zhu, M. Cheng, A. N. Shao-Shan and Z. J. Xue, Effects of Re-vegetation on Characteristics of Soil Aggregates and Humus in Soil Aggregate in Loess Hilly Region of Southern Ningxia, J. Soil Water Conserv., 2013, 27, 247–256 Search PubMed.
- M. Tamura, V. Suseela, M. Simpson, B. Powell and N. Tharayil, Plant litter chemistry alters the content and composition of organic carbon associated with soil mineral and aggregate fractions in invaded ecosystems, Global Change Biology, 2017, 23, 4002–4018 CrossRef PubMed.
- E. H. Esch, D. Lipson and E. E. Cleland, Direct and indirect effects of shifting rainfall on soil microbial respiration and enzyme activity in a semi-arid system, Plant Soil, 2017, 411, 333–346 CrossRef CAS.
- C. Conn and J. Dighton, Litter quality influences on decomposition, ectomycorrhizal community structure and mycorrhizal root surface acid phosphatase activity, Soil Biol. Biochem., 2000, 32, 489–496 CrossRef CAS.
- J. Kozdrój and J. D. V. Elsas, Response of the bacterial community to root exudates in soil polluted with heavy metals assessed by molecular and cultural approaches, Soil Biol. Biochem., 2000, 32, 1405–1417 CrossRef.
- Q. Y. Wang, D. M. Zhou and C. Long, Microbial and enzyme properties of apple orchard soil as affected by long-term application of copper fungicide, Soil Biol. Biochem., 2009, 41, 1504–1509 CrossRef CAS.
- O. N. Belyaeva, R. J. Haynes and O. A. Birukova, Barley yield and soil microbial and enzyme activities as affected by contamination of two soils with lead, zinc or copper, Biol. Fertil. Soils, 2005, 41, 85–94 CrossRef CAS.
- J. L. Yan, Distribution, activity and ecological indicator function of enzymes in wetland and paddy soils, Nanjing Agricultural University, 2011 Search PubMed.
- P. J. Gregory, Roots, rhizosphere and soil: the route to a better understanding of soil science?, Eur. J. Soil Sci., 2010, 57, 2–12 CrossRef.
- F. Eivazi, Phosphatase in soils, Soil Biol. Biochem., 1977, 9, 167–172 CrossRef CAS.
- A. L. Page, Methods of soil analysis. Part 2. Chemical and microbiological properties, Wi American Society of Agronomy Inc & Soil Science Society of America Inc, 1965 Search PubMed.
|
This journal is © The Royal Society of Chemistry 2019 |
Click here to see how this site uses Cookies. View our privacy policy here.