DOI:
10.1039/C9QM00227H
(Research Article)
Mater. Chem. Front., 2019,
3, 1593-1600
A diphenylamino-substituted cationic cyclometalated Ir(III) complex: its aggregation-induced phosphorescent emission and oxygen sensing properties†
Received
11th April 2019
, Accepted 28th May 2019
First published on 30th May 2019
Abstract
A cationic cyclometalated Ir(III) complex, Ir2, with aggregation-induced phosphorescent emission (AIPE) has been prepared and fully characterized. The effects of the diphenylamino (DPA) group on the photophysical properties, redox properties and oxygen sensing behaviors of the Ir(III) complex were investigated systematically, and compared with non-DPA-substituted complex Ir1. The results show that the introduction of an electron-donating DPA group at the 4-position on the phenyl ring of the cyclometalating ligand affects the HOMO levels of the corresponding Ir(III) complex significantly, resulting in a marked decrease in the energy gap. DPA-substituted Ir2 demonstrates obvious aggregation-induced phosphorescent emission, while non-DPA-substituted Ir1 is AIPE-inert. The phosphorescence oxygen sensing properties of both complexes were studied in polymer films, and fast response time and excellent operational stability were observed. Two-site model fitting results indicate that Ir2 exhibits higher sensitivity to O2 than that of Ir1. The DPA-substituted cationic cyclometalated Ir(III) complex is a potential candidate for efficient luminescent oxygen sensing.
1. Introduction
Cyclometalated Ir(III) complexes have attracted much attention due to their high quantum yields,1,2 large Stokes shifts,3,4 and photo- and thermal stabilities5,6 as well as the easy tunability of their properties.7,8 So far, these complexes have been widely studied for their potential applications in organic electroluminescent devices, photocatalysis, cell imaging, analytical probing and so on.9,10 However, like other transition metal complexes, Ir(III) complexes usually suffer from low luminescence efficiency in the solid or aggregated state, due to aggregation-caused quenching (ACQ),11 which significantly limits their applications in many fields. Several approaches including surface fixation, and intercalation into transparent clay membranes have been employed to conquer the ACQ effect but with limited success.12,13 In 2001, Tang et al. discovered an aggregation-induced emission (AIE) phenomenon of silole derivatives, which was of great interest to the scientific community.14 AIE is a special photophysical phenomenon of luminescent materials, which overcomes the limitation that ACQ-active materials cannot be used for solid-state luminescence applications. Since then, a lot of AIE-active organic luminophores have been reported.15–18 In 2002, Manimaran et al. found that Re(I) tricarbonyl complexes with pyridine ligands exhibited phosphorescence enhancement by rigidification, which was the first example of metal-based luminophores with aggregation induced phosphorescent emission (AIPE).19 The first in-depth investigation on the AIPE mechanism of cyclometalated Ir(III) complexes was reported by Li and co-workers in 2008, and they found that the aggregation phosphorescence enhancement was mainly dependent on the structures of ancillary ligands in the complexes.20 The first example of an AIPE-active cationic iridium complex was reported by Su, Liao and co-workers in 2011. These AIPE-active compounds, with carbazole units at the 4 and 7 positions of the phenanthroline ancillary ligands, feature a dendrimer-like structure.21 Consequently, a number of AIPE-active Ir(III) compounds with different molecular structures were developed in the past few years.22–32
Cyclometalated Ir(III) complexes are particularly suitable for use as oxygen-sensitive probes (OSPs) in luminescence oxygen sensing, due to their outstanding optical properties. In 1996, Marco and co-workers33 reported the first use of an Ir(III) complex as the active species in a luminescent oxygen sensor, which attracted much attention and lots of Ir(III) complexes as OSPs have been reported successively thereafter.34–37 We have a long-term interest in disclosing the relationship between the molecular structures of the cyclometalated metal complexes and their oxygen sensing properties. A series of platinum(II) and iridium(III) complexes as efficient OSPs have been synthesized and investigated.5,37–42 In this paper, a new diphenylamino (DPA)-substituted cationic bis-cyclometalated Ir(III) complex with AIPE properties was synthesized, which was used to explore the effects of the DPA group on the photophysical and electrochemical properties of the corresponding Ir(III) complex. In addition, the oxygen sensitivity and operational stability of the Ir(III) complex were studied.
2. Results and discussion
2.1 Synthesis and characterization of the complexes
Chemical structures and the detailed synthetic protocols of the Ir(III) complexes are shown in Scheme 1. Cyclometalating ligands L1 and L2 were prepared efficiently via a palladium-catalyzed ligand-free and aerobic Suzuki–Miyaura reaction in aqueous ethanol developed by our group.43 Both of the Ir(III) complexes were synthesized via a three-step route: cyclometalated Ir(III) m-chloro-bridged dimers were first obtained, followed by treatment with 1,10-phenanthroline, and then a counterion exchange reaction from Cl− to PF6−. The corresponding characterization data for the Ir(III) complexes are presented in the Experimental section.
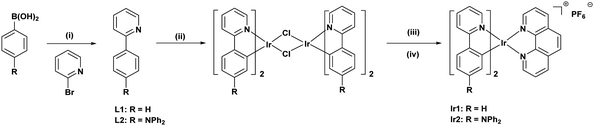 |
| Scheme 1 Synthesis of cyclometalated Ir(III) complexes. (i) Pd(OAc)2, K2CO3, EtOH/H2O 3 : 1 (v/v), 80 °C in air, 30–60 min. (ii) IrCl3·3H2O, EtOCH2CH2OH/H2O, 3 : 1 (v/v), 110 °C, N2, 24 h. (iii) 1,10-Phenanthroline, EtOCH2CH2OH, 120 °C, N2, 24 h. (iv) KPF6, RT, 3 h. | |
2.2 Photophysical properties
UV-vis absorption spectra and emission spectra of Ir1 and Ir2 in CH2Cl2 (1.0 × 10−5 M) at room temperature are presented in Fig. 1 and the related data are listed in Table 1. Similar to the previously reported cyclometalated Ir(III) complexes, both complexes exhibit strong absorption bands in the ultraviolet region belonging to the spin-allowed intraligand (1π–π*) transitions.44,45 The long wavelength with lower extinction coefficient absorption can be assigned to spin-allowed and spin-forbidden metal-to-ligand charge-transfer transitions (1MLCT and 3MLCT).46,47Fig. 1(a) shows that DPA-substituted Ir2 has a larger molar absorption coefficient than that of Ir1.
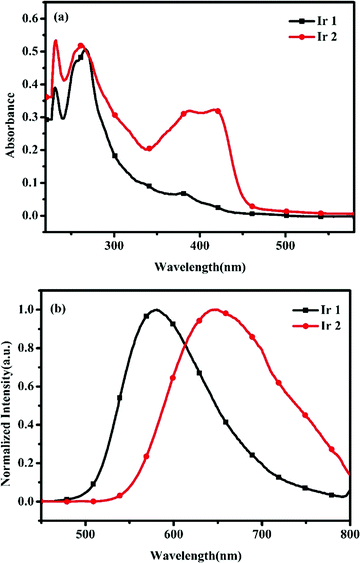 |
| Fig. 1 Absorption (a) and emission spectra (b) of the Ir(III) complexes (1.0 × 10−5 M in CH2Cl2) at room temperature. | |
Table 1 Photophysical data of Ir(III) complexes Ir1 and Ir2
Complex |
λ
abs
(nm) |
λ
em
(nm) |
Φ
PL
(ΦPL)d |
τ
(μs) |
k
r
(105 s−1) |
k
nr
(105 s−1) |
Measured in CH2Cl2 at a concentration of 10−5 mol L−1 and extinction coefficients (104 M−1 cm−1) are shown in parentheses.
The emission maxima are the values in bold style (λexc = 410 nm).
The quantum yields (Φsolution) in deoxygenated CH2Cl2 were measured with [Ir(ppy)2(acac)] (ΦPL = 0.34) as a standard.
The absolute phosphorescence quantum yields determined by employing an integrating sphere.
In deoxygenated CH2Cl2 solution.
The radiative and nonradiative decay rates of kr and knr were calculated from kr = ΦPL × τ−1 and knr = τ−1 − kr. All results were measured at ambient temperature.
|
Ir1
|
230(3.89), 266(5.07), 389(0.66), 413(0.32) |
579(CH2Cl2) |
23.0% (12.5%) |
0.80 |
2.9 |
9.6 |
536(EC film) |
Ir2
|
232(5.35), 262(5.20), 385(3.17), 419(3.23) |
645(CH2Cl2) |
0.65% (6.1%) |
0.13 |
0.5 |
76.4 |
559(EC film) |
The normalized emission spectra of these complexes are shown in Fig. 1(b). The emission maxima are red-shifted 66 nm for Ir2 compared to Ir1, owing to the electron-donating ability of the DPA moiety. These results show that the emission of the complexes could be finely tuned by the modification of the structures of the cyclometalating ligands.
The phosphorescence lifetimes (τ) of Ir1 and Ir2 in CH2Cl2 are 0.80 μs and 0.13 μs at room temperature, respectively (Table 1, the phosphorescence decay profiles of the Ir(III) complexes are provided in Fig. S2, ESI†). However, the τ value for the complex with a DPA group (Ir2) shortens significantly compared with that of Ir1, which may result from large nonradiative decay caused by the rotation of the DPA group in Ir2 (see Table 1). Thus, the substituent effect of the cyclometalating ligands plays an important role in tuning the photoluminescence properties of the Ir(III) complexes.
2.3 Aggregation-induced emission properties
The emission spectra and photoluminescence (PL) quantum yields (ΦPL) of complexes Ir1 and Ir2 in different states were measured and the ΦPL data are summarized in Table S1 (ESI†). Upon illumination under a 365 nm UV lamp, complex Ir1 exhibits intense emission bands in acetonitrile at room temperature, while the dilute acetonitrile solution of Ir2 is almost non-emissive, which is consistent with a low value of ΦPL, as shown in Fig. 2. In sharp contrast, bright orange lights were observed in the solid state, indicating that Ir2 probably is AIPE-active (Fig. 2(b)).
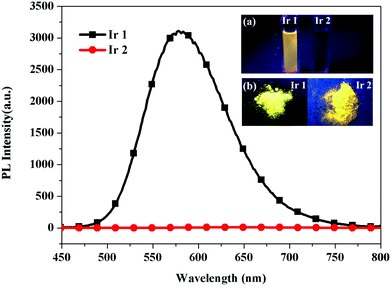 |
| Fig. 2 Emission spectra of the Ir(III) complexes (5.0 × 10−5 M in CH3CN) at room temperature. Insets: Photographs of Ir1 and Ir2 in solution and the solid state taken under a 365 nm lamp. | |
In order to further confirm the AIPE characteristics of Ir2, the emission spectra of complex Ir2 were investigated with different water fractions in a H2O/CH3CN system. As shown in Fig. 3, almost no photoluminescence signal was recorded for Ir2 in acetonitrile, whereas the intensity of emission varied dramatically with the variation of the proportion of water in the mixed solvent. Upon adding water up to 60 vol%, the intensity of emission was increased obviously. The phenomena could be explained by the restriction of intramolecular rotation.15,16 When Ir2 is molecularly dissolved in a good solvent (acetonitrile), it is a weak emitter due to the very high non-radiative decay rate (76.4 × 105 s−1, Table 1), caused by the active twisting motions of the DPA group. In contrast, when large amounts of water were added to its solution, the formation of aggregates restricts the intramolecular rotation and results in a strong phosphorescent emission. The maximum PL intensity was reached with the water content up to 70%. As depicted in Fig. 3(b), the PL intensity at the water fraction (fw) of 70% is almost 300 times higher than that in acetonitrile. And similarly the value of ΦPL at the water fraction (fw) of 70% is almost 17 times higher than that in acetonitrile (Fig. S3, ESI†). However, a clear declining in trend was observed after that. There are two possible reasons for the decrease in emission intensity when water is increased to a certain fraction in the solvent system.48 The first one is that, when bigger aggregates are formed, only the molecules on the surface could be irradiated, most of them were limited internally, resulting in a weak emission intensity. Secondly, crystal particles and amorphous particles could be formed when a large amount of water is added. The former ones would enhance the emission intensity but the latter do not.
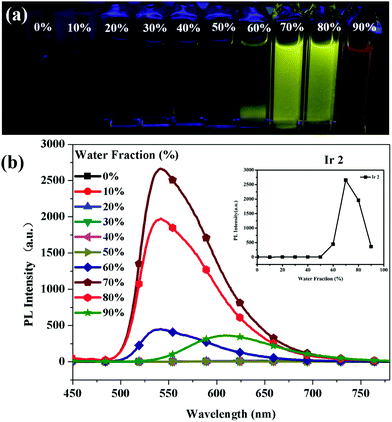 |
| Fig. 3 Emission spectra and photographs of Ir(III) complex Ir2 (5.0 × 10−5 mol L−1) in H2O/CH3CN with different water fractions (0–90%) at room temperature. | |
2.4 Electrochemical properties
The electrochemical properties of the Ir(III) complexes were studied via cyclic voltammetry (CV) and the results are listed in Table 2. During the anodic scan at the rate of 100 mV s−1, the CVs of Ir1 and Ir2 showed oxidation potentials of +1.18 V and +0.70 V versus the saturated calomel electrode (SCE), respectively. This positive oxidative wave is assigned to the metal-centered Ir(III)/Ir(IV) oxidation couple, in accordance with the reported cyclometalated Ir(III) systems.49 Obvious differences in the oxidative potentials were observed between non-DPA substituted Ir1 and DPA-modified Ir2. As shown in Table 2, it is found that introducing the DPA group in the benzene unit leads to a low potential for Ir2. These complexes exhibit a reduction wave at different potentials of −1.31 V and −1.16 V, which can be assigned to the reduction of the phenanthroline ligands. The HOMO and LUMO energies can be calculated using the following equations (EHOMO (eV) = −e(4.4 + Eonsetox), ELUMO (eV) = −e(4.4 + Eonsetred)) and the results are presented in Table 2.
Table 2 Electrochemical data for Ir1 and Ir2
Complex |
E
onsetox a [V] |
E
onsetred a [V] |
E
HOMO
[eV] |
E
LUMO
[eV] |
E
g
[eV] |
0.1 M [Bu4N]PF6 in CH2Cl2, at a scan rate of 100 mV s−1, measured using the saturated calomel electrode (SCE) as the standard.
E
HOMO (eV) = −e(4.4 + Eonsetox).
E
LUMO (eV) = −e(4.4 + Eonsetred).
E
g
= E
LUMO − EHOMO. HOMOs and LUMOs denote highest-occupied molecular orbitals and lowest-unoccupied molecular orbitals, respectively.
|
Ir1
|
1.18 |
−1.31 |
−5.58 |
−3.09 |
2.49 |
Ir2
|
0.70 |
−1.16 |
−5.10 |
−3.24 |
1.86 |
2.5 Theoretical calculations
In order to understand the impacts of the substituent (DPA) on the properties of the Ir(III) complexes and estimate the energy levels and electron density distributions of the orbitals of Ir1 and Ir2, Density Functional Theory (DFT) calculations were performed. As reported in Fig. 4, the HOMO distribution primarily resides on the iridium center and cyclometalating ligands and the LUMO distribution is localized on the whole 1,10-phenanthroline ligand. The HOMO–LUMO gaps calculated for Ir1 and Ir2 are 3.21 eV and 2.65 eV, respectively. The results clearly show that the HOMO–LUMO gaps calculated for Ir1 and Ir2 are different to each other, revealing that the HOMO and LUMO levels are strongly influenced by the DPA group in the 4-position of the benzene ring, resulting in a decrease in Eg from 3.21 eV for Ir1 to 2.65 eV for Ir2. These results reasonably explain the red-shifts observed in the emission maxima (see Fig. 1(b)).
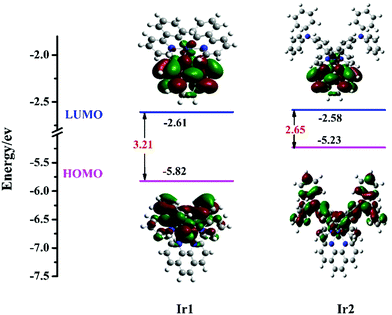 |
| Fig. 4 Calculated energy-level diagram and contour plots of the HOMO and LUMO for the Ir(III) complexes. | |
To investigate the nature of the emissive excited state, the low lying triplet states of these Ir(III) complexes were calculated based on their optimized geometry of the ground state (S0) using the time-dependent approach (TD-DFT). The vertical excitation energies and molecular orbitals involved in the excitations for the lowest energy triplet states are summarized in Table 3 (see Fig. S5 in the ESI† for the electron density maps of the frontier molecular orbitals that are mainly involved in the lowest lying transition). TD-DFT calculations show that the emitting excited state of complexes Ir1 and Ir2 involves much more 3MLCT and 3LLCT character and different degrees of 3LC character, which can explain their broad unstructured emission bands as shown in Fig. 1(b). Compared with Ir2, non-DPA substituted Ir1 has the predominant 3LC nature of the T2 state.
Table 3 Calculated triplet states of Ir1 and Ir2 by using a TD-DFT approach
Complex |
State |
E
(eV) |
Compositionb |
CIc |
Character |
Only the selected low-lying excited states are presented.
Only the main configurations are presented. H and L denote HOMOs and LUMOs, respectively.
The configuration-interaction (CI) coefficients are absolute values. Oscillator strengths are zero because of the neglect of spin–orbit coupling in the TD-DFT calculations.
|
Ir1
|
T1 |
2.50 |
H → L |
0.6958 |
MLCT/LLCT |
T2 |
2.75 |
H → L+1 |
0.6058 |
MLCT/LLCT |
|
|
H → L+3 |
0.2719 |
LC |
Ir2
|
T1 |
2.20 |
H → L |
0.6867 |
MLCT/LLCT |
T2 |
2.26 |
H−1 → L |
0.6932 |
MLCT/LLCT |
T3 |
2.36 |
H−1 → L+2 |
0.3635 |
MLCT/LLCT/LC |
|
H → L+1 |
0.4430 |
MLCT/LLCT |
|
H → L+3 |
0.3529 |
MLCT/LLCT |
2.6 Oxygen sensing properties
The oxygen sensitivity of the Ir(III) complexes was investigated in CH2Cl2 first. As expected, the emission intensity of the complexes decreased with the increase of the oxygen concentration from 0 to 100%. For example, changing from an N2 atmosphere to 2.17% O2 (mixed with N2, v/v) will significantly quench the emission intensity of complex Ir1 by over 50%. In addition, the shape of the emission profile without distortions under different oxygen concentrations guarantees that the decreased phosphorescence can be attributed only to the oxygen quenching process. A similar quenching effect was observed for complex Ir2 as shown in Fig. 5.
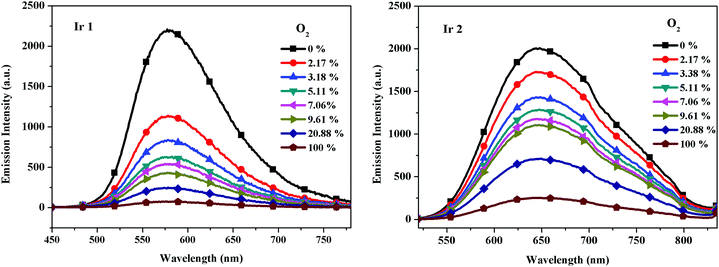 |
| Fig. 5 Emission spectra of Ir1 and Ir2 (1.0 × 10−5 M in CH2Cl2) under different oxygen concentrations at room temperature. | |
In fluid solution, the relationship between the emission intensity of the OSP and the concentration of quencher (O2) is reflected by the Stern–Volmer equation, which can be described as eqn (1), where I is the emission intensity, subscript 0 denotes the value of the quantity in the absence of a quencher, KSV is the Stern–Volmer quenching constant and Po2 is the partial pressure of oxygen.
| 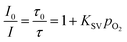 | (1) |
The I0/I100 value is used to evaluate the O2 sensing properties of phosphorescent OSPs, where I0 and I100 represent the detected luminescence intensities in the 100% N2 and 100% O2 atmosphere. The I0/I100 values of these Ir(III) complexes in CH2Cl2 are 29.2 and 7.9, respectively. Stern–Volmer plots are shown in Fig. S6 (ESI†). The results show that AIPE-active Ir2 is not an efficient OSP in CH2Cl2.
To obtain an oxygen sensor for continuous monitoring of molecular oxygen, the oxygen sensitive probe is usually incorporated in a thin layer of matrix polymer ethyl cellulose (EC). This is because EC is easily penetrated by oxygen with a permeability coefficient P of 1.1 × 10−12 cm2 Pa s−1 and has an excellent processability in common organic solvents.50 It is regarded as a standard host material with good long-term stability and commercial availability for oxygen sensing. In this work the oxygen sensitivity of the Ir(III) complexes was also studied in EC films. According to eqn (2), the quenching behaviors of Ir1 and Ir2 were analyzed at various oxygen concentrations. For a heterogeneous oxygen sensing film, a modified Stern–Volmer plot is usually required to quantify the quenching effect. A two-site model was proposed by Demas and co-workers,51,52 which has been generally accepted and widely used ever since.53,54 In the two-site model, the OSP takes into account (at least) two distinctly different environments, one (f1) being quenchable, the other (f2) either not being quenched at all, or being quenched at a very different rate (f1 + f2 = 1). Each component shows different quenching constants (KSV1 and KSV2).
| 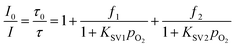 | (2) |
where
I0 and
I are the emission intensities of a probe in the absence and presence of oxygen, respectively.
PO2 is the partial pressure of oxygen. The weighted quenching constant
KappSV (
KappSV =
KSV1·
f1 +
KSV2·
f2) is a guide of the sensitivity of an oxygen sensor, and higher values indicate that the sensor is more sensitive to oxygen.
55 The Stern–Volmer plots for oxygen sensing films of these Ir(
III) complexes immobilized in EC are shown in
Fig. 6. The results in
Table 4 reveal that the
I0/
I100 values of EC film sensors immobilized with
Ir1 and
Ir2 are 3.2 and 8.2, respectively. The
KappSV values of the Ir(
III) complexes immobilized in the EC film are 0.00346 (
Ir1) and 0.01346 (
Ir2). It is clear that complex
Ir2 with a DPA group exhibits higher oxygen sensitivity compared to that of
Ir1. These results are just opposite from those in the solution. When immobilized in an EC film,
Ir2 demonstrates the aggregation-induced phosphorescent emission (AIPE) due to the restriction of intramolecular motion of the DPA moiety. This is the reason why
Ir2 exhibits much stronger oxygen sensitivity in the EC film than that in CH
2Cl
2. Therefore, the DPA group is crucial to enhance the oxygen sensitivity of the corresponding complex in an EC film.
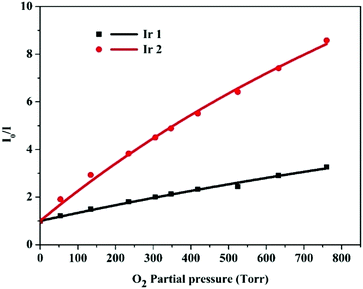 |
| Fig. 6 Stern–Volmer plots for oxygen sensing films of the Ir(III) complexes immobilized in EC (intensity ratios I0/I versus O2 partial pressure). | |
Table 4 Parameters for the O2-sensing films of Ir(III) complexes Ir1 and Ir2 with EC as the supporting matrix (fitting of the result to the two-site model)
Complexes |
I
0/I100a |
f
1
|
f
2
|
K
SV1
|
K
SV2
|
r
2 d |
K
appSV e |
I
0 and I100 represent the detected emission intensities in the 100% N2 and 100% O2 atmosphere, respectively.
Ratio of the two portions of the Ir(III) complexes.
Quenching constant of the two portions.
Determination coefficients.
Weighted quenching constant, KappSV = f1KSV1 + f2KSV2.
|
Ir1
|
3.2 |
0.93220 |
0.06780 |
0.00371 |
0.0001 |
0.99182 |
0.00346 |
Ir2
|
8.2 |
0.96047 |
0.03953 |
0.01401 |
0.0001 |
0.98866 |
0.01346 |
2.7 Operational stability of oxygen sensing films
Reversibility and stability are important factors to influence the overall performance of a sensor.56 Therefore, the operational stability tests of oxygen sensing films were conducted. The emission intensity is monitored when the oxygen sensing film is exposed to an atmosphere which periodically varies between 100% N2 and 100% O2 within 4000 s. Both the oxygen sensing films demonstrated a stable optical signal during the quenching and recovering cycles (Fig. 7 and Fig. S8 in the ESI†), suggesting excellent operational stability. In addition, fast response and recovery times were obtained. Both of the sensing films demonstrate quick response times (<4.6 s when changing from 0 to 100 vol% O2) and recovery times (<12.2 s when changing from 100 to 0 vol% O2), respectively. Thus the oxygen sensing films of these cationic Ir(III) complexes show complete reversibility with short response times. DPA-modified cyclometalated Ir(III) complex Ir2 with excellent oxygen sensing properties is a potential candidate for online continuous monitoring of oxygen concentrations.
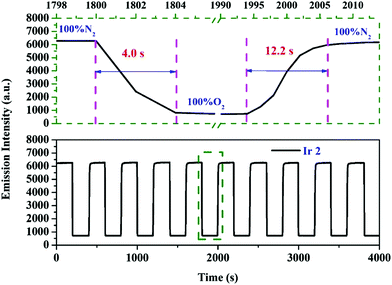 |
| Fig. 7 Reversibility and emission intensity response of the sensing film of Ir2 immobilized in EC when cycling from 100% N2 to 100% O2. | |
3. Conclusions
In summary, two cationic cyclometalated Ir(III) complexes Ir1 and Ir2 have been synthesized and their photophysical and electrochemical properties were studied in detail. The results demonstrate that the DPA group is an attractive substituent for tuning the properties of the corresponding Ir(III) complexes. The emission band of Ir2 with a DPA group is red-shifted 66 nm compared to Ir1. DPA-modified Ir(III) complex Ir2 exhibits obvious aggregation-induced phosphorescent emission, while non-DPA-substituted Ir1 is AIPE-inactive. In addition, the introduction of a DPA moiety affects remarkably the oxygen sensitivity of the Ir(III) complex in an organic solvent and in an EC film. Immobilized in ethyl cellulose, Ir2 demonstrates high oxygen sensitivity at a KappSV value of 0.01346 Torr−1. These findings might bring a useful perspective in the development of new phosphorescent materials with high performances.
4. Experimental
4.1 Materials and instruments
All starting materials were purchased from commercial suppliers and used without further purification. The solvents were treated as required prior to use. 1H NMR and 13C NMR spectra were recorded on a 400 MHz Varian Unity Inova spectrophotometer. Mass spectra were recorded using a MALDI micro MX spectrometer. UV/Vis absorption spectra were recorded on an HP8453 UV/Vis spectrophotometer. Emission spectra were recorded using an F-7000 spectrofluorimeter. Photoluminescence quantum yields were measured relative to [Ir(ppy)2(acac)] (ΦP = 0.34 in CH2Cl2, under degassed conditions). Phosphorescence lifetimes were measured on an Edinburgh FLS920 Spectrometer. Cyclic voltammograms of the Ir(III) complexes were recorded on an electrochemical workstation (BAS100B/W, USA) at room temperature in a 0.1 M [Bu4N]PF6 solution under argon conditions. Phosphorescence intensity responses of sensing films of the Ir(III) complexes were recorded using an F-7000 spectrofluorimeter. DFT calculations were carried out by using B3LYP. The LanL2DZ basis set was employed for the iridium atom and the 6-31G* basis set was used to treat all other atoms. All these calculations were performed using the Gaussian 09 software package.
4.2 Synthesis of cyclometalated Ir(III) complexes
Synthesis of C^N ligands.
The C^N ligands were synthesized by the palladium-catalyzed Suzuki–Miyaura cross-coupling reactions.43
Synthesis of the Ir(III) complexes.
Both Ir(III) complexes [Ir(C^N)2(phen)]PF6 were prepared using the same procedure according to literature methods.38 IrCl3·3H2O was reacted with 2.5 equiv. cyclometalating C^N ligand in a mixture of 2-ethoxyethanol and water (9 mL/3 mL) at 110 °C under nitrogen for 24 h to afford a cyclometalated iridium bridged-chloride dimer. Without further purification the dimer was mixed with 3.0 equiv. 1,10-phenanthroline in 2-ethoxyethanol at 120 °C under nitrogen for 24 h. After cooling down to room temperature, a 10-fold excess of KPF6 was added. The suspension was stirred for 3 h and washed with water and dried over Na2SO4. The crude product was applied to a silica gel column and eluted with CH2Cl2 to afford the desired Ir(III) complexes.
Conflicts of interest
The authors declare no conflict of interest.
Acknowledgements
The authors gratefully acknowledge the financial support from the National Natural Science Foundation of China (21421005, 21276043, and U1603103) and the Talent Fund of Shandong Collaborative Innovation Center of Eco-Chemical Engineering (XTCXYX02). We thank Prof. Jianzhang Zhao at DUT for his assistance with the theoretical calculations.
Notes and references
- F. D. Angelis, S. Fantacci, N. Evans, C. Klein, S. M. Zakeeruddin, M. Jacques-E, K. Kuppuswamy, H. J. Bolink, M. Gretzel and M. K. Nazeeruddin, Inorg. Chem., 2007, 46, 5989 CrossRef PubMed.
- B. Liu, F. Dang, Z. Feng, Z. Tian, J. Zhao, W. Yong, X. Yang, G. Zhou, Z. Wu and W. Wong, J. Mater. Chem. C, 2017, 5, 7871 RSC.
- Q. Zhao, C. Huang and F. Li, Chem. Soc. Rev., 2011, 40, 2508 RSC.
- Y. Liu, P. Zhang, X. Fang, G. Wu, S. Chen, Z. Zhang, H. Gao, W. Tang and L. Xu, Dalton Trans., 2017, 46, 4777 RSC.
- C. Liu, X. Lv, Y. Xing and J. Qiu, J. Mater. Chem. C, 2015, 3, 8010 RSC.
- R. D. Costa, E. Ortí, H. J. Bolink, F. Monti, G. Accorsi and N. Armaroli, Angew. Chem., Int. Ed., 2012, 51, 8178 CrossRef CAS.
- J. Liu, Y. Liu, Q. Liu, C. Li, L. Sun and F. Li, J. Am. Chem. Soc., 2011, 133, 15276 CrossRef CAS PubMed.
- L. Yao, J. Zhou, J. Liu, W. Feng and F. Li, Adv. Funct. Mater., 2012, 22, 2667 CrossRef CAS.
- P. Duan, N. Yanai and N. Kimizuka, Chem. Commun., 2014, 50, 13111 RSC.
- X. Li, M. Boris, H. Ågen and H. Tian, J. Phys. Chem. C, 2011, 115, 20724 CrossRef CAS.
-
J. B. Birks, Photophysics of Aromatic Molecules, Wiley-Interscience, London, 1970 Search PubMed.
- J. Wang, Y. Zhao, C. Dou, H. Sun, P. Xu, K. Ye, J. Zhang, S. Jiang, F. Li and Y. Wang, J. Phys. Chem. B, 2007, 111, 5082 CrossRef CAS PubMed.
- Y. Ishida, T. Shimada and S. Takagi, J. Phys. Chem. C, 2014, 118, 20466 CrossRef CAS.
- J. Luo, Z. Xie, J. W. Lam, L. Cheng, H. Chen, C. Qiu, H. S. Kwok, X. Zhan, Y. Liu, D. Zhu and B. Tang, Chem. Commun., 2001, 1740 RSC.
- Y. Hong, J. W. Lam and B. Tang, Chem. Commun., 2009, 4332 RSC.
- J. Mei, N. L. Leung, R. T. Kwok, J. W. Lam and B. Tang, Chem. Rev., 2015, 115, 11718 CrossRef CAS PubMed.
- M. Gao and B. Tang, Drug Discovery Today, 2017, 22, 1288 CrossRef CAS PubMed.
- J. Tavakoli, H. Zhang, B. Tang and Y. Tang, Mater. Chem. Front., 2019, 3, 664 RSC.
- B. Manimaran, P. Thanasekaran, T. Rajendran, R. Lin, I. Chang, G. Lee, S. Peng, S. Rajagopal and K. Lu, Inorg. Chem., 2002, 41, 5323 CrossRef CAS PubMed.
- Q. Zhao, L. Li, F. Li, M. Yu, Z. Liu, T. Yi and C. Huang, Chem. Commun., 2008, 685 RSC.
- G. Shan, D. Zhu, H. Li, P. Li, Z. Su and Y. Liao, Dalton Trans., 2011, 40, 2947 RSC.
- D. Chao and Y. Zhang, Sens. Actuators, B, 2017, 245, 599 CrossRef CAS.
- T. Gao, J. Zhang, R. Yan, D. Cao, D. Jiang and D. Ye, Inorg. Chem., 2018, 57, 4310 CrossRef CAS PubMed.
- W. Che, G. Li, X. Liu, K. Shao, D. Zhu, Z. Su and M. R. Bryce, Chem. Commun., 2018, 54, 1730 RSC.
- R. Liu, Z. Song, Y. Li, Y. Li, W. Yao, H. Sun and H. Zhu, Sens. Actuators, B, 2018, 259, 840 CrossRef CAS.
- Z. Song, R. Liu, H. Zhu, Y. Lu, X. Li and H. Zhu, Sens. Actuators, B, 2019, 279, 385 CrossRef CAS.
- V. Sathish, A. Ramdass, P. Thanasekaran, K.-L. Lu and S. Rajagopal, J. Photochem. Photobiol., C, 2015, 23, 25 CrossRef CAS.
- L. Ravotto and P. Ceroni, Coord. Chem. Rev., 2017, 346, 62 CrossRef CAS.
- Y. Wang, T. Yang, X. Liu, G. Li, W. Che, D. Zhu and Z. Su, J. Mater. Chem. C, 2018, 6, 12217 RSC.
- F. Ren, P. Liu, Y. Gao, J. Shi, B. Tong, Z. Cai and Y. Dong, Mater. Chem. Front., 2019, 3, 57 RSC.
- D. Li, G. Li, W. Che, D. Zhu and Z. Su, Dalton Trans., 2019, 48, 1955 RSC.
- L. Zhang, Y. Li, W. Che, D. Zhu, G. Li, Z. Xie, N. Song, S. Liu, B. Tang, X. Liu, Z. Su and M. R. Bryce, Adv. Sci., 2019, 6, 1802050 CrossRef PubMed.
- G. D. Marco, M. Lanza, M. Pieruccini and S. Campagna, Adv. Mater., 1996, 8, 576 CrossRef.
- K. Koren, S. M. Borisov, R. Saf and I. Klimant, Eur. J. Inorg. Chem., 2011, 1531 CrossRef CAS PubMed.
- E. M. Boreham, L. Jones, A. N. Swinburne, M. Blanchard-Desce, V. Hugues, C. Terryn, F. Miomandre, G. Lemercier and L. S. Natrajan, Dalton Trans., 2015, 44, 16127 RSC.
- D. E. Achatz, R. J. Meier, L. H. Fischer and O. S. Wolfbeis, Angew. Chem., Int. Ed., 2011, 50, 260 CrossRef CAS PubMed.
- C. Liu, H. Yu, X. Rao, X. Lv, Z. Jin and J. Qiu, Dyes Pigm., 2017, 136, 641 CrossRef CAS.
- H. Yu, C. Liu, Z. Yu, L. Zhang and J. Xiu, J. Mater. Chem. C, 2017, 5, 3519 RSC.
- Y. Xing, C. Liu, X. Song and J. Li, J. Mater. Chem. C, 2015, 3, 2166 RSC.
- Y. Xing, C. Liu, J. Xiu and J. Li, Inorg. Chem., 2015, 54, 7783 CrossRef CAS PubMed.
- H. Yu, C. Liu, X. Lv, J. Xiu and J. Zhao, Dyes Pigm., 2017, 145, 136 CrossRef CAS.
- C. Liu, X. Song, X. Rao, Y. Xing, Z. Wang, J. Zhao and J. Qiu, Dyes Pigm., 2014, 101, 85 CrossRef CAS.
- C. Liu, X. Rao, X. Song, J. Qiu and Z. Jin, RSC Adv., 2013, 3, 526 RSC.
- Y. Zhou, H. Gao, X. Wang and H. Qi, Inorg. Chem., 2015, 54, 1446 CrossRef CAS PubMed.
- W. Lee, T.-H. Kwon, J. Kwon, J.-Y. Kim, C. Lee and J.-I. Hong, New J. Chem., 2011, 35, 2557 RSC.
- R. Wang, L. Deng, T. Zhang and J. Li, Dalton Trans., 2012, 41, 6833 RSC.
- C. L. Ho, C. S. Lam, N. Sun, D. Ma, L. Liu, Z. Yu, L. Xue, Z. Lin, H. Li, Y. H. Lo and W. Y. Wong, Isr. J. Chem., 2014, 54, 999 CrossRef CAS.
- Z. Song, R. Liu, Y. Li, H. Shi, J. Hu, X. Cai and H. Zhu, J. Mater. Chem. C, 2016, 4, 2553 RSC.
- C. L. Ho, W. Y. Wong, G. Zhou, B. Yao, Z. Xie and L. Wang, Adv. Funct. Mater., 2007, 17, 2925 CrossRef CAS.
- D. E. Achatz, R. J. Meier, L. H. Fischer and O. S. Wolfbeis, Angew. Chem., Int. Ed., 2011, 50, 260 CrossRef CAS PubMed.
- E. R. Carraway, J. N. Demas, B. A. Degraff and J. R. Bacon, Anal. Chem., 1991, 63, 337 CrossRef CAS.
- J. N. Demas, B. A. Degraff, W. Xu and A. Chem, Anal. Chem., 1995, 67, 1377 CrossRef CAS.
- W. Wu, S. Ji, W. Wu, H. Guo, X. Wang, J. Zhao and Z. Wang, Sens. Actuators, B, 2010, 149, 395 CrossRef CAS.
- Y. Xiong, Z. Ye, J. Xu, Y. Zhu, C. Chen and Y. Guan, Analyst, 2013, 138, 1819 RSC.
- X. Guo, Y. Liu, Q. Chen, D. Zhao and Y. Ma, Adv. Opt. Mater., 2018, 6, 1700981 CrossRef.
- W. S. Lee, K. Y. Wong, X. Li, Y. B. Leung, C. S. Chan and K. S. Chan, J. Mater. Chem., 1993, 3, 1031 RSC.
Footnote |
† Electronic supplementary information (ESI) available. See DOI: 10.1039/c9qm00227h |
|
This journal is © the Partner Organisations 2019 |