DOI:
10.1039/C9QM00216B
(Research Article)
Mater. Chem. Front., 2019,
3, 1335-1340
Redox-responsive fluorescent AIE bioconjugate with aggregation enhanced retention features for targeted imaging reinforcement and selective suppression of cancer cells†
Received
4th April 2019
, Accepted 29th April 2019
First published on 29th April 2019
Abstract
Considering the distinctions of cancer cell microenvironments, smart systems with stimulus-triggered features have become increasingly popular nowadays. These smart systems are regarded as efficient approaches for targeted cancer cell imaging and selective tumoricidal therapy. On account of the highly reductive micro-environment in tumor tissues, a redox-responsive fluorescent AIE bioprobe based on a disulfide bond is designed and synthesized. This AIE bioprobe possesses excellent water dispersibility and nano-size stability under physiological conditions. Under the reductive microenvironment of tumor cells, the water dispersibility of the AIE probe becomes worse owing to the cleavage of carboxyl groups. The dispersibility alteration further induces the aggregation of AIE nanoparticles and enhances intracellular retention, allowing for targeted imaging reinforcement and selective suppression of cancer cells.
Introduction
Cancer, the greatest threat to human health, causes millions of deaths worldwide and the incidence of cancer is continuously increasing.1 Tremendous endeavors have been devoted to developing effective techniques and anticancer drugs for diagnosis and treatment.2–5 Early diagnosis and therapy of cancer before its metastasis are of great significance for its cure, while early diagnosis is difficult to carry out due to the small size of the tumor. Fluorescent bioprobes can realize real-time monitoring with high resolution, which endows them with unique superiorities in the diagnosis of cancer at an early stage. Thus, many fluorophores have been designed for the early-stage diagnosis of cancer.6
The development of fluorescence bioprobes, such as fluorescent polymers,7,8 inorganic nanoparticles,9 organic dyes,10–12 and quantum dots (QDs),13 which provide alternatives for cell imaging and tracing, has recently attracted a great deal of research attention. These fluorescence bioprobes have significant applications in the monitoring and visualization of biological features, and the diagnosis and tracking of many diseases in living systems. Among these approaches, fluorescence bioprobes with the aggregation-induced emission characteristic (AIEgens) exhibit prominent performances, such as adjustable emission brightness via regulating the aggregation form, high resistance to photobleaching and easy modification of specific groups.14–16 These AIEgens could serve as superior agents for biological process monitoring17–21 and disease theranostics.22–25 Particularly, designing intelligent AIE bioprobes that can respond to stimuli in tumour microenvironments opens new avenues to the advancement of cancer imaging and therapy, providing comprehensive insight into pathogenesis mechanisms and a precise guide in theranostics.26–29
As for the stimuli in tumour microenvironments, reductive-responsiveness has been investigated in controlled drug delivery for better cancer targeting and treatment.30–33 This is based on the fact that the microenvironment in tumour tissues is more reductive than in the normal tissues, with about a 4-fold elevated concentration of glutathione (g-glutamyl-cysteinyl-glycine, GSH), which could cleave the disulfide bond through the thiol–disulfide exchange reaction.31,34 Moreover, an alteration of intracellular GSH level is closely tied with many health problems such as cancer, cardiovascular diseases, diabetes, neurological disorder and ageing.30,35 Enthusiastic research on GSH-triggered drug delivery systems has inspired scientists to explore efficient GSH-responsive AIE bioprobes that are capable of targeted cancer cell imaging, selective cellular tracing and monitoring intricate biological processes.36,37 However, their GSH-responsiveness is realized via the cleavage of the link between AIE molecules and a hydrophilic polymer chain. And the cleaved AIE small molecules are likely to leak, which largely restricts their biomedical applications.38
Water dispersibility, size stability and leakage-free properties are vital elements for the biocompatibility and tracing capability of AIE bioprobes.38–40 Conjugating AIE molecules onto hydrophilic polymers to self-assemble into nanoparticles (NPs) is a prominent approach to improve their water dispersibility retention ability. NPs with a diameter between 30 and 200 nm can be easily internalized through endocytosis, and they have been widely designed to realize various inspiring functions in vivo, especially for the diagnosis and treatment of cancer.41–43 Furthermore, cellular uptake and enhanced retention of small NPs in tumor sites could be achieved by stimulated NP aggregation in certain microenvironments. To date, a few studies have focused on the stimulus-triggered intracellular retention enhancement effect and subsequent aggregation induced emission reinforcement of AIE NP bioprobes.44,45
This contribution reports a rational design and synthesis of redox-responsive AIE bioconjugate NPs based on the disulfide bond for targeted imaging reinforcement and selective suppression of cancer cells. The NP bioprobe (TPE-CS-ss-COOH, shortened as TCSC, see Fig. 1) consists of a chitosan (CS) polymer chain attached with a large number of tetraphenylethylene (TPE) units and disulfide-linked carboxyl groups. In 10 μM DTT solution, which mimics physiological conditions, TCSC exhibits excellent water dispersibility, nano-size stability and dispersive fluorescent emission. Upon exposure to 10 mM DTT solution, which mimics a tumor reductive microenvironment,46,47 the disulfide bonds of the NPs are cleaved via the thiol–disulfide exchange reaction. Consequent detachment of the carboxyl groups induces worse water dispersibility and causes further aggregation of TPE-CS. The augmented aggregation would not only enhance the intracellular retention of NPs, but also elevate the emission brightness and contrast to some extent. Furthermore, the GSH-triggered aggregation-enhanced retention effect makes cellular tracing and monitoring the biological behaviors of cancer cells feasible. It is found that the TCSC NP bioprobes could also selectively suppress the propagation of cancer cells. To the best of our knowledge, this is the first report on the synthesis and application of GSH-triggered AIE fluorescent reinforcement and retention enhancement NP bioprobes, which will inspire more exciting research in the fields of AIE bioimaging and precise cancer theranostics.
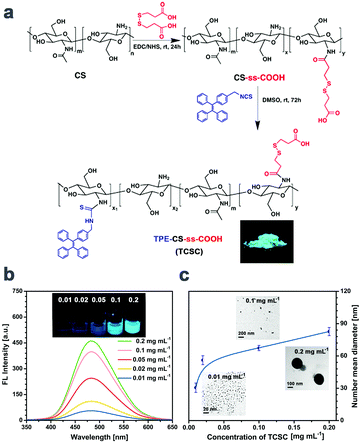 |
| Fig. 1 Molecular design and AIE properties of TCSC. (a) Synthetic route of the NP probes of TCSC. Inset: TCSC solid powder under UV illumination; (b) fluorescence spectrum of the TCSC aqueous solution at different concentrations. Insets: Photographs of TCSC solutions excited by UV light; (c) hydrodynamic diameter of TCSC aqueous solution at different concentrations. Insets: TEM images of the corresponding concentrations. | |
Experimental section
Materials and instruments
All the chemicals and reagents were purchased from Aldrich and Sinopharm Chemical Reagent Co., Ltd and used without further purification, unless specified otherwise. Chitosan (Mη = 2.52 × 104) was supplied by the Qingdao Haihui Bioengineering Co., Ltd (Qingdao, China) with 83.5% degree of deacetylation. TPE-ITC was synthesized according to our previous research.21 DMEM containing 10% fetal bovine serum and antibiotics (100 units mL−1 penicillin and 100 μg mL−1 streptomycin) was purchased from Geno Biopharmaceutical Technology Co., Ltd (Hangzhou, China). MitoTracker® Red, FITC Annexin V and Propidium Iodide (PI) were obtained from Invitrogen Corp. The synthesis, apparatus, cytotoxicity and live cell imaging procedures are described in the ESI.†
Results and discussion
Molecular design and AIE properties
The disulfide-linked carboxyl chitosan (CS-ss-COOH) was first synthesized via amidation between the terminal carboxyl groups of dithiodipropionic acid and amino groups of CS. TPE with isothiocyanate groups (TPE-ITC) was then conjugated with CS-ss-COOH to afford TCSC (Fig. 1a). The detailed synthetic routes and characterization (1H NMR spectrum) are shown in Fig. 1a and Fig. S1 in the ESI.† It was calculated from the 1H NMR spectrum that approximately 22 ss-COOH units are conjugated to one CS chain on average and there are roughly 11 TPE groups on one CS chain on average. The solid powder of TCSC emits a strong greenish-blue light (inset picture in Fig. 1a) when excited by UV light. The high fluorescent emission in the solid state confirms its AIE characteristics, which differ from the traditional aggregation caused quenching (ACQ) of fluorescent dyes.
The fluorescence (FL) intensity of TCSC solutions with different ethanol (poor solvent)/H2O (good solvent) ratios was utilized to further verify the AIE effect of the TCSC bioconjugate (Fig. S2, ESI†). It is obvious that the FL intensity (emission around 480 nm) increases with the increase of the poor solvent content. In addition, FL intensity and the corresponding diameter of the TCSC aqueous solutions with different concentrations (0.01, 0.02, 0.05, 0.1, and 0.2 mg mL−1) were measured by using FL spectroscopy, Dynamic light scattering (DLS) and TEM (Fig. 1). The DLS and TEM results suggest that the hydrodynamic diameters of the TCSC NPs are of nano-size in aqueous media (30–100 nm) and TCSC NPs appear to possess excellent water dispersibility and nano-size stability (Fig. S3 and S4b, ESI†). The mechanism of TCSC NP formation in the aqueous solution can be ascribed to the self-assembly of TCSC polymer chains, with hydrophobic fluorophore TPE as the interior core and the exposed hydrophilic carboxyl groups as the exterior layers. With an increase in the concentration (0.01 to 0.2 mg mL−1) and degree of labelling (2.16 mol% to 8.63 mol%), TCSC NPs exhibit augmented luminance and a slightly red-shifted emission peak, indicating tighter packing and restricted intramolecular rotation of TPE aggregates in the core (Fig. 1c and Fig. S4a, ESI†).48
Redox-induced aggregation of TCSC bioprobes
To verify that TCSC can switch the distribution form of the NPs in response to a highly reductive microenvironment, the FL spectrum and the hydrodynamic diameter changes of the NP bioprobes over time in 10 mM DTT were investigated and their transition tendencies are presented in Fig. 2. As shown in Fig. 2a and b, upon incubation of TCSC NPs in 10 mM DTT, the FL intensity of the mixture increases gradually as time elapses. Correspondingly, the hydrodynamic diameters of the TCSC bioprobes exhibit a positive correlation with incubation time (from 40 nm at 0 h to 200 nm at 36 h, and several μm at 60 h, see Fig. 2c and d). These results manifest the occurrence of the redox-responsive cleavage of carboxyl groups on TCSC in the reductive microenvironment.
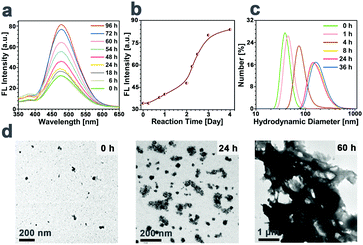 |
| Fig. 2 Redox-responsive properties of TCSC bioprobes. (a) FL spectrum of TCSC after different incubation periods in 10 mM DTT; (b) FL intensity changes of TCSC bioprobes incubated in 10 mM DTT over time; (c) hydrodynamic diameter distribution changes of TCSC after different reaction times in 10 mM DTT; (d) TEM images of the corresponding nanoparticles after different incubation periods. | |
Redox-induced imaging reinforcement and selective suppression of cancer cells
Considering the goal of targeted cellular tracing and biomedical applications of TCSC NPs, it is noteworthy to explore the cytotoxicity of the NP bioprobes against both cancer cells and normal cells. MTT cell viability assays were conducted after 1 d, 3 d, and 5 d incubation of HeLa, HepG2, MCF-7 and FIB with the TCSC NP bioprobes. As shown in Fig. S5 (ESI†), TCSC at a concentration of 0.1 mg mL−1 shows relatively higher cytotoxicity to cancer cells (HeLa, HepG2 and MCF-7, with a higher GSH content) than normal FIB cells, especially for relatively long-term cellular retention at 5 days. These results reveal that the difference in the reductive microenvironments of cancer and normal cells largely affects the cytotoxicity of the TCSC NP bioprobes. The highly reductive microenvironment of cancer cells can induce the aggregation and size augmentation of TCSC NPs, further causing the suppression of cancer cells.49,50
On account of this fact, it is reasonable to hypothesize that the cleavage of carboxyl groups on TCSC in a reductive intracellular microenvironment can not only reinforce its aggregation and FL intensity in cancer cells, but also enhance the intracellular retention of the NPs owing to the augmentation of particle size (Scheme 1). The GSH-triggered augmentation of particle size contributes to the long-term retention and selective suppression of cancer cells. The internalized TCSC NPs function as supramolecular aggregates, and they do not undergo exocytosis once internalized, accounting for their remarkable leakage-free staining behaviors.24 Consequently, the TCSC NPs could hardly be excreted via exocytosis and they maintained their aggregated form in the reductive microenvironment, leading to the suppression of cancer cells.
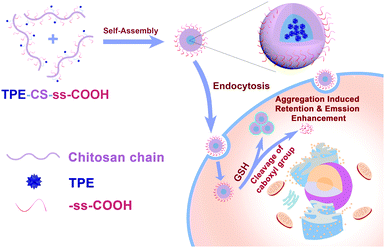 |
| Scheme 1 Schematic illustration of TPE-CS-ss-COOH as a redox-responsive NP bioprobe for GSH-triggered aggregation enhanced retention and targeted imaging reinforcement of cancer cells. | |
In order to authenticate this hypothesis, the utilization of TCSC NP bioprobes for targeted cancer cell imaging was conducted using confocal laser scanning microscopy (CLSM). After incubation with TCSC (0.1 mg mL−1) in the culture medium for 24 h at 37 °C, HeLa, HepG2, MCF-7 and FIB cells were observed, respectively. After a 24 h incubation period, TCSC NPs are internalized into the cells and appear to locate in the cytoplasm region. Merely weak fluorescence can be observed inside the FIB normal cells, while the cancer cells (HeLa, HepG2, and MCF-7) present apparently stronger FL emission than that of the normal cells (Fig. 3). Besides, the statistical results for average FL intensity per cell (Fig. S6, ESI†) corroborate the hypothesis that the TCSC NP bioprobes can serve as a redox-responsive AIE bioprobe for targeted cancer cell imaging reinforcement.
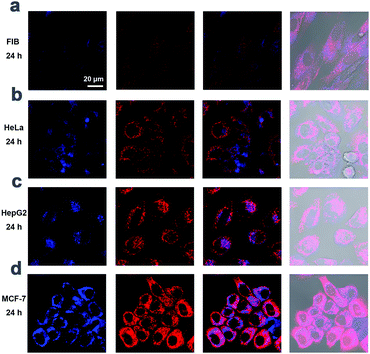 |
| Fig. 3 Confocal laser scanning microscopy (CLSM) images of different cells stained by 0.1 mg mL−1 TCSC for 24 h, and then stained by MitoTracker® Red CMSRos for TCSC NP localization. (a) FIB; (b) HeLa; (c) HepG2; (d) MCF-7. First column: TCSC particles (blue); second column: mitochondria (red); third column: merge images of two fluorescence channels; and fourth column: with corresponding bright field channel images, respectively. | |
Mechanism of redox-induced aggregation enhanced retention (AER) effect
For the purpose of figuring out the mechanism for the different cytotoxicity and imaging effect of TCSC on cancer cells and normal cells, cellular tracing and corresponding apoptosis measurements were carried out on FIB and HeLa for comparison. The living HeLa cells stained by TCSC emit intensively for 5 generations (Fig. 4a), while much weaker emission is noticed for the stained FIB living cells (Fig. 4b). Additionally, there exist different staining regions for the two cells, shown as a more dispersed distribution of TCSC NP bioprobes within the FIB cells, and evident clustered regions of TCSC NP bioprobes appearing inside the HeLa cells. For the purpose of confirming that the higher FL intensity of HeLa cells is unrelated to the probable higher TCSC NP intake caused by cellular activity differences, different cellular seeding densities were applied to HeLa cells and FIB cells to realize a lower TCSC uptake for the HeLa cells. It is noteworthy that the HeLa cells present a relatively higher FL intensity with a comparatively lower TCSC NP intake compared to the FIB cells (Fig. 4c). This phenomenon can be interpreted as follows: the highly reductive microenvironment inside HeLa triggers the cleavage of the hydrophilic shells of the TCSC NPs, further inducing core aggregation and emission reinforcement of the AIE bioprobes. Tangible proof is provided by some especially bright dots and clusters emerging in the HeLa cells (Fig. 4a). Besides, these especially bright dots and clusters tend to appear regularly inside round cells, which is commonly regarded as a sign of apoptosis. With the extension of the staining time, there is clearly a descending tendency in the quantity of cells and an ascending probability for the appearance of round cells with especially bright dots, demonstrating the suppression of HeLa cells.
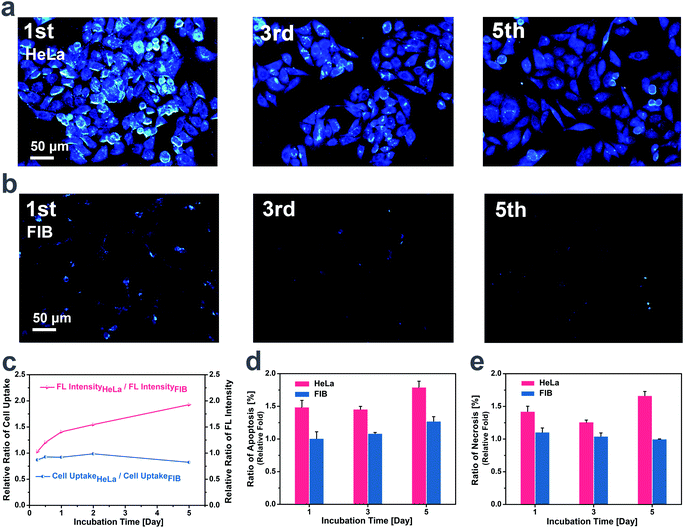 |
| Fig. 4 Mechanism of redox-induced aggregation enhanced retention (AER) effect. Cellular tracing of (a) HeLa cancer cells and (b) FIB normal cells by 0.1 mg mL−1 TCSC at different passages; (c) relative ratio of cell uptake amount for HeLa and FIB (Cell UptakeHeLa/Cell UptakeFIB, blue line) and relative ratio of FL intensity for HeLa and FIB (FL IntensityHeLa/FL IntensityFIB, red line) at different incubation times determined by using a UV standard curve and fluorescence spectrum, respectively; (d) ratio of apoptosis and (e) necrosis for HeLa and FIB relative to the blank control at different incubation times determined by using flow cytometry. | |
The results of the apoptosis measurements determined by flow cytometry indicate that the uptake of TCSC NPs induces the apoptosis of HeLa cells, which further leads to their relatively high ratio of apoptosis and necrosis during long time incubation (Fig. 4d and e). Although there exists an understandable inconsistency in cell viability value owing to the methodological difference, the results and tendency of the flow cytometry assays are in high accordance with the cytotoxicity trend of MTT assays mentioned before. TCSC NPs could suppress the propagation and increase the apoptosis/necrosis ratio of HeLa cells. The redox-induced aggregation and size augmentation of the TCSC NPs can be regarded as the specific cause of the TCSC NP inhibition activity on cancer cells. It can be elucidated that the cleaving of carboxyl residues in cancer cells results in the ulterior aggregation of the TCSC NPs. The enhanced TCSC NP aggregation further induces the retention enhancement (AER) and emission reinforcement of the AIE bioprobes, and the long-term retention and accumulation of large particles finally contribute to the cytotoxicity toward and apoptosis of cancer cells.
Conclusion
In summary, a new design strategy for AIE bioprobes that can respond to a tumor reductive microenvironment is reported. The NP bioprobe possesses excellent water dispersibility, nano-size stability, and dispersive fluorescent emission under physiological conditions. In the highly reductive microenvironment with 10 mM DTT, the NP bioprobe sacrifices its dispersity as a result of carboxyl group detachment, inducing the further aggregation of TCSC NPs and augmentation in both particle size and luminous intensity. In vitro experiments demonstrate that there exist significant distinctions between normal cells and cancer cells after incubation with TCSC, in terms of fluorescence intensity, staining regions, retention time and cytotoxicity. Flow cytometry measurements verify that the aggregated TCSC NPs trigger the apoptosis of HeLa cells and contribute to their necrosis. All these results suggest that the TCSC NP bioprobes can be utilized for targeted cancer cell imaging reinforcement and selective suppression of cancer cells, making them superior agents for biological process monitoring and cancer theranostics. However, there still exists some intrinsic weakness for blue-emitting fluorescent systems, especially due to their restriction in vivo imaging applications. This work just explores the tip of the iceberg. Development of a red-emitting AIE bioconjugate with stimulus-triggered aggregation enhanced retention features would be promising for cancer diagnosis and therapy in vivo.
Conflicts of interest
The authors declare no competing financial interests.
Acknowledgements
This work was financially supported by the National Natural Science Foundation of China (No. 51873187), the National Key Research and Development Program of China (No. 2017YFE0117700 and 2018YFC1004803) and 2018 Zhejiang University Academic Award for Outstanding Doctoral Candidates (No. 2018082).
Notes and references
- D. Hanahan and R. A. Weinberg, Cell, 2011, 144, 646–674 CrossRef CAS PubMed.
- S. S. Gambhir, Nat. Rev. Cancer, 2002, 2, 683–693 CrossRef CAS PubMed.
- D. J. Brenner and E. J. Hall, N. Engl. J. Med., 2007, 357, 2277–2284 CrossRef CAS PubMed.
- M. D. Fox and M. E. Raichle, Nat. Rev. Neurosci., 2007, 8, 700–711 CrossRef CAS PubMed.
- J. E. Kennedy, Nat. Rev. Cancer, 2005, 5, 321–327 CrossRef CAS PubMed.
- J. Mei, N. L. C. Leung, R. T. K. Kwok, J. W. Y. Lam and B. Z. Tang, Chem. Rev., 2015, 115, 11718–11940 CrossRef CAS PubMed.
- K. Wang, X. Zhang, X. Zhang, C. Ma, Z. Li, Z. Huang, Q. Zhang and Y. Wei, Polym. Chem., 2015, 6, 4455–4461 RSC.
- S. R. Meech, Chem. Soc. Rev., 2009, 38, 2922–2934 RSC.
- K. Zhou, Y. Zhang, Z. Xia and W. Wei, Nanotechnology, 2016, 27, 275101 CrossRef PubMed.
- J. Niu, J. Fan, X. Wang, Y. Xiao, X. Xie, X. Jiao, C. Sung and B. Tang, Anal. Chem., 2017, 89, 7210–7215 CrossRef CAS PubMed.
- J. Shi, Q. Deng, C. Wan, M. Zheng, F. Huang and B. Tang, Chem. Sci., 2017, 8, 6188–6195 RSC.
- J. Shi, Q. Deng, Y. Li, M. Zheng, Z. Chai, C. Wan, Z. Zheng, L. Li, F. Huang and B. Tang, Anal. Chem., 2018, 90, 13775–13782 CrossRef CAS PubMed.
- X. Michalet, F. F. Pinaud, L. A. Bentolila, J. M. Tsay, S. Doose, J. J. Li, G. Sundaresan, A. M. Wu, S. S. Gambhir and S. Weiss, Science, 2005, 307, 538–544 CrossRef CAS PubMed.
- J. D. Luo, Z. L. Xie, J. W. Y. Lam, L. Cheng, H. Y. Chen, C. F. Qiu, H. S. Kwok, X. W. Zhan, Y. Q. Liu, D. B. Zhu and B. Z. Tang, Chem. Commun., 2001, 1740–1741 RSC.
- M. Wang, G. X. Zhang, D. Q. Zhang, D. B. Zhu and B. Tang, J. Mater. Chem., 2010, 20, 1858–1867 RSC.
- Y. Hong, J. W. Y. Lam and B. Z. Tang, Chem. Soc. Rev., 2011, 40, 5361–5388 RSC.
- G. Feng, Y. Yuan, H. Fang, R. Zhang, B. Xing, G. Zhang, D. Zhang and B. Liu, Chem. Commun., 2015, 51, 12490–12493 RSC.
- Y. Yuan, R. T. K. Kwok, G. Feng, J. Liang, J. Geng, B. Z. Tang and B. Liu, Chem. Commun., 2014, 50, 295–297 RSC.
- M. Gao, C. K. Sim, C. W. T. Leung, Q. Hu, G. Feng, F. Xu, B. Z. Tang and B. Liu, Chem. Commun., 2014, 50, 8312–8315 RSC.
- J. Chen, M. Gao, L. Wang, S. Li, J. He, A. Qin, L. Ren, Y. Wang and B. Z. Tang, ACS Appl. Mater. Interfaces, 2018, 10, 11436–11442 CrossRef CAS PubMed.
- Z. Wang, S. Chen, J. W. Lam, W. Qin, R. T. Kwok, N. Xie, Q. Hu and B. Z. Tang, J. Am. Chem. Soc., 2013, 135, 8238–8245 CrossRef CAS PubMed.
- X. Gao, G. Feng, P. N. Manghnani, F. Hu, N. Jiang, J. Liu, B. Liu, J. Z. Sun and B. Z. Tang, Chem. Commun., 2017, 53, 1653–1656 RSC.
- K. Han, S. B. Wang, Q. Lei, J. Y. Zhu and X. Z. Zhang, ACS Nano, 2015, 9, 10268–10277 CrossRef CAS PubMed.
- Y. Yu, C. Feng, Y. Hong, J. Liu, S. Chen, K. M. Ng, K. Q. Luo and B. Z. Tang, Adv. Mater., 2011, 23, 3298–3302 CrossRef CAS PubMed.
- S. Chen, H. Wang, Y. Hong and B. Z. Tang, Mater. Horiz., 2016, 3, 283–293 RSC.
- Y. Cheng, C. Sun, X. Ou, B. Liu, X. Lou and F. Xia, Chem. Sci., 2017, 8, 4571–4578 RSC.
- X. Shi, C. Y. Y. Yu, H. Su, R. T. K. Kwok, M. Jiang, Z. He, J. W. Y. Lam and B. Z. Tang, Chem. Sci., 2017, 8, 7014–7024 RSC.
- D. Ding, R. T. K. Kwok, Y. Yuan, G. Feng, B. Z. Tang and B. Liu, Mater. Horiz., 2015, 2, 100–105 RSC.
- Y. Yuan, S. Xu, C.-J. Zhang, R. Zhang and B. Liu, J. Mater. Chem. B, 2016, 4, 169–176 RSC.
- F. Meng, W. E. Hennink and Z. Zhong, Biomaterials, 2009, 30, 2180–2198 CrossRef CAS PubMed.
- L. Jia, D. Cui, J. Bignon, A. Di Cicco, J. Wdzieczak-Bakala, J. Liu and M. H. Li, Biomacromolecules, 2014, 15, 2206–2217 CrossRef CAS PubMed.
- P. Zhang, H. Zhang, W. He, D. Zhao, A. Song and Y. Luan, Biomacromolecules, 2016, 17, 1621–1632 CrossRef CAS PubMed.
- W. Li, P. Zhang, K. Zheng, Q. Hu and Y. Wang, J. Mater. Chem. B, 2013, 1, 6418 RSC.
- L. Jia, Z. Li, D. Zhang, Q. Zhang, J. Shen, H. Guo, X. Tian, G. Liu, D. Zheng and L. Qi, Polym. Chem., 2013, 4, 156–165 RSC.
- D. M. Townsend, K. D. Tew and H. Tapiero, Biomed. Pharmacother., 2003, 57, 145–155 CrossRef CAS.
- W. Cheng, G. Wang, X. Pan, Y. Zhang, B. Z. Tang and Y. Liu, Macromol. Biosci., 2014, 14, 1059–1066 CrossRef CAS PubMed.
- Y. Wang, M. Z. Lv, N. Song, Z. J. Liu, C. Wang and Y. W. Yang, Macromolecules, 2017, 50, 5759–5766 CrossRef CAS.
- Z. K. Wang, L. Yang, Y. L. Liu, X. F. Huang, F. H. Qiao, W. Qin, Q. L. Hu and B. Z. Tang, J. Mater. Chem. B, 2017, 5, 4981–4987 RSC.
- Z. Long, M. Liu, R. Jiang, Q. Wan, L. Mao, Y. Wan, F. Deng, X. Zhang and Y. Wei, Chem. Eng. J., 2017, 308, 527–534 CrossRef CAS.
- X. Zhang, X. Zhang, B. Yang, M. Liu, W. Liu, Y. Chen and Y. Wei, Polym. Chem., 2013, 4, 4317 RSC.
- X. Liu, Y. Chen, H. Li, N. Huang, Q. Jin, K. Ren and J. Ji, ACS Nano, 2013, 7, 6244–6257 CrossRef CAS PubMed.
- R. Pan, G. Liu, Y. Li, Y. Wei, S. Li and L. Tao, Nanoscale, 2018, 10, 8269–8274 RSC.
- J. Zhang, X. Zheng, X. Hu and Z. Xie, J. Mater. Chem. B, 2017, 5, 4470–4477 RSC.
- N. Li, H. Yang, W. Pan, W. Diao and B. Tang, Chem. Commun., 2014, 50, 7473–7476 RSC.
- Z. Wang, B. Xu, L. Zhang, J. Zhang, T. Ma, J. Zhang, X. Fu and W. Tian, Nanoscale, 2013, 5, 2065–2072 RSC.
- L. Jia, D. Cui, J. Bignon, A. Di Cicco, J. Wdzieczak-Bakala, J. Liu and M.-H. Li, Biomacromolecules, 2014, 15, 2206–2217 CrossRef CAS PubMed.
- P. Zhang, H. Zhang, W. He, D. Zhao, A. Song and Y. Luan, Biomacromolecules, 2016, 17, 1621–1632 CrossRef CAS PubMed.
- W. C. Wu, C. Y. Chen, Y. Tian, S. H. Jang, Y. Hong, Y. Liu, R. Hu, B. Z. Tang, Y. T. Lee, C. T. Chen, W. C. Chen and A. K. Y. Jen, Adv. Funct. Mater., 2010, 20, 1413–1423 CrossRef CAS.
- S. Chen, C. Zhang, G. Jia, J. Duan, S. Wang and J. Zhang, Mater. Sci. Eng., C, 2014, 43, 330–342 CrossRef CAS PubMed.
- Q. Sun, T. Ishii, K. Kanehira, T. Sato and A. Taniguchi, Biomater. Sci., 2017, 5, 1014–1021 RSC.
Footnote |
† Electronic supplementary information (ESI) available: Synthesis procedures, 1H NMR spectra, fluorescence spectra, size stability, AIE behaviour, cell viability, flow cytometric analysis, etc. See DOI: 10.1039/c9qm00216b |
|
This journal is © the Partner Organisations 2019 |