DOI:
10.1039/C8QM00458G
(Research Article)
Mater. Chem. Front., 2019,
3, 265-275
A facile metal–phenolic–amine strategy for dual-functionalization of blood-contacting devices with antibacterial and anticoagulant properties†
Received
11th September 2018
, Accepted 11th December 2018
First published on 12th December 2018
Abstract
Thrombosis and infections of extracorporeal circuits and indwelling medical devices are the two major life-threatening complications faced in clinical practice. Herein, we report a novel and facile metal–phenolic–amine surface modification strategy to engineer a multifunctional coating on these devices to combat thrombosis and infection. This strategy is inspired by the metal–catecholamine coordination complex of [Fe(dopa)3] in mussels, in which Cu(II) ions (metal), plant polyphenol gallic acid (phenol) and cystamine (amine) are employed to fabricate a copper–phenolic–amine network. Our in vitro and in vivo experiments reveal that the resultant Cu(II)-chelating coatings endow the modified tubing with not only durable antibacterial properties, but also capability to persistently generate anticoagulant therapeutic nitric oxide (NO) gas in the presence of endogenous S-nitrosothiols (RSNO) from fresh blood. We anticipate that our simple and multifunctional coating strategy will be a milestone in the development of surface engineering, especially that of biomedical devices.
1. Introduction
Thrombosis and infections, the two major clinical complications of indwelling medical devices and extracorporeal circuits, often lead to device failure, patient morbidity, mortality, and increased healthcare costs.1–5 Co-administration of anticoagulant drugs (e.g., heparin) and antibiotics is a common strategy to reduce thrombosis and infections in clinical practice. However, this poses the risk of major bleeding and may lead to the development of life-threatening heparin-induced thrombocytopenia (HIT). Application of antibiotics can induce bacterial resistance.6–8 Moreover, administration of antibiotics may lead to fever, thrombophlebitis and epidermal necrolysis, which are unfavorable and much worse than infection itself.9,10 Therefore, new therapeutic approaches to combat thrombosis as well as infections are highly sought after.
Surface modification strategies, including poly(ethylene glycol) (PEG) coating,11 zwitterionic polymer grafting,12 hyaluronic acid modification,13 superhydrophobic14 and bioinspired omniphobic surface treatment15 have been shown to improve the antibacterial and anticoagulant properties of blood-contacting devices. Current coating technologies generally consist of tedious complicated processes including surface pretreatment of materials, chemical specificity between the material surface and the interfacial modifiers, and complex multistep procedures for anchoring bioactive molecules to the coating. Thus, the development of a simple, efficient and versatile strategy to decorate extracorporeal circuits and indwelling medical devices with durable antithrombogenic and anti-infectious functions is clinically indispensable.
Metal–phenolic surface chemistry inspired by the [Fe(dopa)3] complexes to crosslink the protein mfp-1 in the byssus (Fig. 1A)16 is a promising surface coating strategy.17 For example, metal–phenolic network (MPN) coatings like FeIII–tannic acid (TA) (FeIII–TA) coatings have been used as nanocoatings on many types of substrates like polystyrene (PS), poly(lactic-co-glycolic acid) (PLGA), melamine formaldehyde (MF), polydimethylsiloxane (PDMS), SiO2 and Au.17,18 However, in saline solutions, cations (particularly K+) are easily adsorbed onto the substrate surfaces, forming a tightly bound hydration layer at the solid–liquid interface, weakening the binding of the phenols to the surfaces,19,20 and resulting in a failure of the MPN coating. As amine groups can help to remove the hydrated salt layer from the surface, supporting the catechol binding, adaptive synergy between the catechol and the amine (namely, catecholamine chemistry) has also been proposed for the engineering of surface coatings.21
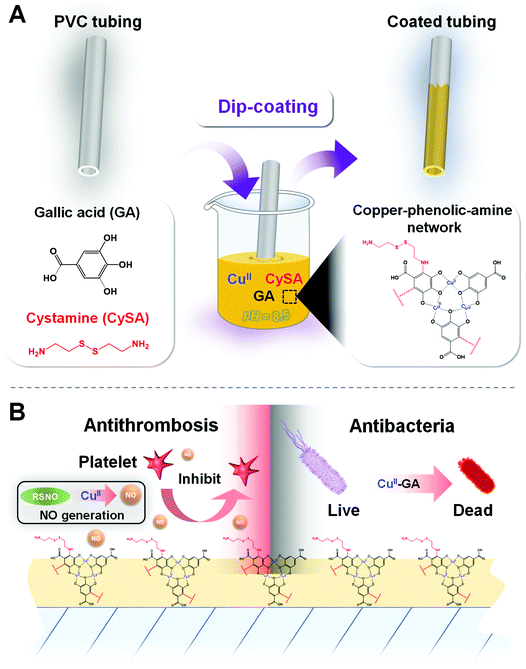 |
| Fig. 1 Scheme of the preparation of the dual-function CuII–GA/CySA coating. (A) The strategy to fabricate the coating on PVC tubing by dip-coating via metal–phenolic–amine surface chemistry in an alkaline mixture solution (pH 8.5) containing CuII, GA and CySA. (B) Such surface coating with a copper–phenolic–amine network can confer the PVC tubing with dual long-term antithrombotic and antibacterial properties. In this coating, Cu2+ with GPx-like catalytic activity is able to achieve antithrombotic properties by generating anticoagulant therapeutic NO gas from RSNOs. On the other hand, GA and Cu2+ in the copper–phenolic–amine network synergistically inhibit bacterial growth. | |
Herein, inspired by metal–phenolic and catecholamine surface chemistry, we developed a biomimetic metal (CuII)–phenolic (plant polyphenol gallic acid)–amine (cystamine) surface chemistry strategy to fabricate an advanced dual-functional coating by mussel-inspired assembly of molecules and ions in a single step. In our design, the copper ions (CuII) are responsible for both the antibacterial22–24 and antithrombotic properties25 as they exhibit glutathione peroxidase (GPx)-like activity to produce the antithrombotic mediator nitric oxide (NO) from endogenous S-nitrosothiols (RSNO) in blood. The herbal polyphenol gallic acid (GA) is selected due to its antibacterial, antiviral, and antiinflammatory properties (Fig. 1B). In addition, cystamine (CySA) is chosen to fabricate the metal–phenolic–amine-based coatings through phenolic–amine (GA–CySA) molecularly assembled crosslinking and metal ion–polyphenol (CuII–GA) coordination bonding in the synergistic processes (Fig. 1). After a simple dip-coating process, the robust chelation of a minute amount of CuII in the metal–phenolic–amine network endows coated polyvinyl chloride (PVC) tubing with durable and significant antibacterial and antithrombogenic properties. We envision that our simple and multifunctional copper–phenolic–amine coating strategy will find wide application in surface engineering of clinically used indwelling blood-contacting devices.
2. Experimental section
Preparation of the CuII–GA/CySA coatings
To prepare CuII–GA/CySA coatings, GA (0.5 mg mL−1) and CySA (0.6 mg mL−1) were dissolved in 10 mM Tris–HCl buffer (pH 8.5). Then, 0, 10, 20, 40 and 60 μg mL−1 feed concentrations of CuCl2·2H2O were added, and planar PVC substrates were immersed in each of the mixed solutions. After 12 hours of immersion, the planar PVC substrates were ultrasonically cleaned with distilled water and dried with N2. The CuII–GA/CySA-coated PVC substrates were named CuII(0), CuII(10), CuII(20), CuII(40) and CuII(60) according to the feed concentrations of CuCl2·2H2O in the reaction system.
Characterization of the CuII–GA/CySA coatings
The surface morphology and roughness of the coatings were analyzed by atomic force microscope (AFM, Bruker, Germany) in tapping mode.
As PVC is too rough to determine the coating thickness, silicon was used instead of PVC for the deposition of the CuII–GA/CySA coating. The thickness of the CuII–GA/CySA coating was measured with a spectroscopic ellipsometer (M-2000V, J. A. Woollam, USA). Wavelengths from 370 to 1000 nm were used to measure the Δ and Ψ values, and a Cauchy model was applied to determine the coating thickness.
We used a Krüss GmbH DSA 100 Mk 2 goniometer (Hamburg, Germany) to measure the water contact angle (WCA) of the CuII–GA/CySA coating at room temperature. A 5 μL of test liquid drop was used for image processing with DSA 1.8 software. A minimum of six values of WCA were obtained for each sample.
To analyze the chemical structure of the CuII–GA/CySA coatings, the CuII–GA/CySA coating was deposited on an Au substrate, and the grazing incidence attenuated total reflection Fourier transform infrared (GATR-FTIR) spectrum was determined using a Nicolet model 5700 instrument.
An X-ray photoelectron spectroscopy (XPS) instrument (XSAM200, Kratos Ltd, UK), supplied with a monochromatic A1 Kα source (1486.6 eV) and operated at 12 kV × 15 mA and a pressure of 20 MPa, was utilized to analyze the surface elemental composition of the CuII–GA/CySA coatings. With the use of 300 eV pass energy, overview XPS spectra were taken between 50 and 1300 eV at 0.5 eV energy steps. Detailed spectra of peaks of interest were taken with a 0.05 eV energy step. Each sample had 15 min of total acquisiton time.
The measurement of electron paramagnetic resonance (EPR) was performed by a Bruker A320 equipped with a Microwave Bridge to analyze the chelation of CuII to GA, in the CuII–GA/CySA coatings.
A MALDI micro MX time-of-flight mass spectrometer (Waters, Milford, MA), equipped with a 337 nm N2 laser operated with 4 ns-duration pulse, was utilized to perform matrix assisted laser analysis of ionization spectrometry (MALDI-MS) measurements in reflection mode. The detailed operation and analysis were reported elsewhere.26
Measurement of catalytic NO release
NO release induced by CuII–GA/CySA coating was monitored in real-time using a chemiluminescence NO analyzer (NOA) (Seivers 280i, Boulder, CO). CuII–GA/CySA coatings were prepared on PVC substrates (0.5 cm × 1 cm). The catalytically produced NO by the CuII–GA/CySA coated PVC was purged from the test solution of PBS containing NO donor S-nitrosoglutathione (GSNO, one of the most typical RSNO species used for this test) and reducing agent glutathione (GSH), and was transported to the NO analyzer with a stream of N2. The amount of NO induced by different samples was determined according to the calibration curves of the NOA reported in detail elsewhere.27,28 To investigate the long-term catalytic effect of the CuII–GA/CySA coatings, the coated PVC substrates were immersed in NO donor solution at 37 °C, which was replaced every 12 hours. Then, the NO donor treated samples were used for testing catalytic NO generation. Also, XPS measurements were adopted to analyze the changes of the surface chemical compositions of these NO donor-treated samples.
Platelet adhesion and activation
Platelet rich plasma (PRP) was obtained by centrifugation (1500 rpm, 15 min) of fresh human whole blood, acquired from the central blood station of Chengdu, China. The blood was treated with tri-sodium citrate in a volumetric ratio of 9
:
1. Due to the chemical instability of PRP, we added an extra NO donor (10 μM GSNO, 10 μM GSH) to perform a comparison analysis. Then, 1 mL of PRP, with or without NO donor, was added to the surface of each specimen and incubated for 2 hours. During incubation, the same amount of the NO donor was added to the NO donor group every 30 min. Then, the specimens were washed with saline and immersed in 2.5% glutaraldehyde overnight. Following further dehydration, we sputtered gold onto the samples and examined them with SEM (Quanta 200, FEI, Netherlands).29
Hemolysis evaluation
The sample was firstly placed in a 15 mL centrifuge tube with 9.8 mL saline. An equal amount of saline and distilled water without sample were set as negative and positive control, respectively. Then, 2 mL of fresh blood was diluted with 2.5 mL of saline. After that, 200 μL of the diluted blood was added into each tube and placed into an incubator at 37 °C for an hour. Finally, each of the incubated blood samples were centrifuged at 3000 rpm for 5 min. The supernatant was tested with a microplate reader at 540 nm to determine the released hemoglobin. The hemolysis ratio was calculated using the following equation:
R = (AX − ANC)/(APC − ANC) × 100% |
In the equation, AX, ANC and APC are the absorbance of the tested sample, the negative control and the positive control, respectively. All of the tests were performed at least twice.
Cell viability assays
In this work, we determined the biocompatibility of the CuII–GA/CySA coatings using human umbilical vein endothelial cells (HUVECs). We analyzed the HUVEC morphology with actin immunostaining and measured cell proliferation with Cell Counting Kit-8 (CCK-8), after 1 and 3 days of cell culture time. The detailed processes of cell culture are described elsewhere.29
Bactericidal assays
Bactericidal activities of the CuII–GA/CySA coatings were performed in accordance with ISO22196-2011.30 Both Gram-positive Staphylococcus aureus (S. aureus), strain ATCC 6538, and Gram negative Escherichia coli (E. coli), strain ATCC 25922, were chosen for bacteria tests. They were pre-cultured and diluted either 1/500 (E. coli) or 1/100 (S. aureus) in nutrient broth to obtain a test inoculum at a concentration of 6 × 105 bacteria per mL. Planar PVC substrates (25 mm × 25 mm) with or without coating with CuII–GA/CySA were UV-sterilized. Using a pipet, 0.1 mL of the test inoculum was placed onto the substrate surfaces. They were then covered with a piece of 20 mm × 20 mm film (0.05 to 0.10 mm thick, made of polyethylene, polypropylene or poly(ethylene terephthalate)) and incubated at 35 ± 1 °C at a humidity of no less than 90% for 24 hours. The antibacterial rate R was calculated as:
with NC and N representing the numbers of colonies on the control sample and the coated sample, respectively.
Ex vivo blood circulation thrombogenicity test
We followed all ethical guidelines in accordance with the animal use protocol of the China Council on Animal Care and Southwest Jiaotong University. Prior to conducting the experiment, general anesthesia was administered to all experimental animals. Six New Zealand white rabbits (2.5–3.5 kg) were used in this experiment. The left carotid artery and the right jugular vein of the rabbits were isolated and then cannulated and connected with an arteriovenous (AV) extracorporeal circuit (ECC). The ECC was unclamped at both sides to allow blood to flow through for 2 hours. Photographs of the cross sections and residual thrombosis of the tubes were taken to analyze the percent occlusion of the circuits. The specimens then were rinsed with PBS (pH 7.4) and kept in 2.5% glutaraldehyde solution (with PBS) overnight, and then were dehydrated and critical point dried for SEM analysis. The flow rates in the PVC tubing circuits after 2 hours of circulation were further tested.26,31
Statistical analysis
All quantitative results are expressed as mean ± standard deviation (SD). Statistical significance was evaluated by one-way analysis of variance (ANOVA) using SPSS software. Probability values less than 0.05 are considered as statistically significant.
3. Results and discussion
Characterization of the CuII–GA/CySA coatings
To test the feasibility of metal–phenolic surface functionalization, a solution consisting of CuCl2·2H2O (40 μg mL−1), the polyphenol GA (0.5 mg mL−1) and CySA (0.6 mg mL−1) was first employed on the PVC tubing. After 12 hours of dip-coating, the coated tubing exhibited the dark-brown color of polyphenoles (Fig. 2A), indicating the formation of the coatings on the PVC tubing. To fabricate the CuII–GA/CySA coatings with adjustable and controllable content of copper, various feed concentrations of CuCl2·2H2O, ranging from 0 to 60 μg mL−1 were explored, meanwhile GA and CySA kept at a constant concentration of 0.5 mg mL−1 and 0.6 mg mL−1, respectively. The successful formation of surface coatings on PVC (Fig. S1A and B, ESI†) as well as silicon (Fig. 2B) were confirmed by SEM, AFM and ellipsometry, respectively. XPS analysis showed that the atomic concentration of copper was a function of the feed concentration of CuCl2·2H2O (Fig. 2C and D), indicating a robust control of CuII–GA/CySA chemistry at an ion/molecular level for surface engineering of PVC. We also noted that the atomic composition of sulfur of the CuII–GA/CySA coatings decreased with increase of the feeding concentration of CuCl2·2H2O, suggesting the competitive chemistry of metal–phenolic (cooper–GA) coordination over phenolic–amine (GA–CySA) chemical cross-linking (e.g. Michael addition and Schiff-base cross-linking).
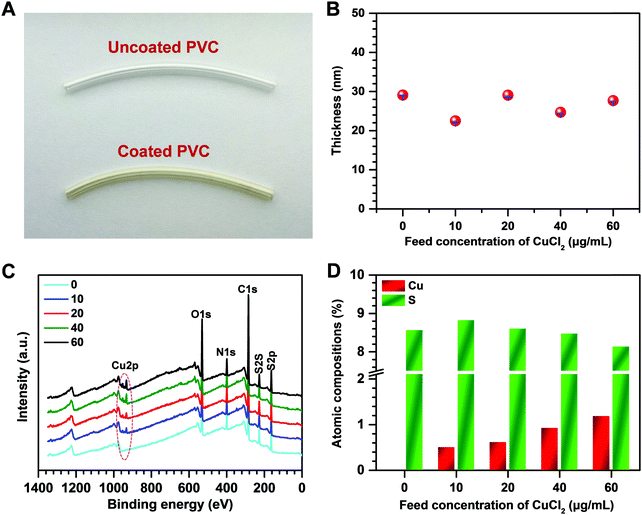 |
| Fig. 2 (A) Photographs of the PVC catheter before and after coating with CuII–GA/CySA. (B) Thickness of the CuII–GA/CySA coating on the silicon surface as a function of CuCl2·2H2O feed concentration. (C) XPS spectra of the CuII–GA/CySA coatings prepared with feed concentrations of CuCl2·2H2O ranging from 0 to 60 μg mL−1. (D) Shift of Cu and S atomic compositions of the CuII–GA/CySA coatings fabricated with different feed concentrations of CuCl2·2H2O. | |
To understand the formation mechanism of the CuII–GA/CySA coatings, surface analysis using GATR-FTIR and high-resolution O1s spectra of XPS were carried out. As shown in Fig. S2 (ESI†), two new peaks assigned to Cu–O stretching were detected at 540 and 619 cm−1, indicating the chelation of the copper ion by the phenolic oxygens of GA. Meanwhile, the peaks at 3280 and 3370 cm−1 assigned to O–H stretching of Ph–OH were largely attenuated. There is a broad peak around 1600 cm−1 in the spectra of the CuII–GA/CySA coatings, indicating the reinforcement of C
N stretching, ascribed to the Schiff-base reaction induced by the primary amine group of the CySA and the GA. The chemistry of the GA–copper ion coordination, Michael addition and Schiff-base formation between the primary amine and the gallol groups were further verified using high-resolution O1s spectra of XPS, EPR and MALDI-MS spectra. The high-resolution O1s spectra revealed that the chemical component of the peak being assigned as O–Cu and COO− at ∼530 eV of the CuII(40) coating significantly increased compared to the CuII(0) coating (Fig. S3, ESI†). The formation of CuII–GA coordination in the CuII(40) coating was confirmed by the CuII–GA signals at ∼3460 mT in the EPR spectra (Fig. 3A) and the tris CuII–GA coordination complex at 569 m/z in MALDI-MS (Fig. 3B). The peak of the [M + H]+ ion at 719 m/z demonstrates the role of CySA in cross-linking the tris CuII–GA coordination complex. We also found that the peaks of [M + H]+ ions at 302, 320 and 489 m/z were clearly present in the MS spectrum of the CuII(40) coating (Fig. 3B), confirming the crosslinking reactions between the CySA and GA based on Schiff-base and Michael addition reactions (Fig. 3C).
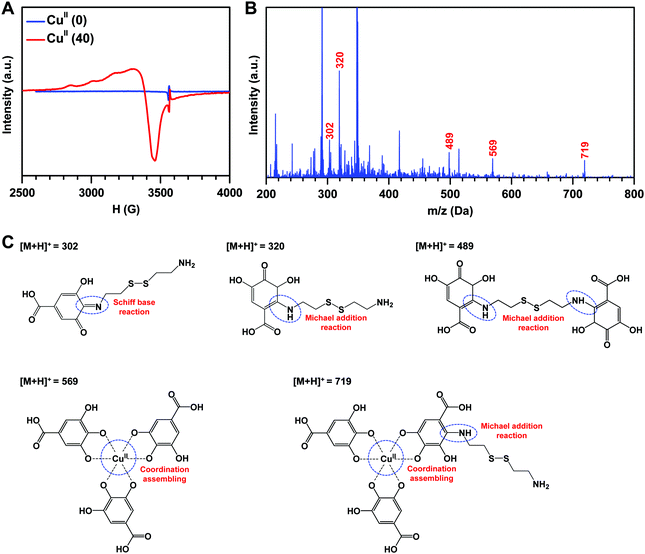 |
| Fig. 3 EPR spectra and MALDI MS analysis of the CuII–GA/CySA coatings. (A) EPR spectra of CuII(0) and CuII(40)–GA/CySA coatings. (B) MALDI-MS spectra of CuII(40) coating. (C) Possible molecule/ion co-assembly of GA, Cu2+ and CySA based on EPR and MALDI-MS analysis. | |
A real-time chemiluminescent assay was used to evaluate in vitro the generation of NO from CuII–GA/CySA coated PVC surface in deoxygenated NO donor solution (pH 7.4), consisting of 10 μM GSNO and 10 μM GSH. The real-time monitoring of the NO production patterns revealed a stable release of NO from the CuII–GA/CySA coatings (Fig. 4A). This suggests a robust chelation of Cu2+ to CuII–GA/CySA network. More importantly, Cu was chelated in a dose-dependent manner, which is supported by the release rates of NO produced by CuII–GA/CySA coatings (Fig. 4B), confirming the successful formation of the metal–phenolic surface coating for Cu chelation. Cu was chelated in a dose dependent manner, supported by the dependence of NO release on the feed concentration of CuCl2·2H2O. We also found that the NO release rate of CuII–GA/CySA coatings was a function of the feed concentration of CuCl2·2H2O and reached a value of up to ∼18 × 10−10 mol cm−2 min−1 when 60 μg mL−1 of CuCl2·2H2O was used for coating preparation. Additionally, the CuII–GA/CySA coatings, in particular the CuII(40) coating, exhibited excellent retention of NO catalytic activity. After continuous exposure to the NO donor solution for 30 days, the CuII(40) coating maintained ∼51% of the NO release compared to a native CuII(40) coating (Fig. 4C), owing to the good preservation of Cu content within the coating (Fig. 4D). Such good preservation of Cu and the resulting catalytic activity for NO formation imply a promising application in long-term indwelling biomedical devices. The actual level of endogenous GSNO in the human blood was lower than that used for NO generation in this work. Therefore, NO-generating behavior of the coating using lower levels of GSNO (e.g., 1 μM) was further investigated. We found that the lower level of GSNO led to a reduction in the catalytic release of NO induced by the CuII–GA/CySA coating. The release rate of NO induced by the CuII(40) coating was reduced to 5.6 ± 0.63 × 10−10 mol cm−2 min−1 when 1 μM GSNO was used (Fig. S4, ESI†), indicating the significant influence of concentration of RSNO on the capacity of NO production. It is noteworthy to mention that the considerable release rate of NO generated by the CuII–GA/CySA coating in such a low level of GSNO also demonstrated its high catalytic activity.
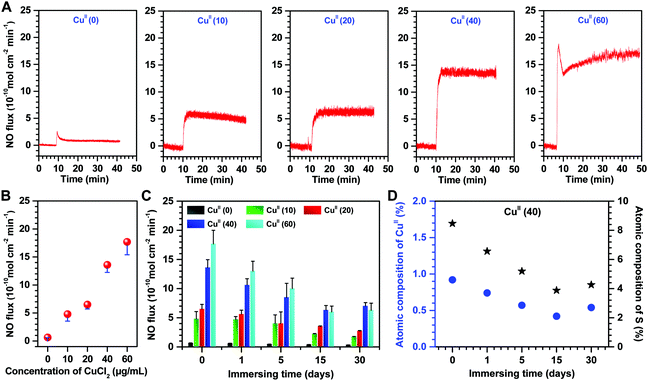 |
| Fig. 4 (A) Catalytic NO generation patterns induced by CuII–GA/CySA coated PVC substrates from deoxygenated PBS (pH 7.4) containing 10 μM GSNO, and 10 μM GSH at 37 °C. (B) Influences of the content of Cu2+ in the CuII–GA/CySA coatings on NO release rate. (C) NO release from CuII–GA/CySA coatings after exposure to the NO donor solution consisting of 10 μM GSNO and 10 μM GSH for up to 30 days, rated to initial as 100%. Such CuII coatings were prepared with different feed concentrations of CuCl2·2H2O ranging from 0 to 60 μg mL−1. (D) Atomic compositions of Cu (blue) and S (black) retained on the surface of CuII(40) coating after inmmersion into the NO donor for up to 30 days. The NO donor solution was replaced every 12 hours. Atomic compositions of the coatings were determined by XPS. Data presented as mean ± SD (n = 4). | |
Reduced adhesion and activation of platelets in vitro
NO is essential in preventing thrombosis as it inhibits platelet adhesion and activation27,32 through up-regulating the expression of cyclic guanylate monophosphate (cGMP).33–36 In order to verify the biological effects of the CuII–GA/CySA coatings on platelets, the platelet adhesion in vitro and the cGMP synthesis were evaluated. In view of the short half-lives of most endogenous RSNO species and the consequent deficiency of RSNOs in PRP, PRP supplemented with GSNO (one of the most typical endogenous RSNO species) was used for the in vitro platelet assay. The concentration of cGMP synthesized by platelets incubated with uncoated and CuII–GA/CySA coated PVC for 2 hours revealed that it was equally low for all surfaces in the group without supplement of the NO donor, whereas the addition of NO donor in PRP led to a remarkable increase in the cGMP concentration (Fig. S5, ESI†). This increase in cGMP formation demonstrated that the physiologic activity of NO catalytically formed the CuII–GA/CySA coatings. Upon further investigation, it was also found that the cGMP synthesis in the groups of CuII–GA/CySA coatings increased with the increase of catalytic NO release. The biological activity of cGMP formation was confirmed by analyzing platelet adhesion with the use of SEM. In the group without the NO donor, platelets on all surfaces were severely activated, presented in a full spreading state (Fig. 5A). After supplement of the NO donor, the CuII–GA/CySA coated surfaces showed an impressive inhibition of the platelet adhesion/activation. Although some platelets were observed on their surfaces, most platelets remained in a resting, nonactivated state. In particular, coating PVC with CuII(40) and CuII(60) reduced platelet adhesion and activation by approximately 17- and 38-fold respectively, as compared to the uncoated PVC (Fig. 5B and C).
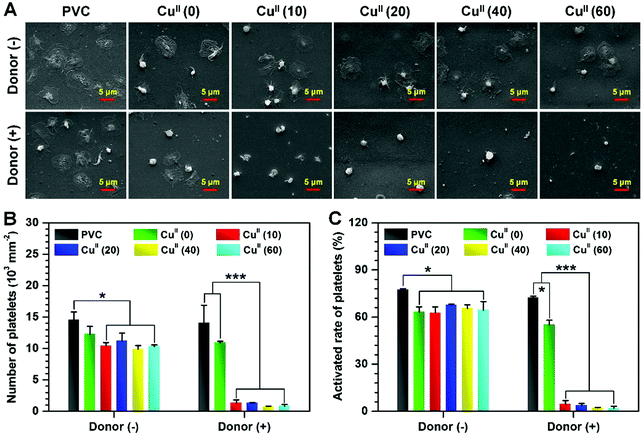 |
| Fig. 5 (A) Representative SEM images of platelet adhesion in vitro. Statistical results of (B) the number and (C) the activated rate of platelets incubated with uncoated and CuII–GA/CySA coated PVC for 2 hours. Data presented as mean ± SD and analyzed using a one-way ANOVA, *p < 0.05, ***p < 0.001. | |
Hemolysis and cytotoxicity test
A hemocompatible blood-contacting material should not induce activation of coagulation factors, platelet adhesion/activation and hemolysis. We thereby determined the hemolysis rate of our new synthesized material of CuII–GA/CySA. The hemolysis ratios of the CuII–GA/CySA-coated PVC revealed that their values were all within 1% (Table S1, ESI†), and were far below the accepted threshold value of 5%,37 suggesting that these coatings have good hemocompatibility.
Additionally, coatings used for blood-contacting indwelling biomedical devices (such as central venous catheters) inevitably come into contact with the endothelium of the blood vessel. Therefore, evaluation of the cytotoxicity against endothelial cells (ECs) was necessary. The metabolic Cell Counting Kit-8 (CCK-8) was used to investigate the cytotoxicity of the CuII–GA/CySA coatings (Fig. S6, ESI†). The CuII–GA/CySA coatings enhanced proliferation of ECs, indicating a good biocompatibility of the CuII–GA/CySA coatings, implying it to be a promising platform for the engineering of blood-contacting devices.
Antibacterial properties of the CuII–GA/CySA coating
Given the efficient anticoagulation and excellent biocompatibility of the CuII–GA/CySA coatings in vitro, it was explored whether such a metal–phenolic surface functionalization strategy could combat bacteria. In this study, representative bacterial strains, namely E. coli and S. aureus were selected, as they are Gram-negative and Gram-positive bacteria, respectively, and also are the most prevalent bacterial species with regards to the development of stent graft infections.38,39 The CuII–GA/CySA coating on PVC led to effective suppression of both E. coli and S. aureus (Fig. 6, Fig. S7 and Table S2, ESI†). The antibacterial effect was strongly dependent on the content of CuII in the CuII–GA/CySA coatings, and the antibacterial rate reached a value of up to 99% when the feed concentration of CuCl2·2H2O increased to 20 μg mL−1 (Fig. 6A and B).
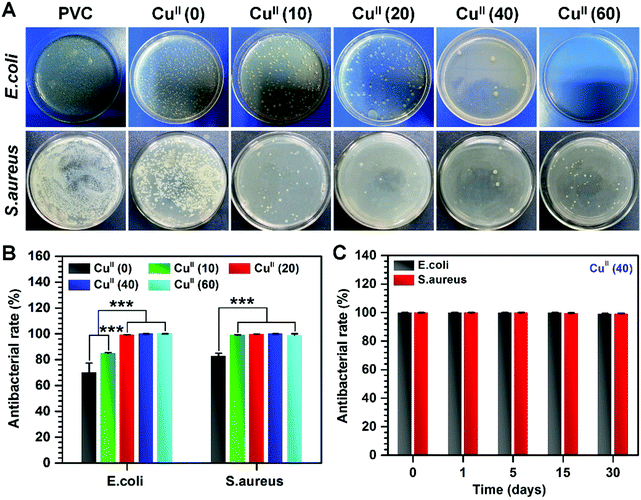 |
| Fig. 6 (A) Representative photographs of colonization by E. coli and S. aureus on bare PVC substrates and after coating with CuII–GA/CySA. (B) Quantitative results of the antibacterial rates of different CuII–GA/CySA coatings against E. coli and S. aureus. (C) Antibacterial rate of CuII(40)-coated PVC against E. coli and S. aureus after continuous exposure to the NO donor consisting of 10 μM GSNO and 10 μM GSH for up to 30 days; the good preservation of the antibacterial effects of CuII(40)-coated PVC after continuous exposure to PBS containing NO donor reveals excellent stability of the CuII(40) coatings and sufficient dose of CuII retained in the CuII(40) coatings. The NO donor solutions were replaced every 12 hours. Data presented as mean ± SD and analyzed using a one-way ANOVA, ***p < 0.001. | |
With regards to the NO catalytic behavior, retention of Cu content, in vitro platelet adhesion, hemolysis rate, cytotoxicity and antibacterial properties of the CuII–GA/CySA coatings, 40 μg mL−1 CuCl2·2H2O feed concentration was optimal to form biocompatible and bioactive CuII–GA/CySA coatings. To evaluate the durability of the antibacterial effect of the CuII–GA/CySA coating, before this study, the CuII(40)-modified PVC was continuously immersed into NO donor for 1, 5, 15 and 30 days. As shown in Fig. 6C, the CuII(40)-modified PVC after continuous treatment by PBS containing NO donor for up to 30 days still exhibited ∼99% antibacterial rate, suggesting the suitability for long-term clinical application.
Reduced thrombosis in vivo
To examine the antithrombogenic properties of CuII–GA/CySA coated PVC, an ex vivo perfusion experiment in New Zealand rabbits without any systemic heparin anticoagulation was performed. We assembled bare and CuII(40) coated PVC tubes into an AV shunt as shown in Fig. 7A. The abilities of the CuII–GA/CySA coatings to prevent thrombosis and support patency of the circuit and blood flow in the AV shunt were then evaluated. To investigate the long-term anti-thrombogenic effect of the CuII(40)-coated tubes, these tubes were immersed in NO donor solution, which was replaced every 12 hours for 30 days (marked as CuII(40)-30 days) for the antithrombogenic test.
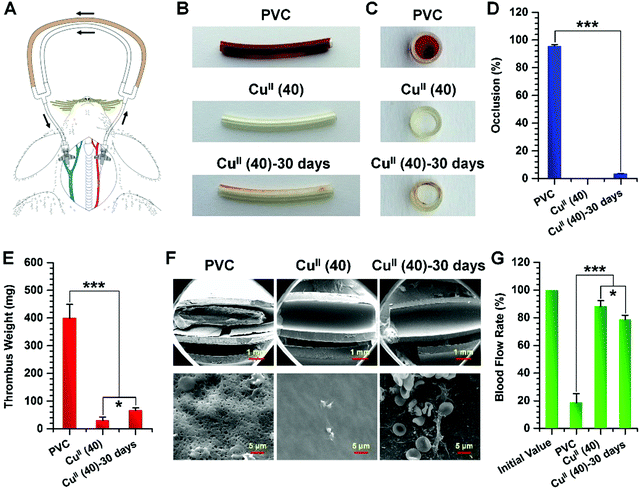 |
| Fig. 7 (A) Scheme of the New Zealand white rabbit AV shunt model exhibiting placement of the cannulae in the carotid artery and jugular vein connected by PVC tubing. (B) Photographs and (C) cross sectional photographs of the bare, CuII(40)-coated and CuII(40)-30 days-coated PVC tubings. (D) Occlusion rate of the bare PVC tubing and after coating with CuII(40). (E) Thrombus weight on bare PVC tubings and after coating with CuII(40). (F) SEM images of the luminal sides of the PVC tubings after 2 hours of circulation. (G) Blood flow rates in different PVC tubing circuits at the end of circulation experiments. Data presented as mean ± SD and analyzed using a one-way ANOVA, *p < 0.05, ***p < 0.001. | |
After 2 hours of ex vivo circulation in the AV shunts with systemic supplement of NO donor, the circuit of uncoated PVC presented complete occlusion, whereas the circuit coated with CuII(40) showed no detectable occlusion (Fig. 7B and C). Despite the detectable occlusion in the group of CuII(40)-30 days, it had only 3.4 ± 0.2% occlusion (the cross-section diameter of the tubing was used for assessing the occlusion) (Fig. 7D). Evaluation of the thrombi harvested from the circuits after 2 hours of circulation revealed that both the CuII(40) and the CuII(40)-30 days-functionalized groups presented a significant decrease in occlusive thrombosis. The total thrombus weights in the CuII(40) and CuII(40)-30 days-coated circuits decreased from 399.1 ± 49.4 mg in uncoated PVC to 30.4 ± 12.0 and 66.4 ± 8.8 mg, respectively (Fig. 7E). SEM analysis revealed that both CuII(40) and CuII(40)-30 days functionalized PVC and impressively prevented the formation of thrombi on their surfaces (Fig. 7F). On the internal surface of the uncoated PVC tubing, severe thrombi were observed, whereas only a few scattered non-activated platelets were found on the CuII(40)-coated PVC. Although a small amount of thrombus was detected in the CuII(40)-30 days-modified PVC tubing, the vast majority of the area was free of blood clots, suggesting that it also possessed excellent antithrombogenic properties.
In addition, as the undisturbed blood flow in the implanted tubing lumen plays a vital role in hemocompatibility, the flow rates in the PVC tubing circuits after 2 hours of circulation were further tested. As shown in Fig. 7G, the CuII(40)- and the CuII(40)-30 days-modified PVC tubing retained 88.2 ± 4.1 and 78.7 ± 3.1% of the initial blood flow rate, respectively, which were much higher than 18.6 ± 6.6% in the group of the unmodified PVC tubing. The good maintenance of the antithrombogenic effects of CuII(40)-coated PVC was attributed to the good retention of CuII in the coatings after continuous immersion into NO donor solution, demonstrating the long-term thromboprotective property.
Surface modification is considered as one of the best means to prevent the clinical complications (e.g. thrombosis and infections) of the blood-contacting indwelling medical devices and extracorporeal circuits because it avoids the systemic risks associated with anticoagulant drugs and antibiotics. Although encouraging progress has been achieved, many available strategies have significant limitations for practical use. For instance, although the immobilization of the biomolecules with anti-fouling property significantly suppressed the thrombogenicity and bacterial adhesion, it also inhibits cell adhesion and growth, limiting the clinical success. Other surface treatments, like micro- and nanostructuring or superhydrophobic coating usually involve mandatory material surface pretreatment and dreary multistep processes.
Since Lee et al. introduced polydopamine coating inspired by mussel protein in 2007 as universal surface chemistry,40 catecholamine surface chemistry and metal–phenolic surface chemistry have been widely used in many fields of science and engineering, in biomedicine,41 energy storage,42 and environmental sciences1,18 due to the simplicity of application. We recently reported a catalytic NO-generating metal–phenolic surface chemistry,43 which expands such material-independent surface chemistry in the field of catalysis. The introduction of copper opened a new application of metal–phenolic surface chemistry for antithrombotic NO catalysis. However, the potential antibacterial effects of copper–phenolic coatings still needed to be explored. Herein, the strategy of copper–phenolic–amine surface chemistry was explored to engineer dual-functional blood-contacting devices with antibacterial and anticoagulant properties. Our strategy of copper–phenolic surface chemistry for tailoring the functionalities of blood-contacting materials demonstrated not only a controllable and long-term anticoagulant NO catalytic activity, but also durable antibacterial effects. Importantly, the resultant copper–phenolic–amine coatings are friendly to cells, indicating the suitability for wider application.
4. Conclusions
In summary, we have developed a straightforward and universal strategy to fabricate a novel dual functional coating with a metal–phenolic–amine network, which could confer vascular devices with long-term antithrombotic and antibacterial properties. These specific functionalities were realized by the incorporation of CuII ions into a metal–phenolic–amine network based on the simple ion/molecule co-assembly of CuII, GA and CySA. The CuII ions in the resulting coatings not only allow the modified surfaces to be bacterial resistant, but also catalytically generate NO from endogenous RSNO in blood to prevent platelet adhesion and activation, and impressively reduce thrombosis in vivo. As the durable metal–phenolic–amine network provides the resultant coating with excellent stability, it retains the properties of antibacterial and antithrombogenic effects over a long term period. We envision that this copper–phenolic–amine surface functionalization strategy can serve as a propitious and functional solution to the complications of indwelled blood-contacting biomedical devices in clinical applications.
Conflicts of interest
There are no conflicts to declare.
Acknowledgements
This work was supported by the National Key Research and Development Program of China (2017YFB0702504), the National Natural Science Foundation of China (Project 31570957 and 31500778), the Fundamental Research Funds for the Central Universities (Project 2682018ZT23) and the start-up fund (1-ZE7S) and central research fund (G-YBWS) from the Hong Kong Polytechnic University.
References
- B. Furie and B. C. Furie, N. Engl. J. Med., 2008, 359, 938–949 CrossRef CAS PubMed
.
- E. A. Vogler and C. A. Siedlecki, Biomaterials, 2009, 30, 1857–1869 CrossRef CAS PubMed
.
- S. Li and J. J. Henry, Annu. Rev. Biomed. Eng., 2011, 13, 451–475 CrossRef CAS PubMed
.
- R. C. Moellering Jr, J. Antimicrob. Chemother., 2011, 67, 4–11 CrossRef
.
- B. Allegranzi, S. B. Nejad, C. Combescure, W. Graafmans, H. Attar, L. Donaldson and D. Pittet, Lancet, 2011, 377, 228–241 CrossRef
.
- G. Conn, A. G. Kidane, G. Punshon, R. Y. Kannan, G. Hamilton and A. M. Seifalian, Expert Rev. Med. Devices, 2006, 3, 245–261 CrossRef CAS PubMed
.
- J. Aw, F. Widjaja, Y. Ding, J. Mu, Y. Liang and B. Xing, Chem. Commun., 2017, 53, 3330–3333 RSC
.
- H. Ji, H. Sun and X. Qu, Adv. Drug Delivery Rev., 2016, 105, 176–189 CrossRef CAS PubMed
.
- M. P. Pai, R. C. Mercier and S. A. Koster, Ann. Pharmacother., 2006, 40, 224–228 CrossRef CAS PubMed
.
- M. de Hoog, J. W. Mouton and J. N. van den Anker, Clin. Pharmacokinet., 2004, 43, 417–440 CrossRef CAS PubMed
.
- I. Banerjee, R. C. Pangule and R. S. Kane, Adv. Mater., 2011, 23, 690–718 CrossRef CAS PubMed
.
- S. Jiang and Z. Cao, Adv. Mater., 2010, 22, 920–932 CrossRef CAS PubMed
.
- H. P. Felgueiras, L. Wang, K. Ren, M. Querido, Q. Jin, M. Barbosa, J. Ji and M. Martins, ACS Appl. Mater. Interfaces, 2017, 9, 7979–7989 CrossRef CAS PubMed
.
- T. Sun, H. Tan, D. Han, Q. Fu and L. Jiang, Small, 2005, 1, 959–963 CrossRef CAS PubMed
.
- D. C. Leslie, A. Waterhouse, J. B. Berthet, T. M. Valentin, A. L. Watters, A. Jain, P. Kim, B. D. Hatton, A. Nedder and K. Donovan, Nat. Biotechnol., 2014, 32, 1134–1140 CrossRef CAS PubMed
.
- N. Holten-Andersen, T. E. Mates, M. S. Toprak, G. D. Stucky, F. W. Zok and J. H. Waite, Langmuir, 2008, 25, 3323–3326 CrossRef PubMed
.
- H. Ejima, J. J. Richardson, K. Liang, J. P. Best, M. P. van Koeverden, G. K. Such, J. Cui and F. Caruso, Science, 2013, 341, 154–157 CrossRef CAS PubMed
.
- H. Ejima, J. J. Richardson and F. Caruso, Nano Today, 2017, 12, 136–148 CrossRef CAS
.
- R. Pashley, Adv. Colloid Interface Sci., 1982, 16, 57–62 CrossRef CAS
.
-
J. N. Israelachvili, in Intermolecular and Surface Forces, ed. J. N. Israelachvili, Academic Press, Boston, 3rd edn, 2011, vol. 15, pp. 341–380 Search PubMed
.
- G. P. Maier, M. V. Rapp, J. H. Waite, J. N. Israelachvili and A. Butler, Science, 2015, 349, 628–632 CrossRef CAS PubMed
.
- G. Grass, C. Rensing and M. Solioz, Appl. Environ. Microbiol., 2011, 77, 1541–1547 CrossRef CAS PubMed
.
- G. Kirakosyan, K. Trchounian, Z. Vardanyan and A. Trchounian, Cell Biochem. Biophys., 2008, 51, 45–50 CrossRef CAS
.
- M. K. Ballo, S. Rtimi, S. Mancini, J. Kiwi, C. Pulgarin, J. M. Entenza and A. Bizzini, Appl. Microbiol. Biotechnol., 2016, 100, 5945–5953 CrossRef CAS PubMed
.
- S. Hwang, W. Cha and M. E. Meyerhoff, Angew. Chem., 2006, 118, 2811–2814 CrossRef
.
- Z. Yang, Y. Yang, L. Zhang, K. Xiong, X. Li, F. Zhang, J. Wang, X. Zhao and N. Huang, Biomaterials, 2018, 178, 1–10 CrossRef CAS PubMed
.
- P. N. Coneski and M. H. Schoenfisch, Chem. Soc. Rev., 2012, 41, 3753–3758 RSC
.
- Z. Yang, Y. Yang, K. Xiong, X. Li, P. Qi, Q. Tu, F. Jing, Y. Weng, J. Wang and N. Huang, Biomaterials, 2015, 63, 80–92 CrossRef CAS
.
- Z. Yang, Q. Tu, M. F. Maitz, S. Zhou, J. Wang and N. Huang, Biomaterials, 2012, 33, 7959–7971 CrossRef CAS
.
- B.E. ISO, 22196, Measurement of antibacterial activity on plastics and other nonporous surfaces, Geneva: International Organization for Standardization, 2011.
- X. Li, H. Qiu, P. Gao, Y. Yang, Z. Yang and N. Huang, NPG Asia Mater., 2018, 10, 482–496 CrossRef CAS
.
- C. Moore, C. Tymvios and M. Emerson, J. Thromb. Haemostasis, 2010, 104, 342–349 CrossRef CAS
.
- W. P. Arnold, C. K. Mittal, S. Katsuki and F. Murad, Proc. Natl. Acad. Sci. U. S. A., 1977, 74, 3203–3207 CrossRef CAS
.
- C. K. Mittal, J. M. Braughler, K. Ichihara and F. Murad, Biochim. Biophys. Acta, 1979, 585, 333–342 CrossRef CAS
.
- F. Murad, Biosci. Rep., 2004, 24, 452–474 CrossRef PubMed
.
- T. Malinski, Am. J. Cardiol., 2005, 96, 13–24 CrossRef
.
- L. Li, M. Tu, S. Mou and C. Zhou, Biomaterials, 2001, 22, 2595–2599 CrossRef CAS
.
- D. D. Bannerman, M. J. Paape, J. W. Lee, X. Zhao, J. C. Hope and P. Rainard, Clin. Diagn. Lab. Immunol., 2004, 463, 463–472 Search PubMed
.
- C. Setacci, E. Chisci, F. Setacci, L. Ercolini, G. D. Donato, N. Trois, G. Galzerano and S. Michelagnoli, Aorta, 2014, 256, 255–264 CrossRef
.
- H. Lee, S. M. Dellatore, W. M. Miller and P. B. Messersmith, Science, 2007, 318, 426–430 CrossRef CAS
.
- G. Pan, S. Sun, W. Zhang, R. Zhao, W. Cui, F. He, L. Huang, S. Lee, K. J. Shea, Q. Shi and H. Yang, J. Am. Chem. Soc., 2016, 138, 15078–15086 CrossRef CAS
.
- J. F. Gnichwitz, R. Marczak, F. Werner, N. Lang, N. Jux, D. M. Guldi, W. Peukert and A. Hirsch, J. Am. Chem. Soc., 2010, 132, 17910–17920 CrossRef CAS PubMed
.
- Z. Yang, Y. Yang, K. Xiong, J. Wang, H. Lee and N. Huang, Chem. Mater., 2018, 30, 5220–5226 CrossRef CAS
.
Footnotes |
† Electronic supplementary information (ESI) available. See DOI: 10.1039/c8qm00458g |
‡ Qiufen Tu and Xuehong Shen contributed equally to this work. |
|
This journal is © the Partner Organisations 2019 |