A differentially selective probe for trivalent chemosensor upon single excitation with cell imaging application: potential applications in combinatorial logic circuit and memory devices†
Received
25th August 2018
, Accepted 31st October 2018
First published on 1st November 2018
Abstract
A new rhodamine 6G-benzylamine-based sensor (L1), having only hydrocarbon skeletons in the extended part, was synthesized and characterized by single-crystal X-ray crystallographic study. It exhibited excellent selective and sensitive recognition of trivalent metal ions M3+ (M = Fe, Al and Cr) over mono- and di-valent and other trivalent metal ions. A large enhancement of the fluorescence intensity for Fe3+ (41-fold), Al3+ (31-fold) and Cr3+ (26-fold) was observed upon the addition of 3.0 equivalent of these metal ions into the probe in H2O/CH3CN (4
:
1, v/v, pH 7.2) with naked eye detection. The corresponding Kf values were evaluated to be 9.4 × 103 M−1 (Fe3+), 1.34 × 104 M−1 (Al3+) and 8.7 × 103 M−1 (Cr3+). Quantum yields of the L1, [L1–Fe3+], [L1–Al3+] and [L1–Cr3+] complexes in H2O/CH3CN (4
:
1, v/v, pH 7.2) were found to be 0.012, 0.489, 0.376 and 0.310, respectively, using rhodamine-6G as standard. LODs for Fe3+, Al3+ and Cr3+ were determined by 3σ methods and found to be 1.28, 1.34 and 2.28 μM, respectively. Cyanide ion scavenged Fe3+ from the [Fe3+–L1] complex and quenched its fluorescence via its ring-closed spirolactam form. Advanced level molecular logic devices using different inputs (2 and 4 inputs) as advanced level logic gates and memory devices were constructed. The large enhancement in fluorescence emission of L1 upon complexation with M3+ metal ions makes the probe suitable for the bio-imaging of M3+ (M = Fe, Al and Cr) in living cells.
Introduction
With the increase in urbanization and socioeconomic activities, unlike other pollutants like petroleum hydrocarbons and domestic and municipal litter, which may visibly build up in the environment, the traces of heavy metal ions increase the toxicity level to a higher extent in the environment and also cause harmful effects on human health. Nowadays, contamination by toxic metal ions is increasing due to leather tanning, electroplating, pigments, emissions from vehicular traffic gas exhausts, energy and fuel production, intensive agriculture and sludge dumping and from mining industries.
As a result, drinking water and food, especially in developing countries, with metal contamination is very much unsafe. So many researchers have tried to detect toxic metal ions, such as iron,1 chromium,2 aluminium,3,4 lead,5 silver,6 cadmium,7 zinc8,9 and mercury,10 in water and foods.
Among these trivalent metal ions, Fe3+, Al3+and Cr3+ have biological as well as environmental importance.11–24 Fe3+ is not only the most abundant transition metal in cellular systems but also plays an important role in many metabolic pathways, such as oxygen transport processes in tissues, nerves signal conduction, cellular growth and tissue formation.25 On the other hand, the excess accumulation of Fe3+ can lead to a variety of diseases, such as cell damage and organ dysfunction through the abnormal production of reactive oxygen species (ROS),26,27 leading to Alzheimer's, Huntington's, Parkinson's etc. diseases.28 Moreover, disruption of iron homeostasis can lead to a number of disease, such as cancer,29 hepatitis30 and neurodegenerative diseases.31
Cr3+ is an effective nutrient and gives immunity power to the human body. Cr3+ overdose is known to inflict a negative effect on normal enzymatic activities, and the cellular structure and function causing a disturbance in glucose levels and lipid metabolism, while a deficiency of Cr3+ in humans can cause maturity-onset diabetes and cardiovascular disease and nervous system disorders.32,33 The Cr3+ ion, present in the cytoplasm, is known to bind non-specifically to DNA at an elevated level, affecting the cellular structures and damaging the cellular components, which can lead to mutation and cancer.34 Chromium deficiency can cause a risk of diabetes, cardiovascular diseases and nervous system disorders.35
Al3+ is the third most abundant metal in the Earth's crust and also one of the most common species of metal cations that are mostly found in the +3 oxidation state in most kinds of animal and plant tissues and in natural waters everywhere.36–40 It has been found that aluminium accumulates in various mammalian tissues, such as the brain, bone, liver and kidney,41,42 which causes renal failure43 and problems associated with age.44 Aluminium toxicity damages the central nervous system and it is surmised to play a role in neurodegenerative Alzheimer's and Parkinson's diseases. It is also responsible for intoxication in haemodialysis patients.45 Moreover, aluminium toxicity may cause gastrointestinal problems and interference with Ca2+ metabolism.46–48 Again, increasing free Al3+ due to acid rain and human activities in the environment and surface water is detrimental to growing plants.49
Various methods, such as inductively coupled plasma emission spectrometery (ICP),50 X-ray photoelectron spectrometry (XPS) and atomic fluorescence spectroscopy (AFS) have been used for heavy metal ion detection.51,52 Compared with these complicated methods, optical probes are inexpensive, simple and rapid.
Thus, there is an urgent need to design single fluorogenic probes, displaying changes in optical properties through a “turn-on” response that are capable of detecting the presence of Fe3+, Al3+ and Cr3+ ions simultaneously and in the presence of large number of monovalent, divalent and other trivalent metal cations53–55 in biological samples.
As Cr3+ and Fe3+ are paramagnetic in nature, they function as fluorescent quenchers,56 which makes it a challenging task to develop a turn-on fluorescent sensor for these ions. Very few turn-on sensors for Cr3+ and Fe3+ have been reported with cell-imaging applications.57–59
Although, Al3+ functions as a turn-on fluorescent sensor, due to its strong hydration in water, most of the reported dye-based Al3+ sensors require organic solvents or mixed solvents, with very few being suitable for Al3+-imaging applications.60
Given our interest in developing new chemosensors, we report herein a rhodamine 6G-based probe (Scheme 1), characterized by X-ray single-crystal diffraction analysis (Fig. 1) and by other common spectroscopic analysis, for the detection of trivalent cations, like Fe3+, Al3+ and Cr3+, in an aqueous medium over monovalent, divalent and other trivalent metal ions. Though there are a few61,62 reports on trivalent sensors, where in all cases external coordinating atom(s) are present along with the basic amidic moiety of rhodamine, however, in our reported probe it is absent. Herein, we disclose a benzylamine-rhodamine-6G (L1) conjugate (Scheme 1) that selectively senses these trivalent metal ions in a mostly aqueous medium (4
:
1, H2O
:
CH3CN, v/v) with very high fluorescence enhancement.
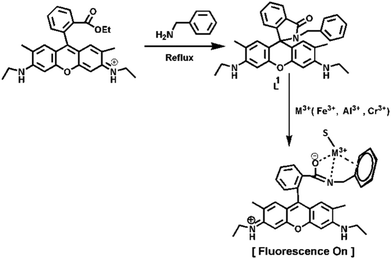 |
| Scheme 1 Tentative binding mode of L1 with M3+. | |
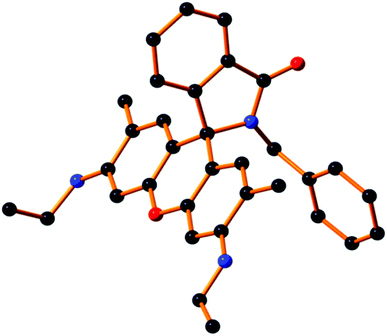 |
| Fig. 1 The molecular view of ligand L1. All H-atoms are omitted for clarity. | |
Experimental section
Materials and methods
All solvents used for synthesis were of reagent grade (Merck). For the spectroscopic (UV/Vis and fluorescence) studies, HPLC-grade MeCN and double-distilled water were used. Rhodamine 6G hydrochloride and metal salts, such as perchlorates of Na+, Fe2+, Co2+, Ni2+, Zn2+, Pb2+, Cd2+, Hg2+, Cu2+, Al(NO3)3·9H2O, Cr(NO3)3·9H2O, Fe(NO3)3·9H2O were purchased either from Sigma–Aldrich or Merck and used as received. All other compounds were purchased from commercial sources and used without further purification.
Physical measurements
1H-NMR spectra were recorded in CDCl3 and DMSO-d6, on a Bruker 300 MHz NMR spectrometer using tetramethylsilane (δ = 0) as an internal standard. Infrared spectra (400–4000 cm−1) were recorded in the liquid state using a Nickolet Magna IR 750 series-II FTIR spectrometer. ESI-MS+ (m/z) of the ligand and complexes were recorded on a Waters’ HRMS spectrometer (Model: XEVO G2QTof). UV-Vis spectra were recorded on an Agilent diode-array spectrophotometer (Model, Agilent 8453). Steady-state fluorescence measurements were performed on a PTI QM-40 spectrofluorometer. Lifetimes were measured using an Horiba Jobin–Yvon Hamamatsu MCP photomultiplier (R3809) and data were analyzed using IBH DAS6 software. The pH of the solutions were recorded using a digital pH meter 335, calibrated using pH 4, 7 and 10 buffers in the range pH 2–12.
Synthesis of rhodamine 6G conjugate (L1)
Rhodamine 6G (5.0 mmol) and benzylamine (10.0 mmol) were dissolved in EtOH and refluxed for 10 h with continuous stirring, whereupon a white crystalline solid of the probe (L1) was deposited (Scheme 1). The solid was filtered and washed several times with ethanol and dried in air (75% yield). The compound (L1), was dissolved in MeOH and refluxed for 2 h with constant stirring and filtered. After 2 days, single crystals suitable for X-ray diffraction studies were obtained. 1H NMR (300 MHz, DMSO-d6) (ppm): 1.18 (t, J = 6.8 Hz, 6H (–CH3)), 1.69 (s, 6H (–Ar–CH3)), 2.49 (s, 2H, (–CH2)), 3.09 (t, J = 6.3 Hz, 4H (–Ar–CH2)), 4.96 (s, 2H, (–NH)), 5.87 (s, 2H, (–Ar–H)), 6.18 (s, 2H, (–Ar–H)), 6.85 (s, 2H, (–Ar–H)), 6.95 (d, J = 5.4 Hz, 4H (–Ar–H)), 7.48 (m, 1H,(–Ar–H)), 7.50 (m, 1H, (Ar–H)), 7.80 (m, 1H, (–Ar–H)) (Fig. S1†). 13C NMR: 14.63, 17.32, 37.90, 43.69, 65.08, 95.90, 104.76, 118.44, 122.84, 124.09, 126.53, 127.75, 128.39, 128.68, 130.92, 133.16, 138.07, 147.90, 151.54, 153.95, 167.38 (Fig. S2†). ESI-MS+ (m/z): 504.26 (L1 + H+) (Fig. S3†). IR spectrum: 1684 cm−1 (–C
O), 1378 cm−1 (–C–N) (Fig. S4†).
Solution preparation for UV-Vis and fluorescence studies
For both the UV-Vis and fluorescence titrations, a stock solution of 1.0 × 10−3 M of the probe L1 was prepared by dissolving 12.58 mg in 25 mL CH3CN. Analogously, 1.0 × 10−3 M stock solutions of Fe3+, Al3+ and Cr3+ were prepared in MeOH. A solution of 20 mM HEPES buffer (4
:
1, H2O
:
CH3CN) was prepared and the pH was adjusted to 7.2 by using HCl and NaOH. For the UV-Vis spectra, a 60 μM probe was taken in a cuvette containing 2.5 mL of buffer solution and then Fe3+ salt solution was added incrementally starting from 0 to 240 μM in a regular interval of time, and the absorption spectra were recorded. Similar experiments were performed for Al3+ and Cr3+. Again 2.5 ml of this buffer solution was pipetted into a cuvette to which 60 μM of the probe (L1) solution was added and Fe3+ salt solution was then added incrementally starting from 0 to 140 μM in a regular interval of time, and the fluorescence spectra were recorded, setting the excitation wavelength at 502 nm. Similar titrations were conducted with Al3+ and Cr3+. Path lengths of the cells used for absorption and emission studies were 1 cm. Fluorescence measurements were performed using a 2 nm × 2 nm slit width.
Cell culture
Human hepatocellular liver carcinoma (HepG2) cell lines (NCCS, Pune, India), were grown in DMEM supplemented with 10% FBS and antibiotics (penicillin, 100 μg ml−1; streptomycin, 50 μg ml−1). Cells were cultured at 37 °C in a 95% air/5% CO2 incubator.
Cell cytotoxicity assay
To assess if there was any cytotoxic effect of the ligand (L1), a cell viability assay was performed using 3-(4,5-dimethylthiazol-2-yl)-2,5-diphenyltetra-zolium bromide (MTT).63 HepG2 cells (1 × 105 cells per well) were cultured in a 96-well plate with incubating at 37 °C, and were treated with increasing concentrations of L1 (1, 10, 20, 40, 60, 80 and 100 μM) for 24 h. After the incubation, 10 μl of MTT solution [5 mg ml−1, dissolved in 1× phosphate-buffered saline (PBS)] was added to each well of the 96-well culture plate, and then incubated at 37 °C for 4 h. Media were decanted from the wells and 100 μL of 0.04 N acidic isopropyl alcohol was added into each well to solubilize the intracellular formazan crystals (blue-violet) formed, and the absorbance of the solutions was measured at 595 nm wavelength (EMax Precision Micro Plate Reader, Molecular Devices, USA). Values were calculated as the mean ± standard errors of three independent experiments. The cell viability was expressed as the optical density ratio of the treatment to control.
Cell-imaging study by fluorescence microscopy
HepG2 cells were cultured in a 35 × 10 mm culture dish on a coverslip for 24 h at 37 °C. The cells were treated with 10 μm solutions of L1, prepared by dissolving L1 into the mixed solvent DMSO
:
water = 1
:
9 (v/v) and incubated for 1 h at 37 °C. To study the complex formation of L1 with the three metal ions (Fe3+, Cr3+, Al3+), HepG2 cells were pre-incubated separately with 10 μM, 20 μM and 40 μM of each of the metal ions for 60 min at 37 °C, followed by washing them twice with 1× PBS and subsequent incubation with 10 μM L1 for 60 min at 37 °C. Fluorescence images of HepG2 cells were taken using a fluorescence microscope (Leica DM3000, Germany) with an objective lens of 40× magnification.
Job's plot
This method is based on the measurement of the fluorescence of a series of solutions in which molar concentrations of the probe (L1) and M3+ vary but their sum remains constant. Here, the fluorescence of each solution was measured at 558 nm and plotted against the mole fraction of M3+. The maximum fluorescence occurred at the mole ratio corresponding to the combined ratio of the two components. The composition of the complex was determined by Job's method and found to be 1
:
1 with respect to L1 for the Fe3+, Al3+ and Cr3+ complexes.
Results and discussion
As depicted in Scheme 1, receptor L1 was synthesized from the reaction of rhodamine-6G with benzylamine in EtOH in reflux conditions for 10 h. The final crystallized product (L1) was well characterized by 1H NMR (Fig. S1†), 13C NMR (Fig. S2†), HRMS (Fig. S3†), IR (Fig. S4†) and a single-crystal X-ray diffraction method (Fig. 1). The receptor L1 was found to be a very sensitive and highly selective colorimetric and fluorogenic chemosensor for trivalent metal ions, M3+ (M3+ = Fe3+, Al3+ and Cr3+), while in the absence of M3+, the solution of L1 was colourless and very weakly fluorescent.
X-ray crystallography study
Single-crystal X-ray diffraction studies revealed that the compound L1 crystallizes in a triclinic system of space group P
(no. 2). The crystallographic details are depicted in Table 1. A molecular view of L1 is shown in Fig. 1, with H atoms are removed to get better clarity.
Table 1 Crystallographic data and details of the structure determination
Molecular formula |
C33H33N3O2 |
Formula weight |
503.62 |
Crystal system |
Triclinic |
Space group |
P (no. 2) |
a/Å |
9.0397(8) |
b/Å |
12.5823(11) |
c/Å |
12.8196(11) |
α/° |
86.214(2) |
β/° |
73.428(2) |
γ/° |
72.357(2) |
V/Å3 |
1331.5(2) |
Z
|
2 |
D(calc)/g cm−3 |
1.256 |
μ(MoKα)/mm−1 |
0.079 |
F(000) |
536 |
T/K |
273 |
θ min, max/° |
2.3, 27.5 |
Dataset |
−11: 11; −16: 16; −16: 16 |
Tot., Uniq. data, R(int) |
13 172, 6036, 0.023 |
Observed data [I > 2σ(I)] |
4637 |
N
ref, Npar |
6036, 356 |
R, wR2, S |
0.0527, 0.1522, 1.05 |
UV-Vis absorption studies
The UV-Vis spectrum of L1 (60 μM) was recorded in a mixed aqueous solvent of H2O/CH3CN (4
:
1, v/v, pH 7.2, 20 mM HEPES buffer). The gradual addition of Fe3+, Al3+ and Cr3+ separately to a solution of L1 revealed that there was a development of two absorption peaks at 350 nm and 530 nm (Fig. 2 and Fig. S5, S5a†), with a sharp visual colour change of the representative solution from colourless to orange-red, whereas no such peaks appeared in the presence of other monovalent, divalent or trivalent metal ion solutions (Fig. S5b†). Between these two peaks, the second one is very important as it exhibits a greater increase in absorbance. The appearance of this peak clearly manifested the opening of the spirolactam ring due to the coordination of Fe3+, Al3+ and Cr3+ with the probe L1. The probable coordination mode of L1 towards M3+ (Fe3+, Al3+ and Cr3+) is demonstrated in Scheme 1. UV-Vis titrations were carried out by varying the trivalent metal ion Fe3+, Al3+ and Cr3+ concentration (0–240 μM) keeping the probe concentration fixed at 60 μM in H2O/CH3CN (4
:
1, v/v, pH 7.2, 20 mM HEPES buffer). Plots of absorbance vs. [M3+] yielded linear curves, which were analyzed by linear curve-fitting of the titration data according to eqn (1) (where a, b and c have the usual meaning) under the conditions 1 ≫ c × x with n = 1, giving apparent association constant Kf values as 1.19 × 104 M−1, 1.09 × 104 M−1 and 1.04 × 104 M−1 for Fe3+, Al3+ and Cr3+, respectively. | y = (a + b × c × xn)/(1 + c × xn) | (1) |
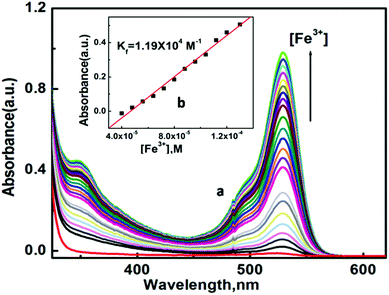 |
| Fig. 2 (a) UV-Vis absorption spectra of L1 (60 μM) in H2O/CH3CN (4 : 1, v/v, pH 7.2, 20 mM HEPES buffer) solutions with the increase in concentration of Fe3+ solution (0–240 μM); (b) linear fit of absorbance vs. [Fe3+] plot. | |
The absorbance intensity of the [L1–Fe3+] complex was found to be selectively quenched in the presence CN− ion (Fig. S6†).
Fluorescence studies
The emission spectra of L1 and its fluorescence titration with M3+ (Fe3+, Al3+ and Cr3+) were performed in H2O/CH3CN (4
:
1, v/v, pH 7.2, 20 mM HEPES buffer) with the fixed concentration of L1 at 60 μM. A significant turn-on fluorescence response was observed in the presence of Fe3+, Al3+ and Cr3+ with a fluorescent maximum at 558 nm. For example, upon the gradual addition of Fe3+ (0–3 equivalent) to the non-fluorescent solution of L1, a 41-fold enhancement in fluorescence intensity at 558 nm was observed following excitation at 502 nm, which also suggests the opening of the spirolactam ring in L1 upon coordination to the Fe3+ ion64 (Fig. 3). A 31-fold and 26-fold enhancement of fluorescence intensity was observed during the titration of L1 with Al3+ and Cr3+ respectively (Fig. S7 and S7a†). Fascinatingly, this change was also accompanied with a naked-eye colour change from colourless to orange-red after the addition of Fe3+, Al3+ and Cr3+, indicating that the probe L1 is a highly sensitive colorimetric chemosensor for these trivalent metal cations.
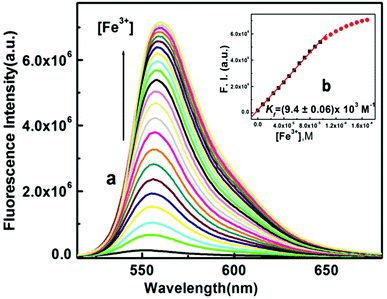 |
| Fig. 3 (a) Fluorescence spectra of L1 (60 μM) in H2O/CH3CN (4 : 1, v/v, pH 7.2, 20 mM HEPES buffer) solutions upon the addition of Fe3+ (0–3.0 equivalent), each spectrum was taken after 3 min of Fe3+ addition; λex = 502 nm, λem = 558 nm; (b) linear curve fitting of the titration curves with Kf values. | |
Plots of FI vs. [M3+] give linear curves. Linear curve-fitting of the titration data according to eqn (1) (where a, b and c have the usual meaning) gave an apparent association constant Kf = (0.94 ± 0.01) × 104 M−1 for Fe3+ under the conditions 1 ≫ c × x with n = 1. Similarly, binding constants for Al3+ and Cr3+ were calculated and found to be (1.34 ± 0.1) × 104 M−1, Kf = (0.87 ± 0.01) × 104 M−1, respectively (Fig. 4). There was an excellent agreement between the values of Kf obtained from the absorption and fluorescence titration data, manifesting the self-consistency of our results. Using these fluorescence data, the detection limits of the probe L1 for Fe3+, Al3+ and Cr3+ were calculated to be 1.28, 1.34 and 2.28 μM, respectively (Fig. S8, S8a and S8b†). These results strongly indicate that this probe L1 is sensitive enough to detect trace levels of Fe3+, Al3+ and Cr3+. In this context, we must highlight that the quantum yield of the ligand (L1) was very less. The quantum yields of L1 and [L1–Fe3+], [L1–Al3+] and [L1–Cr3+] complexes in H2O/CH3CN (4
:
1, v/v, pH 7.2) were found to be 0.012, 0.489, 0.376, 0.310 respectively using rhodamine-6G as a standard. The comparatively higher values of quantum yields for complexes compared to the free ligands indicate the higher stability of the complexes in the excited states.
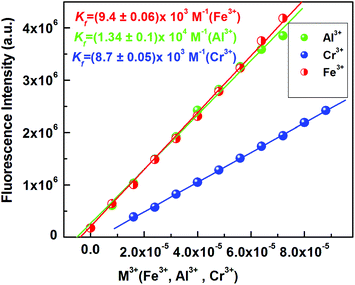 |
| Fig. 4 Linear fitting of the fluorescence titration curves for Fe3+, Al3+ and Cr3+ with Kf values. | |
Job's method was again employed to determine the composition of the complex, which was found to be 1
:
1 (Fig. S9†) and was further supported by the mass spectrometric analysis results (m/z = 724.18 [Fe(L1)(NO3−)2(CH3CN)]+); (m/z = 198.06 [Al(L1)(MeOH)2]3+); 721.52 [Cr(L1)(NO3−)2(CH3CN)]+ (Fig. S3a and S3b†).
Moreover, a conspicuous reddish-orange fluorescence response of the probe upon interaction with M3+ (Fig. 5a) provides the scope for naked eye detection. The possibility of using the chemosensor L1 in the development of paper test strips was examined and it was found that the turn-on fluorescence response of L1 towards M3+ is also visually detectable in test paper strips (Fig. 5b).
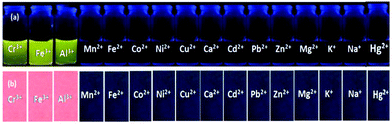 |
| Fig. 5 (a) Visual fluorescent response of L1 towards Fe3+, Al3+ and Cr3+ (under 365 nm UV light). (b) Paper strip for the fluorescent sensing of Fe3+, Al3+ and Cr3+ towards the probe L1. | |
Selectivity studies
Selectivity is an important and essential requirement for an excellent chemosensor. Selectivity experiments were carried out by taking 60 μM of probe L1 in a cuvette containing 2.5 mL of 20 mM buffer solution and then different metal ion solutions of about 5 equivalent were added separately. Surprisingly, L1 could selectively recognize the trivalent metal ions Cr3+, Fe3+ and Al3+ in a mixed aqueous medium over other biologically abundant divalent 3d transition metal cations, like Mn2+, Fe2+, Co2+, Ni2+, Cu2+ and Zn2+, hazardous heavy metal ions, like Pb2+, Cd2+ and Hg2+, alkali and alkaline earth metal ions, like Na+, K+, Ca2+, Mg2+ (Fig. 6 and Fig. S10†) and also in the presence of Ga(III), Y(III), Sm(III), Dy(III), Au(III), Ru(III), Co(III) and Cr(VI) ions (Fig. S17†), which were taken in 5 equivalent with respect to the probe (1
:
5 ratio).
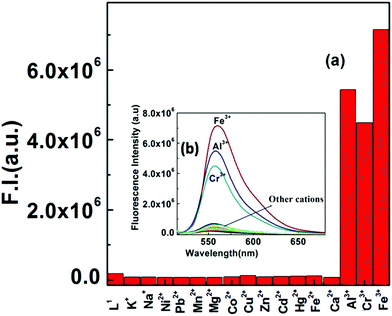 |
| Fig. 6 (a) Fluorescence bar diagram for the selective response of L1 (60 μM) towards M3+ (M = Fe, Al, Cr) over other di- and monovalent metal ions (5 equivalent) in H2O/CH3CN (4 : 1, v/v, pH 7.2, 20 mM HEPES buffer), λex = 502 nm, λem = 558 (nm); (b) fluorescence response of L1 (60 μM) upon the addition of 3.0 equivalent of Fe3+, Al3+, Cr3+. | |
It was also found that not a single anionic species among OAc−, HCO3–, CO32–, S2O32–, SCN−, N3–, NO3–, NO2–, H2PO4–, SO42–, ClO4−, F−, Cl−, Br−, I−, PO43– and CN− could enhance the fluorescence intensity of the probe L1 (Fig. 7) when taken in 5 equivalent with respect to the probe (1
:
5 ratio). However, the fluorescence intensity of the [L1–M3+] complex was found to be selectively quenched in the presence CN− ions (Fig. S11 and S12†). An excellent reversible fluorescence ON–OFF property of L1 was observed through fluorescence study with the sequential addition of M3+ and CN− ions in 20 mM HEPES buffer in H2O/CH3CN (4
:
1) (pH 7.2) solution at room temperature (Fig. 8). The addition of cyanide ions to the solution containing L1–M3+ complex quenched the emission of the probe with the disappearance of the orange-red colour of the solution. The fluorescence quenching of the complexes was characterized by a linear Stern–Volmer (SV) plot and analyzed using the classical Stern–Volmer (SV) eqn (2)
65
| 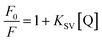 | (2) |
where
F0 and
F are the steady state fluorescence intensities at the maximum wavelength in the absence and presence of a quencher (
Q), respectively, [Q] is the quencher concentration and
KSV is the Stern–Volmer constant. The Stern–Volmer quenching constant (
KSV) of [
L1–Fe
3+], [
L1–Al
3+] and [
L1–Cr
3+] complexes with CN
− ion were calculated and found to be (5.60 ± 0.20) × 10
2 M
−1, (2.42 ± 0.24) × 10
2 M
−1 and (1.88 ± 0.11) × 10
2 M
−1, respectively (Fig. S18
†). The reason behind this observation is that the interaction of M
3+ with the probe results in opening of the spirolactam ring, thereby producing strong fluorescence. Then treatment with CN
− results in the abstraction of a metal ion and regeneration of the spirolactam ring, leading to quenching of the emission. The mechanism between
L1–M
3+ with CN
− ion sensing was confirmed by the HRMS study (Fig. S13
†). It is clear from the HRMS study that the ligand is in a ring-closed structure exactly the same with the
L1. This reversibility test suggests the reusability of this chemosensor.
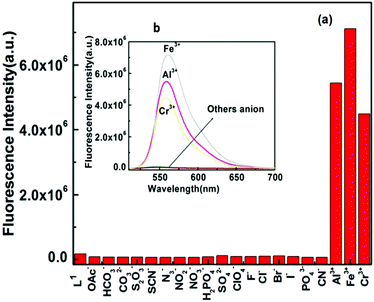 |
| Fig. 7 (a) Histogram of the fluorescence responses of different anions (5 equivalent) towards L1 (60 μM) in 4 : 1 v/v, water/MeCN in HEPES buffer at pH 7.2 with λex = 502 nm, λem = 558 nm. (b) The fluorescence response of L1 towards Fe3+, Al3+, Cr3+ with respect to different anions (300 μM). | |
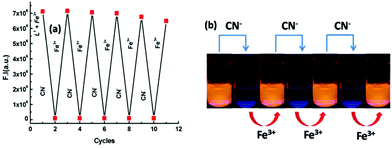 |
| Fig. 8 Fluorescence experiment to show the reversibility and reusability of the receptor for sensing Fe3+ by the alternate addition of CN−: (a) Fluorescence intensity obtained during the titration of L1–Fe3+ with CN− followed by the addition of Fe3+. (b) Visual fluorescent colour changes after each addition of CN− and Fe3+ sequentially. | |
pH studies
For practical application, the appropriate pH condition for the sensor was evaluated. At pH > 4.0, no obvious ring opening of the probe was observed, thereby satisfying the usefulness of the probe in biological systems over a wide pH range (4–8) for the detection of Fe3+ (Fig. 9), Al3+ and Cr3+ (Fig. S14†). However, upon the addition of 3.0 equivalent of Fe3+, the FI jumps to a very high value and remains almost unchanged in the range pH 3–7, but then on a further increase in pH, the FI gradually falls. At pH > 8, no FI was observed in the case of Fe3+, Al3+ and Cr3+ due to the precipitation of hydroxides of these metal ions.
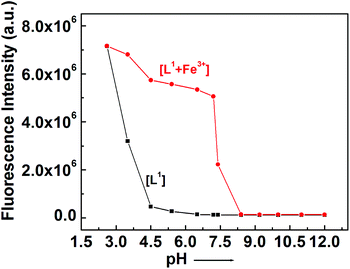 |
| Fig. 9 pH dependence of fluorescence responses of L1 and its [L1–Fe3+] complex. | |
Spectral studies
The mechanistic pathway proposed for the formation of the L1–M3+ complex by the opening of the spirolactam ring was established through IR and 1H-NMR studies. The IR studies revealed that the characteristic stretching vibrational frequencies of the amidic ‘C
O’ of the rhodamine moiety at 1684 cm−1 and azomethine group (C
N) at 1636 cm−1 were shifted to lower wavenumbers 1636, 1637, 1635 cm−1 and 1605, 1604, 1604 cm−1 in the presence of 3.0 equivalent of Fe3+, Al3+ and Cr3+, respectively (Fig. 10). These large shifts in IR frequencies signify a strong polarization of the C
O and C
N bonds upon efficient binding to the M3+ (M = Al, Fe and Cr) ion. The coordination mode of L1 towards Al3+ was supported by 1H-NMR studies (Fig. S1a†), which showed a downfield shift of azomethine proton in L1 and also of the protons on the benzene ring of the benzylamine moiety in the L1–Al3+ complex. The broadening of the –NH proton at 4.9 was due to opening of the spirolactam ring and it bearing a positive charge on it. HRMS study (Fig. S1a†) also confirmed the formation of a complex with M3+ (M = Al, Fe and Cr).
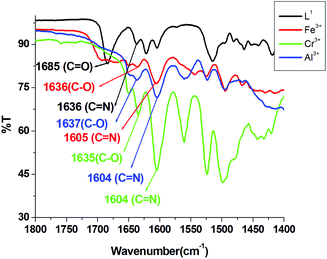 |
| Fig. 10 IR spectra of (L1), [L1 + Fe3+], [L1 + Al3+] and [L1 + Cr3+] complexes in MeOH. | |
Selective sensing of Fe3+, Al3+ or Cr3+
It is desirable for the probe to be selective towards one trivalent metal ion over the other two. The probe was sensitive towards all the three trivalent metal ions, but in the presence of ppi (inorganic pyrophosphate), the fluorescence of L1–Al3+ and L1–Cr3+ were quenched significantly while the fluorescence of L1–Fe3+ remained almost unchanged, making L1 selective towards Fe3+ in the presence of ppi (Fig. S19†). Again in the presence of I−, the fluorescence of L1–Fe3+ and L1–Al3+ undergoes quenching, while that of L1–Cr3+ remains unchanged, making L1 selective towards Cr3+ in the presence of I− (Fig. S20†). Now if L1 is fluorescent towards an unknown solution, but remains non-fluorescent in the presence of both ppi and I−, then the initial fluorescence is due to the presence of Al3+. Thus, L1 can be made selective towards Fe3+, Cr3+ or Al3+ in an unknown solution.
Molecular logic operations
The spectroscopic properties of the probe L1 encouraged us to apply it for multiple logic operations with the sequential addition of inputs like cations, such as Al3+, Fe3+, Cr3+, and CN− anions and to then monitor their emission as the output. An INHIBIT logic gate was constructed with a particular combination of logic operations, like NOT and AND functions, which was important due to its non-commutative behaviour, i.e. its output signal is inhibited by only one type of input. To demonstrate this INHIBIT logic function, first we chose two inputs, namely Fe3+ (Input 1) and CN− (Input 2), and used its emission intensity at 558 nm as the output. A high value of emission intensity (>5 × 105 at 558 nm) has been designated as 1 (ON) and a low value (≤5 × 105) as 0 (OFF). In the absence of both the 1st input (Fe3+) and 2nd input (CN−), the emission intensity was low, indicating the OFF state; whereas when only input 1 was present, then a significant enhancement of the emission (at 558 nm) took place, indicating the 1 (ON) state, while, on the other hand, in the presence of input 2, the output emission value became very weak indicating the OFF state. Therefore, it was necessary to apply NOT gate with Input 2. Additionally, it is noteworthy that L1 displayed the emission output signal in such a way that it seemed to understand the requirements of the AND operation. In the presence of both inputs, the output emission value was again low, indicating the OFF state, in accordance with the truth table (Fig. 11(a)). Thus, by the sequential addition of these two inputs, an INHIBIT function logic gate could be achieved.
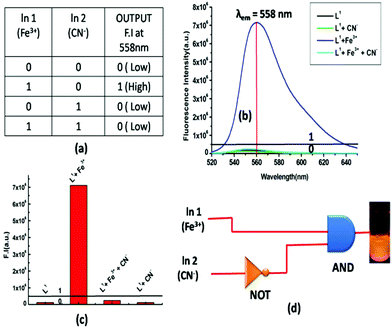 |
| Fig. 11 (a) Corresponding truth table of the logic gate. (b) Output signals (at 558 nm) of the logic gate in the presence of different inputs. (c) Corresponding bar diagram at 558 nm in the presence of different inputs. (d) General representation of an INHIBIT logic gate-based circuit. | |
Advanced level OR-INHIBIT gate based 4 input logic gate
A combination of OR and INHIBIT logic functions was used for the construction of the 4 inputs 1 output logic circuit. Now to imitate an OR logic gate function, the emission intensity at 558 nm was used as the output response similar to the earlier 2 input logic gate, and the inputs were Al3+, Fe3+, Cr3+ and CN− (Fig. S15†). When the 1st (Al3+) and 2nd (Fe3+) inputs were both absent, the output response, i.e. the emission intensity, was very low, indicating the 0 (OFF) state. However, when only any one of the two inputs was present, the output signal was high, indicating the 1 (ON) state. Again in the presence of both the input Al3+ and Fe3+, the output response was 1 (ON). Thus, according to its truth table (Fig. 12a), an OR function logic gate could be contracted by the sequential addition of these two inputs. Then we verified the nature of the output signal in the presence of a 3rd ionic input (Cr3+) in the presence of the first two ionic inputs. Here, any one of these three inputs or the presence of two of these three inputs caused a high intensity emission, output indicating the ON state (1). Thus, the probe behaved like an OR logic function. On the other hand, when only a 4th input (CN−) was present or in the presence of all other inputs (Al3+, Fe3+ and Cr3+) in the system, the output emission was very weak, indicating the 0 (OFF) state. Therefore, we applied a NOT logic function with a 4th input. As the probe functions parallel with the output signal, so we could imply another AND logic function. Thus, from an INHIBIT logical function and following its corresponding truth table, an advanced level 4 input logic gate circuit could be constructed (Fig. 12b).
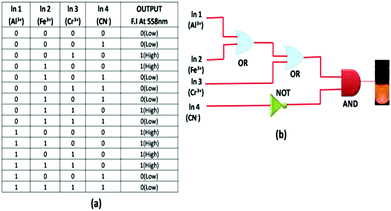 |
| Fig. 12 (a) Truth table of an advanced level 4 input logic gate. (b) Schematic representation of a combined logic circuit of INHIBIT and OR logic gates. | |
Molecular memory device
Molecular memory devices are used for data storage technologies that use molecular species as the data storage element and can be constructed by sequential logic circuits. One of the output signals acts as the input of the memory device and it is memorized as a “memory element”. So by using binary logic function, we developed a sequential logic circuit showing a “write–read–erase–read” property. For our system, we chose a strong emission output at 558 nm as the ON state (1) and a weak emission output as the OFF state (0). Now to construct this memory device, we chose two inputs, namely Fe3+ and CN−, for the SET and RESET processes, respectively. In this memory function, the system writes when it gets input A (Fe3+), i.e. a high emission value, and it memorizes the binary number 1. However, in the presence of input B (CN−), which is a reset input, it erases the data and then memorizes the binary number 0 (Fig. 13). The properties of the material allow for a much greater capacitance per unit area than with conventional DRAM (dynamic random-access memory), thus potentially leading to smaller and cheaper integrated circuits.
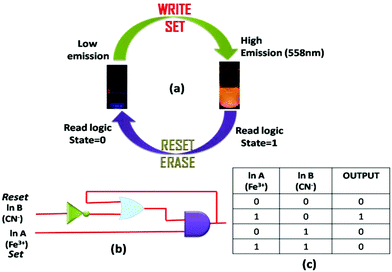 |
| Fig. 13 (a) Schematic demonstration of the reversible logic operation for the memory element with a “write–read–erase–read” kind of behaviour. (b) Sequential logic circuit showing a memory unit with two inputs (In A and In B) and one output, and (c) the corresponding truth table. | |
The most important thing is that this write–erase–write cycle could be repeated many times (Fig. 8) using the same concentration of the system without any significant change in emission intensity.
Cell-imaging studies
As L1 showed extensive selective complex formation with trivalent metal ions (namely Fe3+, Cr3+, Al3+ ions), it was further checked for its ion-sensing ability in living cells (Fig. 14). A cell viability assay using MTT66–68 was done to find out whether L1 had cytotoxic effects, with calculating the % cell viability on HepG2 cells (Fig. S16†). As found from the result, no significant decrease in formazan production occurred up to 40 μM concentration of L1, thus reflecting that a below 40 μM ligand concentration for L1 would be much more effective for the analysis of its complex formation with trivalent metal ions in vitro. More than 95% cell viability was observed for L1 at 10 μM, after which the viability of the HepG2 cells decreases slightly. Hence, further experiments were carried out with 10 μM for L1 for treatment.
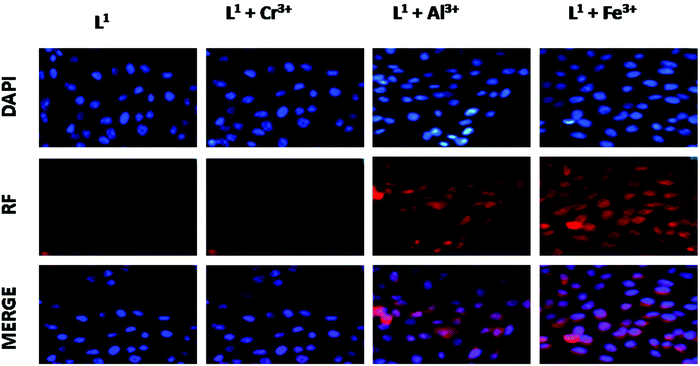 |
| Fig. 14 Phase contrast and fluorescence images of HepG2 cells captured (40×) after cells were incubated with L1 for 30 min at 37 °C and pre-incubated with Al3+, Cr3+ and Fe3+ for 30 min at 37 °C, followed by washing with 1× PBS and treatment with L1 for 30 min at 37 °C. The cytoplasmic complex formation was confirmed by nuclear stain DAPI. | |
Upon incubation with 10 μM of the ligand L1 for 1 h, it exhibited weak intracellular fluorescence on HepG2 cells due to the presence of intracellular Fe3+ (Fig. 14). However, a distinct red fluorescence was observed inside the cells when the HepG2 cells were incubated with 10 μM of the trivalent metal ions for 1 h at 37 °C, washed twice with PBS buffer and then reincubated with 10 μM of the ligand L1 for 30 min at 37 °C. At 10 μM concentration of the Fe3+ ions, the ligand L1 showed more intense fluorescence emission than Al3+ and Cr3+ ions.
Keeping the ligand L1 concentration constant (10 μM) and increasing the concentration of metal ions (from 10 μM, to 20 μM, 40 μM) showed a concentration-dependent increase in the intracellular red fluorescence, caused by the formation of the complex of L1 with either of the trivalent metal ions. Highly enhanced fluorescence was observed due to complex formation between the ligand L1 and the metal ions nearly at 40 μM of metal ion concentration. These results suggest that the ligand L1 with low cytotoxicity and biocompatibility has a high potential for in vitro application as an ion sensor of trivalent Fe3+, Cr3+, Al3+ ions as well as for live cell imaging for their detection in biological samples.
Fig. 15 represents some trivalent sensors reported so far and Table S1† displays some important parameters. A closer inspection of Table S1† reveals that our probe is superior to all the probes listed here in the sense that it provides higher excitation wavelength (502 nm). There is one report (probe 6) where CH3OH–H2O (6
:
4, v/v) was used, but the serious drawback of this system was that the excitation wavelength was in UV region (330 nm), which is not desirable for bioimaging applications.
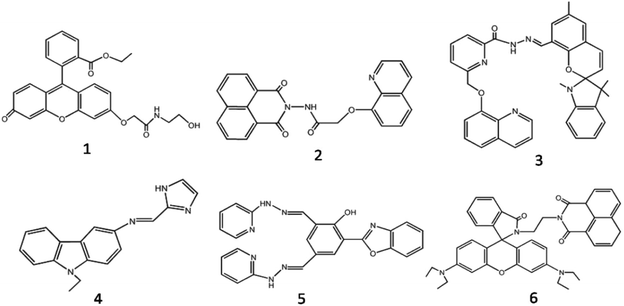 |
| Fig. 15 Some representative trivalent sensors. | |
Conclusion
In summary, we reported herein a new rhodamine-6G based fluorogenic probe, which showed a selective colorimetric as well as “turn-on” fluorescence response towards trivalent metal ions M3+ (M = Al, Fe and Cr) over mono- and divalent metal ions. A large enhancement of fluorescence intensity of L1 [Fe3+ (41-fold), Al3+ (31-fold) and Cr3+ (26-fold)] was observed upon the addition of 3.0 equivalent of these metal ions in H2O/CH3CN (4
:
1, v/v, pH 7.2), which clearly indicate the feasibility of the naked eye detection of these metal ions. Take-off values were evaluated from the fluorescence titration data at variable concentrations of metal ions and a fixed concentration of ligand and were found to be 9.4 × 103 M−1 (Fe3+); 8.7 × 103 M−1 (Cr3+) and 13.4 × 103 M−1 (Al3+). The higher values of quantum yields (0.489, 0.376, 0.310 for [L1–Fe3+], [L1–Al3+] and [L1–Cr3+], respectively) over the free ligand (0.012) indicate the higher stability of the complexes in the excited states. An excellent reversible fluorescence ON–OFF property of L1 was observed through fluorescence study with the sequential addition of M3+ and CN− ions at room temperature, which suggests the reusability of this chemosensor. The very low detection limit for Fe3+, Al3+ and Cr3+ were 1.28 μM, 1.34 μM and 2.28 μM, respectively, which could make it have a potential application in real water samples for trivalent ion detection. Advanced level molecular logic devices using different inputs (2 and 4 inputs) in advanced level logic gates and memory device were constructed. A suitably large increase in fluorescence intensity of L1 upon complexation with M3+ suggests the probe may be used for bioimaging applications in living cells.
Conflicts of interest
There are no conflicts to declare.
Acknowledgements
Financial supports from DST (Ref. SR/S1/IC-20/2012) New Delhi are gratefully acknowledged.
References
- M. Wang, F. Wang, Y. Wang, W. Zhang and X. Chen, Dyes Pigm., 2015, 120, 307 CrossRef CAS.
- X. Xing, H. Yang, M. Tao and W. Zhang, J. Hazard. Mater., 2015, 297, 207 CrossRef CAS PubMed.
- P. Wang, J. Wu, L. Liu, P. Zhou, Y. Ge, D. Liu, W. Liu and Y. Tang, Dalton Trans., 2015, 44, 18057 RSC.
- Z. Xu, K.-H. Baek, H. N. Kim, J. Cui, X. Qian, D. R. Spring, I. Shin and J. Yoon, J. Am. Chem. Soc., 2009, 132, 601 CrossRef PubMed.
- L. Tang, X. Dai, K. Zhong, X. Wen and D. Wu, J. Fluoresc., 2014, 24, 1487 CrossRef CAS PubMed.
- M. Idrees, M. Silva, A. F. Silva, L. M. Zimmermann, J. Bruch, C. O. Mendonça, G. I. Almerindo, R. A. Nome, T. D. Z. Atvars, H. D. Fiedler and F. Nome, J. Phys. Chem. C, 2012, 116, 3517 CrossRef CAS.
- S. Maiti, Z. Aydin, Y. Zhang and M. Guo, Dalton Trans., 2015, 44, 8942 RSC.
- S. B. Maity and P. K. Bharadwaj, Inorg. Chem., 2013, 52, 1161 CrossRef CAS PubMed.
- S. Sarma, P. K. Bhattacharyya and D. K. Das, J. Fluoresc., 2015, 25, 1537 CrossRef CAS PubMed.
- Mercury Study Report to Congress, United States Environmental Protection Agency, Volume V: Health Effects of Mercury and Mercury Compounds, EPA-452/R-97-007 December (1997).
- Y. Upadhyay, S. Bothra, R. Kumar and S. K. Sahoo, ChemistrySelect, 2018, 3, 6892 CrossRef CAS.
- T. Anand, S. K. A. Kumar and S. K. Sahoo, Spectrochim. Acta, Part A, 2018, 204, 105 CrossRef CAS PubMed.
- Y. Upadhyay, T. Anand, L. T. Babu, P. Paira, S. K. A. Kumar, R. Kumar and S. K. Sahoo, J. Photochem. Photobiol., A, 2018, 361, 34 CrossRef CAS.
- S. K. Sahoo, D. Sharma, R. K. Bera, G. Crisponi and J. F. Callan, Chem. Soc. Rev., 2012, 41, 7195 RSC.
- S. Samanta, S. Goswami, A. Ramesh and G. Das, Sens. Actuators, B, 2014, 194, 120 CrossRef CAS.
- S. Dey, S. Sarkar, D. Maity and P. Roy, Sens. Actuators, B, 2017, 246, 518 CrossRef CAS.
- X. Chen, X. Y. Shen, E. Guan, Y. Liu, A. Qin, J. Z. Sun and B. Z. Tang, Chem. Commun., 2013, 49, 1503 RSC.
- N. R. Chereddy, P. Nagaraju, M. V. NiladriRaju, V. R. Krishnaswamy, P. S. Korrapati, P. R. Bangal and V. J. Rao, Biosens. Bioelectron., 2015, 68, 749 CrossRef CAS PubMed.
- A. Barba-Bon, A. M. Costero, S. Gil, M. Parra, J. Soto, R. M. Máñez and F. Sancenón, Chem. Commun., 2012, 48, 3000 RSC.
- T. Simona, M. Shellaiah, V. Srinivasadesikan, C.-C. Lina, F.-H. Koa, K. W. Sun and M.-C. Lin, Sens. Actuators, B, 2016, 231, 18 CrossRef.
- A. Singh, R. Singh, M. Shellaiah, E.
C. Prakash, H.-C. Chang, P. Raghunath, M.-C. Lin and H.-C. Lin, Sens. Actuators, B, 2015, 207, 338 CrossRef CAS.
- P. N. Borase, P. B. Thale, S. K. Sahoo and G. S. Shankarling, Sens. Actuators, B, 2015, 215, 451 CrossRef CAS.
- M. Shellaiah, T. Simon, V. Srinivasadesikan, C.-M. Lin, K. W. Sun, F.-H. Ko, M.-C. Lin and H.-C. Lin, J. Mater. Chem. C, 2016, 4, 2056 RSC.
- S. Tao, Y. Wei, C. Wang, Z. Wang, P. Fan, D. Shi, B. Ding and J. Qiu, RSC Adv., 2014, 4, 46955 RSC.
- B. L. Su, N. Moniotte, N. Nivarlet, L. H. Chen, Z. Y. Fu, J. Desmet and J. Li, J. Colloid Interface Sci., 2011, 358, 136 CrossRef CAS PubMed.
- T. Hirayama, K. Okuda and H. Nagasawa, Chem. Sci., 2013, 4, 1250 RSC.
- J. Xu, Z. Jia, M. D. Knutson and C. Leeuwenburgh, Int. J. Mol. Sci., 2012, 13, 2368 CrossRef CAS PubMed.
- D. J. Bonda, H. Lee, J. A. Blair, X. Zhu, G. Perry and M. A. Smith, Metallomics, 2011, 3, 267 RSC.
- S. Toyokuni, Cancer Sci., 2009, 100, 9 CrossRef CAS PubMed.
- K. V. Kowdley, Gastroenterology, 2004, 127, S79–S86 CrossRef CAS.
- F. Molina-Holgado, R. Hider, A. Gaeta, R. Williams and P. Francis, BioMetals, 2007, 20, 639 CrossRef CAS PubMed.
- Z. Zhou, M. Yu, H. Yang, K. Huang, F. Li, T. Yi and C. Huang, Chem. Commun., 2008, 29, 3387 RSC.
- J. B. Vincent, Nutr. Rev., 2000, 58, 67 CrossRef CAS PubMed.
- M. Zhang, Z. Chen, Q. Chen, H. Zou, J. Lou and J. He, Mutat. Res., Genet. Toxicol. Environ. Mutagen., 2008, 654, 45 CrossRef CAS PubMed.
- B. Vincent, Nutr. Rev., 2000, 58, 67 CrossRef PubMed.
- H. X. Jiang, L. S. Chen, J. G. Zheng, S. Han, N. Tang and B. R. Smith, Tree Physiol., 2008, 28, 1863 CrossRef CAS PubMed.
- G. Berthon, Coord. Chem. Rev., 1996, 149, 241 CrossRef CAS.
-
S. M. Candura, L. Manzo and L. G. Costa, Role of occupational neurotoxicants in psychiatric and neurodegenerative disorders, in Occupational Neurotoxicology, ed. L. G. Costa and L. Manzo, CRC Press, Boca Raton, 1998, p. 131 Search PubMed.
- R. J. P. Williams, Coord. Chem. Rev., 1992, 149, 1 CrossRef.
- G. R. Rout, S. S. Roy and P. Das, Agronomie, 2001, 21, 3 CrossRef.
-
(a) J. Barcelo and C. Poschenrieder, Environ. Exp. Bot., 2002, 48, 75 CrossRef CAS;
(b) B. Valeur and I. Leray, Coord. Chem. Rev., 2000, 205, 3 CrossRef CAS;
(c) Z. Krejpcio and R. W. P. Wojciak, Int. J. Environ. Stud., 2002, 11, 251 CAS.
- M. R. Wills, C. D. Hewitt, B. C. Sturgill, J. Savory and M. M. Herman, Ann. Clin. Lab. Sci., 1993, 23, 1 CrossRef CAS PubMed.
- G. Sahin, I. Varol and A. Temizer, Biol.
Trace Elem. Res., 1994, 41, 129 CrossRef CAS PubMed.
- A. C. Alfrey, NeuroToxicology, 1980, 1, 43 CAS.
-
(a) G. D. Fasman, Coord. Chem. Rev., 1996, 149, 125 CrossRef CAS;
(b) P. Nayak, Environ. Res., 2002, 89, 111 CrossRef;
(c) C. S. Cronan, W. J. Walker and P. R. Bloom, Nature, 1986, 324, 140 CrossRef CAS;
(d) G. Berthon, Coord. Chem. Rev., 2002, 228, 319 CrossRef CAS;
(e) D. R. Burwen, S. M. Olsen, L. A. Bland, M. J. Arduino, M. H. Reid and W. R. Jarvis, Kidney Int., 1995, 48, 469 CrossRef CAS PubMed.
- S. G. Lambert, J. A. M. Taylor, K. L. Wegener, S. L. Woodhouse, S. F. Lincoln and A. D. Ward, New J. Chem., 2000, 24, 541 RSC.
- D. Maity and T. Govindaraju, Chem. Commun., 2010, 46, 4499 RSC.
- Y. S. Jang, B. Yoon and J. M. Kim, Macromol. Res., 2011, 19, 97 CrossRef CAS.
- A. M. Zayed and N. Terrey, Plant Soil, 2003, 249, 139 CrossRef CAS.
-
W. Fresenius, K. E. Quentin and W. Schneider, Water Analysis, Springer, Berlin, 1988 Search PubMed.
- X.-d. Wang and O. S. Wolfbeis, Chem. Soc. Rev., 2014, 43, 3666 RSC.
- B. Gibson, S. Carter, A. S. Fisher, S. Lancaster, J. Marshall and I. Whiteside, J. Anal. At. Spectrom., 2014, 29, 1969 RSC.
- J. Wang, Y. Li, N. G. Patel, G. Zhang, D. Zhouand and Y. Pang, Chem. Commun., 2014, 50, 12258 RSC.
- S. Goswami, K. Aich, S. Das, A. K. Das, D. Sarkar, S. Panja, T. K. Mondal and S. Mukhopadhyay, Chem. Commun., 2013, 49, 10739 RSC.
- M. Venkateswarulu, T. Mukherjee, S. Mukherjee and R. R. Koner, Dalton Trans., 2014, 43, 5269 RSC.
- J. Miao, L. Wang, W. Dou, X. L. Tang, Y. Yan and W. S. Liu, Org. Lett., 2007, 9, 4567 CrossRef PubMed.
- E. Delhaize and P. R. Ryan, Plant Physiol., 1995, 107, 315 CrossRef CAS PubMed.
- S. Goswami, A. K. Das, A. K. Maity, A. Manna, K. Aich, S. Maity, P. Saha and T. K. Mandal, Dalton Trans., 2014, 43, 231 RSC.
- S. Fakih, M. Podinovskaia, X. Kong, H. L. Collins, V. E. Schoible and R. C. J. Hider, Med. Chem., 2008, 51, 4539 CrossRef CAS PubMed.
- S. Kim, J. Y. Noh, K. Y. Kim, J. H. Kim, H. K. Kang, S. W. Nam, S. H. Kim, S. Park, C. Kim and J. H. Kim, Inorg. Chem., 2012, 51, 3597 CrossRef CAS PubMed.
- S. Paul, A. Manna and S. Goswami, Dalton Trans., 2015, 44, 11805 RSC.
- R. Alam, R. Bhowmick, A. S. M. Islam, A. Katarkar, K. Chaudhuri and M. Ali, New J. Chem., 2017, 41, 8359 RSC.
- R. Alam, T. Mistri, R. Bhowmick, A. Katarkar, K. Chaudhuri and M. Ali, RSC Adv., 2016, 6, 1268 RSC.
- H. A. Molla, R. Bhowmick, A. Katarkar, K. Chaudhuri, S. Gangopadhyay and M. Ali, Anal. Methods, 2015, 7, 5149 RSC.
- Y. Liu, K. Ai, X. Cheng, L. Huo and L. Lu, Adv. Funct. Mater., 2010, 20, 951 CrossRef CAS.
- R. Alam, T. Mistri, R. Bhowmick, A. Katarkar, K. Chaudhuri and M. Ali, RSC Adv., 2015, 5, 53940 RSC.
- R. Alam, T. Mistri, A. Katarkar, K. Chaudhuri, S. K. Mandal, A. R. Khuda-Bukhsh, K. K. Das and M. Ali, Analyst, 2014, 139, 4022 RSC.
- R. Alam, T. Mistri, P. Mondal, D. Das, S. K. Mandal, A. R. Khuda-Bukhsh and M. Ali, Dalton Trans., 2014, 43, 2566 RSC.
Footnote |
† Electronic supplementary information (ESI) available. CCDC 1836133. For ESI and crystallographic data in CIF or other electronic format see DOI: 10.1039/c8pp00381e |
|
This journal is © The Royal Society of Chemistry and Owner Societies 2019 |